DOI:
10.1039/C5RA01081K
(Paper)
RSC Adv., 2015,
5, 28814-28821
Efficient sodium bisulfite-catalyzed synthesis of benzothiazoles and their potential as ureases inhibitors†
Received
19th January 2015
, Accepted 17th March 2015
First published on 17th March 2015
Abstract
In this work we report the successful use of sodium bisulfite as catalyst for the synthesis of 19 benzothiazoles (BZTs) under microwave irradiation with yields from 80% to 100%. BZT-15 was the most active jack bean urease inhibitor exhibiting a mechanism of action typically of a mixed inhibitor. Its affinity to bind urease active site is 3-fold higher than that to bind allosteric site(s). The BZTs 2, 8–10, 15 and 16 are described for the first time as soil ureases inhibitors. Overall, these results show the potential of BZTs to be used either as lead compound for the design of drugs for treating urease-induced diseases or as additive in urea-based fertilizers to improve N input in soils used for crop production.
Introduction
Benzothiazoles (BZTs) comprise a class of heterocyclic compounds that bear a benzene ring fused to a thiazole ring at positions 4 and 5. The BZT core is a privileged scaffold as attested by its use for the development of products of agricultural, pharmaceutical and technological interest.1–5 For instance, the water soluble L-lysyl amide (phortress) prodrug of BZT-5F 203 (Fig. 1) is currently on clinical trial in the UK due to the selective antitumor activity of BZT-5F 203 against breast, ovarian and renal human carcinomas.6–8 Riluzole (or Rilutek®; Fig. 1) is one of the few drugs approved for the treatment of the neurodegenerative disease amyotrophic lateral sclerosis.9 BZT-I and BZT-II (Fig. 1) exhibit excellent in vitro antifungal activities. Additionally, in vivo assays disclosed that BZT-I is more effective than the commercial antifungal kresoxim-methyl against Sphaerotheca fuliginea and Pseudoperoniospora cubensis.10 A potent inhibitory effect on the ureolytic activity of urease was reported for 2-aminobenzothiazole (BZT-III), exhibiting an IC50 value of 28.88 μg mL−1 (79.7 μM) under the tested experimental conditions.11
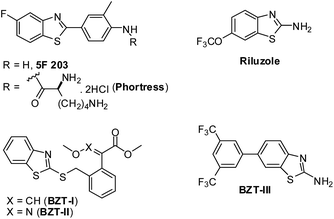 |
| Fig. 1 Benzothiazoles (BZTs) of pharmacological interest. | |
Several methods were developed for preparing benzofused heterocycles.2,12 Among them, two main methodologies have been widely explored for the synthesis of BZTs.12,13 The first one is based on the direct reaction of 2-aminothiophenol with aldehydes, ketones, halides or carboxylic acids and derivatives; this is the most used approach for the preparation of BZTs since it develops with no need of sequential multistep reactions to prepare starting materials. The second one involves the intramolecular cyclization of o-substituted-thiobenzamides. The direct approach used to synthesize BZTs presents, however, some bottlenecks that include (i) the use of toxic (e.g. H2O2/HCl,14 H2O2/Co(NO3)2,15 H2O2/CAN16) and expensive reagents or catalysts (e.g. RuCl3,17 Ru(PPh3)3(CO)H2/Xantphos,18 YCl3 (ref. 19)), (ii) the requirement of long reaction time,17,19 (iii) the occurrence of side reactions, (iv) the requirement of laborious workup and/or purification procedures,14,17 (v) formation of the desired products in low yields17,18 and (vi) the requirement of catalyst synthesis.20,21 Therefore, the search for new cost-effective catalysts, in particular, is certainly needed to make the synthesis of BZTs more feasible.
Sodium bisulfite (NaHSO3) has emerged as a cheap catalyst for the formation of C–C (ref. 22) and C–O (ref. 23 and 24) bonds. Its catalytic efficiency for the synthesis of 2,5-substituted 1,3,4-oxadizoles and substituted pyrano[2,3-c]pyrazoles is also documented.25,26 Additionally, NaHSO3 was shown to act as a cocatalyst in phosphorus-free Wittig reactions.27 Indeed, the use of such inorganic reducing reagent considerably simplifies the product purification since the reaction is carried out in the absence of triphenyl phosphite. Although NaHSO3 was determined to be an efficient catalyst for the synthesis of benzimidazoles under conventional heating,28 its use as a catalyst for the synthesis of benzothiazoles (BZTs) was not reported up-to-date.
As for the biological activities, the potential of BZTs as ureases inhibitors is still poorly investigated. Urease (EC 3.5.1.5) is a key enzyme for the global nitrogen (N) cycle, occurring in plants,29 fungi30 and bacteria.31 This type of hydrolase speeds up the urea hydrolysis rate to ammonia (NH3) and carbon dioxide by one hundred trillion folds.32 The persistence of urease activity in human and animal cells is known to be the cause of some diseases and pathogen infections. For example, urine and/or gastrointestinal infections by ureolytic bacteria can cause health complications in humans and animals such as kidney stone formation, pyelonephritis and hepatic encephalopathy.33,34 Indeed, major public health issues are related to the Gram-negative bacteria Helicobacter pylori, known to be able to survive under acidic conditions as that of the stomach (pH 2.0). As a result, uncontrolled proliferation of H. pylori can induce duodenal and gastric ulcers, gastric adenocarcinoma or gastric lymphoma.7,35 Then, the use of urease inhibitors may be effective therapies to overcome health issues associated with urease activity.36–40
In addition to health problems, ureases (particularly soil ureases) can negatively affect food production by stimulating N losses to atmosphere from urea-based fertilizers when applied to soil surface. Urea is some of the most used N fertilizers worldwide. Its advantages over other nitrogen fertilizers include high N content (46%), low price, water solubility and easy management.41 A common practice of soil fertilization is the application of urea to soil surface that, in turn, can lead to over 50% of N losses to atmosphere due to the activity of soil ureases.41,42 Thus, the use of urease inhibitors as additive to urea-based fertilizers has received considerable attention as a strategy to overcome N losses in field.41
Taking all of these into account, we describe herein the use of sodium bisulfite (NaHSO3) as an efficient catalyst for the preparation of 19 BZTs under microwave irradiation (MWI). The potential of BZTs synthesized as urease inhibitors of clinical and agricultural interested was also investigated in in vitro and in soil assays.
Results and discussion
Chemistry: sodium bisulfite-catalyzed synthesis of benzothiazoles
For the preparation of benzothiazoles (BTZs), we initially carried out several reactions of o-aminothiophenol with benzaldehyde in the presence of sodium bisulfite (NaHSO3) as catalyst to find the best reaction conditions to synthesize BZT-1. The N,N-dimethylacetamide (DMA) was used as solvent in reactions carried out for 15 or 30 min under microwave irradiation (MWI) at different temperatures (80, 100 or 120 °C). The increase of reaction time, accompanied by an increment in temperature, furnished BZT-1 in much higher yields (Table 1). The maximum reaction yield of 90% was registered upon 30 min-reaction regardless of the use of temperatures higher than 120 °C. It is noteworthy that under the optimal conditions, reactions devoid of NaHSO3 furnished BZT-1 in yields lower than 25%. Thus, we demonstrate for the first time that NaHSO3, a cheap chemical, is a great catalyst for the synthesis of BZTs as it is for providing unsymmetrical ethers, 2,4,5-triphenyl-1H-imidazoles, 2,5-disubstituted 1,3,4-oxadiazoles and substituted pyrano[2,3-c]pyrazoles.22–26 Additionally, the use of MWI was very effective to speed up BZT-1 formation since it took 12-fold more time (6 h) to obtain BZT-1 in comparable yields (85%) from NaHSO3-catalyzed reactions under conventional heating (120 °C). In fact, MWI has been extensively employed for reducing reaction time and also improving product yields.43–47
Table 1 Effect of time of microwave irradiation (MWI) and temperature on the formation of BZT-1a

|
Temperature |
Time (min) |
Yield (%) |
Reagents and solvent: o-aminothiophenol (5.0 mmol), benzaldehyde (5.5 mmol), NaHSO3 (10.0 mmol) and N,N-dimethylacetamide (DMA; 2.0 mL per 5.5 mmol of aldehyde). |
80 °C |
15 |
6 |
30 |
45 |
100 °C |
15 |
27 |
30 |
84 |
120 °C |
15 |
42 |
30 |
90 |
Based on these results, 30 min of MWI and temperature of 120 °C were selected as the reaction parameters for further exploring the use of NaHSO3 as catalyst for obtaining other 18 BZTs. Thus, different (hetero)aromatic aldehydes and cyclohexanecarboxaldehyde were placed to react with o-aminothiophenol under MWI in the presence of NaHSO3 to obtain a variety of BZTs (Table 2). The use of NaHSO3 as catalyst furnished BZTs bearing electron-donating or electron-withdrawing substituents in excellent yields (>80%; Table 2). Previous report described that NaHSO3 is an efficient catalyst for the synthesis of benzimidazoles under conventional heating, with yields in the range from 13% to 90%.28 By using a domestic microwave oven and NaHSO3, benzimidazoles were obtained in yields from 67% to 99%.48 However, we failed to obtain BZTs in yields higher than 42% under the optimized conditions described for benzimidazoles48 when using CEM – Microwave-Enhanced Life reactor for organic synthesis instead of a domestic microwave oven. Indeed, results of organic synthesis based on the use of domestic microwave ovens are difficult to be reproducible because such equipment does not allow the reliable control of temperature, irradiation power and the homogeneity of magnetic field.49,50 Under our experimental conditions, NaHSO3-catalyzed reactions yielded BZT-9 and BZT-11 in 100% while the use of the catalyst yttrium chloride (YCl3) furnished these same BZTs in yields lower than 94%.51 As for the synthesis of BZT-4, BZT-6 and BZT-11, NaHSO3 furnished higher yields (90–100%) than the copper–DiAmSar complex anchored onto mesoporous SBA-15 silica whose yields ranged from 85–90%.21 The yield of BZT-14 in NaHSO3-catalyzed reactions (Table 2) was 61% and 28% higher than that of bakers' yeast- and Fe/montmorillonite K-10-catalyzed reactions, respectively.20,52 Also, the efficiency of NaHSO3 for obtaining BZT-4 and BZT-8 (Table 2) was, respectively, ca. 25% and 15% higher than that of bakers' yeast.20 The much higher performance of the catalyst NaHSO3 is also evidenced for the synthesis of BZT-2, BZT-4, BZT-6, BZT-14, BZT-16 and BZT-18 (Table 2) when compared to that of the catalyst sodium dithionite.53 Overall, our methodology for the synthesis of BZTs was proven to be efficient and interesting as it uses (i) mild conditions and NaHSO3, a cheap catalyst, (ii) short reaction time (30 min) due to the employment of MWI and (iii) an easy procedure (precipitation) for purifying the BZTs.
Table 2 Use of different aldehydes in sodium bisulfite-catalyzed reaction for the synthesis of BZTs under optimized conditionsa
Biology: ureases inhibitory activity and kinetic studies
The effect of 19 BZTs synthesized on ureases activity was first addressed toward jack bean type III urease in reactions containing 10 mM urea and compounds-test at 1.6 mM. The compounds BZT-1, BZT-2, BZT-9 and BZT-15-18 were shown to inhibit the jack bean urease (Fig. 2). The BZT-15, the most active synthesized compound, was determined to be as active as hydroxyurea (HU; 62% inhibition), a known urease inhibitor, while BZT-16 was found to be as effective as thiourea (TU; 26% inhibition), another known urease inhibitor (Fig. 2). The inhibitory activity of BZT-17 was slightly lower than that of TU. The urease inhibition exhibited by the other active BZTs was less expressive being lower than 10% (Fig. 2). Under our experimental conditions, compounds BZT-6 and BZT-10–BZT-12 were inactive against jack bean urease. These results are in agreement with those reported elsewhere.54 The results described here for BZT-9, BZT-15 and BZT-16 against jack bean urease contrast those previously reported, in which such BZTs were determined to be almost inactive.54 Although the enzymatic reaction conditions were not detailed in the previous report, one can assume they were likely distinct from those described herein. Other BZTs such as 2-amino-6-aryl-benzothiazoles were also active against jack bean urease, exhibiting IC50 values in the range from 79.7 to 123.5 μM (concentrations were originally reported in μg mL−1).11
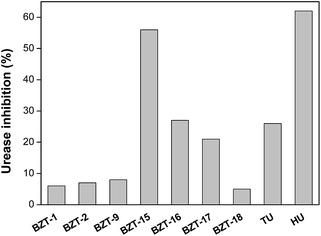 |
| Fig. 2 Inhibitory effect of benzothiazoles (BZTs) synthesized on jack bean urease. BZTs (1.6 mM) were tested in reactions containing 10 mM urea. Thiourea (TU) and hydroxyurea (HU) were used as reference of urease inhibitors. | |
Thus, we selected the most active compound synthesized (BZT-15) to investigate the mechanism of action by which this substance inhibits jack bean urease. Kinetic studies experiments carried out with different concentrations of inhibitor showed that 0.5 mM BZT-15 caused an increment of 30% in urea KM while an increase of 47% in urea KM was observed in reactions containing 0.7 mM BZT-15 (Table 3). On the other hand, the urease Vmax decreased by 14% and 26% in reactions containing BZT-15 at 0.5 mM and 0.7 mM, respectively (Table 3). The concentration-dependent effect of BZT-15 on kinetic parameters of urease, along with the profile of the Lineweaver–Burk plot (Fig. 3), indicates that this molecule is a typical mixed inhibitor. This type of inhibitor is known to be able to bind both the free enzyme and the enzyme–substrate complex being, therefore, related to two inhibitor dissociation constants (Ki and K′i).55 The dissociation constant for the urease–BZT-15 complex (Ki) was determined to be 1.02 ± 0.04 mM and the dissociation constant for the urease–urea–BZT-15 complex (K′i) was 3.17 ± 0.69 mM. These values indicate that BZT-15 affinity to urease active site is 3-fold higher than that for urease–urea complex. To the best of our knowledge this is the first study about the effect of a BZT on the kinetic parameters of urease.
Table 3 Effect of BZT-15 on kinetic parameters of jack bean type III ureasea
BZT-15 (mM) |
KM or KM (app) (mM) |
Vmax or Vmax (app) (μmol NH4+ min−1 mg−1 prot) |
KM (app) and Vmax (app) are respectively the apparent KM and apparent Vmax, i.e., the values of KM and Vmax measured for urea and urease in reactions containing BZT-15. |
0.0 |
3.0 |
8.8 |
0.5 |
3.9 |
7.6 |
0.7 |
4.4 |
6.5 |
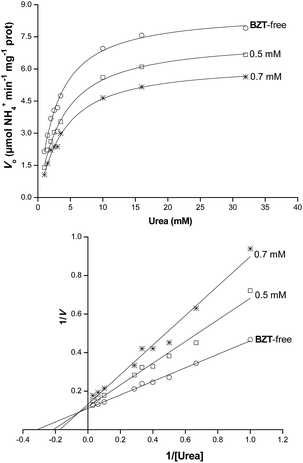 |
| Fig. 3 Michaelis–Menten hyperbola (upper graph) and Lineweaver–Burk plot (lower graph) for jack bean urease as a function of increased concentrations of BZT-15. Reactions containing urea (1–32 mM) were carried out in the absence (BZT-free) or presence of BZT-15 at concentrations indicated. | |
Assays using amended soil from Brazilian Cerrado were also performed to investigate the potential of BZTs synthesized as urease inhibitors of agricultural interest. Interestingly, 14 out of 19 BZTs tested inhibited soil ureases at different extents (Fig. 4). Results allowed us to categorize the BZTs in four groups: (i) group 1 constituted of inhibitors more active than NBPT (BZT-10); (ii) group 2 formed by BZTs as active as NBPT (BZT-2, BZT-8, BZT-9, BZT-15 and BZT-16); (iii) group 3 formed by BZTs that inhibited soil ureases by lower than 13% and (iv) group 4 that includes non-active BZTs (BZT-6, BZT-11, BZT-13, BZT-17 and BZT-19). Surprisingly, BZT-10, found to be inactive in in vitro assays (Fig. 2), was determined to be the most potent soil ureases inhibitor (Fig. 4). In the same way, BZT-17 exhibited in vitro urease inhibition comparable to that of the reference TU, but fail to inhibit soil ureases (Fig. 2 and 4). These can be explained by differences with respect both procedures. The in vitro assay with purified urease comprises a less complex system when compared to soil, in which both physicochemical features and the variety of microorganisms present in it may affect at different extents the performance of xenobiotics, as is the case of synthetic urease inhibitors.56 As a result, interaction of a urease inhibitor with soil matrix may either improve or compromise the efficacy of the inhibitor.
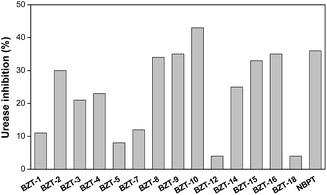 |
| Fig. 4 Activity of benzothiazoles (BZTs) synthesized on soil ureases. Soil (0.5 g) was incubated with 72 mM urea in the presence of BZTs (1.6 mM) or NBPT (1.6 mM; reference of soil ureases inhibitor). | |
Conclusions
This work demonstrates that the use of sodium bisulfite (NaHSO3) as a catalyst under MWI furnish benzothiazoles (BZTs) in excellent yields. It is demonstrated for the first time that BZT-15 is a mixed-type urease inhibitor that might be used as a lead compound for the design of drugs to treat urease-associated diseases. We also disclose the potential of six BZTs (2, 8, 9, 10, 15 and 16) for use as additive in urea-based fertilizers to improve N availability in soil for crop production.
Experimental section
All chemicals (analytical grade) were obtained from commercial suppliers and used without further purification. Jack bean type III urease was purchased from Sigma-Aldrich. Uncorrected melting points were determined in Gehaka PF 1500 apparatus. Reactions under microwave irradiation (MWI) were carried out in a CEM – Microwave-Enhanced Life reactor. The 1H nuclear magnetic resonance (NMR) and 13C NMR spectra were recorded on a Bruker Avance DRX/400 or DPX/200. Chemical shift values (δ) were given in parts per million (ppm). Infrared (IR) spectra were recorded on a Spectrometer One Perkin Elmer. Mass spectra were determined on a Shimadzu LCMS-IT-TOF.
General procedure for the synthesis of benzothiazoles (BZT-1–BZT-19)
A mixture of NaHSO3 (10.0 mmol) and dimethylacetamide (DMA) (2 mL) was stirred for 10–20 min. Then, o-aminothiophenol (5.0 mmol) and different sorts of aldehyde (5.5 mmol) were individually added to the mixture. Each reaction was subjected to microwave irradiation (MWI) for 30 min at 120 °C in a CEM – Microwave-Enhanced Life reactor, unless otherwise stated. The reaction mixtures were cooled down to room temperature and poured into water to precipitate the product in high purity grade.
2-Phenylbenzothiazole (BZT-1). Yield (90%). White solid. M. p. 109.1–110.4 °C (Lit:57 112.0–113.0 °C). 1H NMR (DMSO-d6, 200 MHz): δ 7.40–7.56 (m, 5H), 8.05–8.11 (m, 4H). 13C NMR (DMSO-d6, 50 MHz): δ 121.1, 122.8, 125.4, 126.5, 127.1, 129.2, 131.2, 132.7, 134.4, 153.5, 167.1. IR (KBr): 3062, 1510, 1478, 1432, 1312, 1224, 962, 766, 730, 686 cm−1. LCMS-IT-TOF calculated for C13H10NS [M + H]+: 212.0528, found: 212.0477.
2-(4-Nitrophenyl)benzothiazole (BZT-2). Yield (80%). Yellow solid. M. p. 229.5–230.9 °C (Lit:58 230.0–232.0 °C). 1H NMR (CDCl3, 200 MHz): δ 7.44–7.60 (m, 2H), 7.97 (d, 1H, J = 7.8 Hz), 8.14 (d, 1H, J = 8.0 Hz), 8.27 (d, 2H, J = 8.9 Hz), 8.36 (d, 2H, J = 8.9 Hz). 13C NMR (CDCl3, 50 MHz): δ 121.8, 124.0, 124.3, 126.2, 126.9, 128.3, 135.6, 139.2, 149.1, 154.2, 164.8. IR (KBr): 3088, 3062, 2990, 2936, 2844, 1606, 1596, 1522, 1342, 1312, 1108, 970, 852, 766 cm−1. LCMS-IT-TOF calculated for C13H9N2O2S [M + H]+: 257.0379, found: 257.0325.
2-(4-Cyanophenyl)benzothiazole (BZT-3). Yield (85%). Yellow solid. M. p. 167.8–168.9 °C (Lit:58 169.0–172.0 °C). 1H NMR (DMSO-d6, 200 MHz): δ 7.51 (sl, 2H), 7.92–8.14 (m, 6H). 13C NMR (DMSO-d6, 50 MHz): δ 113.2, 118.2, 122.4, 123.2, 126.1, 126.8, 127.6, 133.1, 134.8, 136.5, 153.3, 165.1. IR (KBr): 3062, 2992, 2226, 1606, 1558, 1480, 1406, 1314, 1250, 968, 838, 764 cm−1. LCMS-IT-TOF calculated for C14H9N2S [M + H]+: 237.0481, found: 237.0407.
2-(4-N,N-Dimethylaminophenyl)benzothiazole (BZT-4). Yield (90%). Yellow solid. M. p. 168.2–169.9 °C (Lit:57 173.0–174.0 °C). 1H NMR (CDCl3, 200 MHz): δ 3.03 (s, 6H), 6.73 (d, 2H, J = 8.8 Hz), 7.29 (t, 1H, J = 8.0 Hz), 7.43 (t, 1H, J = 7.6 Hz), 7.83 (d, 1H, J = 8.0 Hz), 7.92 (m, 3H). 13C NMR (CDCl3, 50 MHz): δ 40.1, 111.8, 121.3, 121.6, 122.3, 124.1, 125.9, 128.9, 134.7, 152.3, 154.5, 168.7. IR (KBr): 3052, 3028, 2900, 2814, 1610, 1554, 1484, 1430, 1368, 1314, 1226, 1188, 818, 752, 720 cm−1. LCMS-IT-TOF calculated for C15H15N2S [M + H]+: 255.0950, found: 255.0890.
2-(4-Methylthiophenyl)benzothiazole (BZT-5). Yield (98%). Yellow solid. M. p. 146.3–147.6 °C (Lit:59 142.0–144.0 °C). 1H NMR (CDCl3, 200 MHz): δ 2.54 (s, 3H), 7.30–7.53 (m, 4H), 7.89 (d, 1H, J = 7.4 Hz), 7.98–8.07 (m, 3H). 13C NMR (CDCl3, 50 MHz): δ 15.3, 121.5, 123.1, 125.1, 126.2, 126.3, 127.8, 130.4, 135.0, 142.8, 154.3, 167.5. IR (KBr): 3052, 2986, 2918, 1654, 1596, 1474, 1434, 1310, 1230, 1094, 960, 814, 758 cm−1. LCMS-IT-TOF calculated for C14H12N2S [M + H]+: 258.0406, found: 258.0397.
2-(4-Methoxyphenyl)benzothiazole (BZT-6). Yield (100%). Beige solid. M. p. 117.7–119.3 °C (Lit:57 119.0–120.0 °C). 1H NMR (DMSO-d6, 200 MHz): δ 3.83 (s, 3H), 7.08 (d, 2H, J = 8.4 Hz), 7.37–7.54 (m, 2H), 7.99–8.08 (m, 4H). 13C NMR (DMSO-d6, 50 MHz): δ 55.3, 114.6, 122.0, 122.4, 124.9, 125.4, 126.4, 128.7, 134.1, 153.6, 161.7, 166.9. IR (KBr): 3062, 2928, 2852, 1594, 1560, 1516, 1438, 1314, 1246, 992, 758, 730 cm−1. LCMS-IT-TOF calculated for C14H12NOS [M + H]+: 242.0634, found: 242.0688.
2-(3-Methoxyphenyl)benzothiazole (BZT-7). Yield (100%). Beige solid. M. p. 79.2–81.3 °C (Lit:58 80.0–81.0 °C). 1H NMR (DMSO-d6, 200 MHz): δ 3.38 (s, 3H), 7.10–7.16 (m, 1H), 7.41–7.54 (m, 3H), 7.57–7.64 (m, 2H), 8.09 (t, 2H, J = 7.4 Hz). 13C NMR (DMSO-d6, 50 MHz): δ 55.2, 111.5, 117.2, 119.7, 122.2, 122.8, 125.4, 126.5, 130.4, 134.1, 134.4, 153.4, 159.6, 167.0. IR (KBr): 3078, 3060, 2962, 2934, 2834, 1606, 1582, 1512, 1430, 1314, 1290, 996, 900, 762, 728 cm−1. LCMS-IT-TOF calculated for C14H12NOS [M + H]+: 242.0634, found: 242.0703.
2-(4-Fluorophenyl)benzothiazole (BZT-8). Yield (85%). Yellow solid. M. p. 93.8–95.9 °C (Lit:60 97.2–98.5 °C). 1H NMR (DMSO-d6, 200 MHz): δ 7.33–7.57 (m, 4H), 8.02–8.15 (m, 4H). 13C NMR (DMSO-d6, 50 MHz): δ 116.1, 116.5, 122.2, 122.7, 125.4, 126.6, 129.4, 129.5, 134.5, 153.4, 161.3, 166.0, 166.3. IR (KBr): 3052, 3028, 2990, 1604, 1520, 1480, 1434, 1228, 968, 836, 756, 728 cm−1. LCMS-IT-TOF calculated for C13H9FNS [M + H]+: 230.0434, found: 230.0367.
2-(4-Hydroxyphenyl)benzothiazole (BZT-9). Yield (100%). Yellow solid. M. p. 224.1–225.9 °C (Lit:61 227.0 °C). 1H NMR (DMSO-d6, 200 MHz): δ 6.95 (sl, 1H), 7.37–7.50 (m, 2H), 7.94–8.04 (m, 4H). 13C NMR (DMSO-d6, 50 MHz): δ 116.1, 122.0, 122.2, 123.9, 124.8, 126.3, 129.0, 134.1, 153.7, 160.7, 167.4. IR (KBr): 3054, 2994, 2796, 2590, 1606, 1586, 1432, 1284, 1224, 976, 826, 756, 724 cm−1. LCMS-IT-TOF calculated for C13H10NOS [M + H]+: 228.0478, found: 228.0389.
2-(3-Hydroxyphenyl)benzothiazole (BZT-10). Yield (92%). Yellow solid. M. p. 167.2–168.0 °C (Lit:61 169.0 °C). 1H NMR (DMSO-d6, 200 MHz): 7.00 (d, 1H, J = 7.4 Hz), 7.32–7.55 (m, 5H), 8.07 (t, 2H, J = 8.3 Hz), 9.89 (s, 1H). 13C NMR (DMSO-d6, 50 MHz): δ 113.4, 118.0, 118.4, 122.1, 122.8, 125.3, 126.5, 130.4, 134.0, 134.3, 153.4, 157.9, 167.3. IR (KBr): 3058, 2698, 2564, 1600, 1480, 1446, 1294, 1270, 1242, 996, 886, 760 cm−1. LCMS-IT-TOF calculated for C13H10NOS [M + H]+: 228.0478, found 228.0398.
2-(2-Hydroxyphenyl)benzothiazole (BZT-11). Yield (100%). Yellow solid. M. p. 126.7–127.1 °C (Lit:61 127.0–128.0 °C). 1H NMR (DMSO-d6, 200 MHz): δ 6.97–7.12 (m, 2H), 7.37–7.57 (m, 3H), 8.04–8.17 (m, 3H), 11.67 (sl, 1H). 13C NMR (DMSO-d6, 50 MHz): δ 116.9, 118.2, 119.6, 121.9, 122.0, 125.0, 126.4, 128.4, 132.4, 134.1, 151.3, 156.4, 165.3. IR (KBr): 3058, 2996, 2938, 1624, 1590, 1488, 1316, 1272, 1220, 974, 818, 756, 742 cm−1. LCMS-IT-TOF calculated for C13H10NOS [M + H]+: 228.0478, found: 228.0388.
2-(2-Thienyl)benzothiazole (BZT-12). Yield (95%). Yellow solid. M. p. 92.4–94.1 °C (Lit:59 92.0–94.0 °C). 1H NMR (DMSO-d6, 200 MHz): δ 7.20–7.24 (m, 1H), 7.38–7.55 (m, 2H), 7.80–7.86 (m, 2H), 7.97–8.09 (m, 2H). 13C NMR (DMSO-d6, 50 MHz): δ 122.2, 122.4, 125.4, 126.6, 128.6, 129.5, 130.7, 134.1, 136.3, 153.0, 160.8. IR (KBr): 3096, 3056, 1542, 1476, 1312, 1222, 912, 852, 826, 762, 714 cm−1. LCMS-IT-TOF calculated for C11H8NS2 [M + H]+: 218.0093, found: 217.9985.
2-(2-Pyrrolyl)benzothiazole (BZT-13). Yield (98%). Brown solid. M. p. 153.4–155.3 °C (Lit:62 158.0–160.0 °C). 1H NMR (DMSO-d6, 200 MHz): δ 6.24 (sl, 1H), 6.85 (sl, 1H), 7.05 (sl, 1H), 7.35–7.47 (m, 2H), 7.89–8.01 (m, 2H), 12.12 (s, 1H). 13C NMR (DMSO-d6, 50 MHz): δ 110.0, 112.3, 121.5, 121.9, 123.1, 124.3, 125.5, 126.2, 133.4, 153.4, 159.8. IR (KBr): 3154, 3124, 3006, 2860, 1610, 1572, 1488, 1440, 1398, 1102, 912, 762, 740 cm−1. LCMS-IT-TOF calculated for C11H9N2S [M + H]+: 201.0481, found: 201.0402.
2-(2-Furfuryl)benzothiazole (BZT-14). Yield (92%). Brown solid. M. p. 102.2–104.0 °C (Lit:57 102.0–103.0 °C). 1H NMR (DMSO-d6, 200 MHz): δ 6.76–6.78 (m, 1H), 7.34–7.57 (m, 3H), 7.99–8.13 (m, 3H). 13C NMR (DMSO-d6, 50 MHz): δ 111.8, 113.0, 122.2, 122.6, 125.3, 126.7, 133.6, 146.0, 147.9, 153.3, 156.7. IR (KBr): 3144, 3122, 3050, 1598, 1578, 1434, 1246, 1114, 898, 748, 730 cm−1. LCMS-IT-TOF calculated for C11H8NOS [M + H]+: 202.0321, found: 202.0281.
2-(4-Pyridyl)benzothiazole (BZT-15). Yield (90%). Beige solid. M. p. 129.8–131.2 °C (Lit:63 130.0–132.0 °C). 1H NMR (DMSO-d6, 200 MHz): δ 7.59 (sl, 2H), 8.01–8.20 (m, 4H), 8.80 (sl, 2H). 13C NMR (DMSO-d6, 50 MHz): δ 120.8, 122.5, 123.5, 126.2, 126.9, 134.7, 139.4, 150.8, 153.2, 164.8. IR (KBr): 3052, 3026, 1598, 1588, 1476, 1312, 1214, 980, 820, 756, 704 cm−1. LCMS-IT-TOF calculated for C12H9N2S [M + H]+: 213.0481, found: 213.0422.
2-(3-Pyridyl)benzothiazole (BZT-16). Yield (90%). Yellow solid. M. p. 124.4–125.7 °C (Lit:64 137.0–138.0 °C). 1H NMR (DMSO-d6, 200 MHz): δ 7.44–7.62 (m, 3H), 8.07–8.18 (m, 2H), 8.41 (d, 1H, J = 7.8 Hz), 8.73–8.75 (m, 1H), 9.25 (sl, 1H). 13C NMR (DMSO-d6, 50 MHz): δ 122.4, 123.0, 124.2, 125.8, 126.7, 128.8, 134.4, 134.5, 147.6, 151.8, 153.3, 164.4. IR (KBr): 3050, 3032, 1586, 1574, 1426, 1310, 1234, 964, 814, 766, 702 cm−1. LCMS-IT-TOF calculated for C12H9N2S [M + H]+: 213.0481, found: 213.0399.
2-(2-Carboxyphenyl)benzothiazole (BZT-17). Yield (90%). Yellow solid. M. p. 179.3–180.7 °C (Lit:65 177.0–178.0). 1H NMR (DMSO-d6, 200 MHz): δ 7.47–7.80 (m, 6H), 8.03 (d, 1H, J = 7.4 Hz), 8.15 (d, 1H, J = 7.2 Hz). 13C NMR (DMSO-d6, 50 MHz): δ 122.1, 122.9, 125.4, 126.4, 129.2, 130.4, 131.0, 132.3, 133.2, 135.5, 153.1, 166.3, 168.7. IR (KBr): 3066, 2866, 2756, 2582, 2466, 1702, 1592, 1438, 1318, 1254, 1200, 976, 770, 752 cm−1. LCMS-IT-TOF calculated for C14H10NO2S [M + H]+: 256.0427, found: 256.0321.
2-(1,3-Benzodioxol-5-yl)benzothiazole (BZT-18). Yield (100%). Yellow solid. M. p. 118.4–119.9 °C (Lit:66 125.0–126.0 °C). 1H NMR (CDCl3, 200 MHz): δ 6.03 (s, 2H), 6.88 (d, 1H, J = 8.4 Hz), 7.25–7.50 (m, 2H), 7.55–7.60 (m, 2H), 7.85 (d, 1H, J = 7.6 Hz), 8.02 (d, 1H, J = 7.6 Hz). 13C NMR (CDCl3, 50 MHz): δ 101.7, 107.5, 108.6, 121.4, 122.4, 122.9, 124.9, 126.2, 128.0, 134.8, 148.3, 150.0, 154.1, 167.5. IR (KBr): 3058, 2996, 2908, 2784, 1604, 1512, 1474, 1442, 1256, 1032, 928, 882, 806, 756, 728 cm−1. LCMS-IT-TOF calculated for C14H10NO2S [M + H]+: 256.0427, found: 256.0383.
2-Cyclohexyl-benzothiazole (BZT-19). Yield (94%). Yellow oil (Lit:67 yellow oil). 1H NMR (CDCl3, 200 MHz): δ 1.27–1.93 (m, 8H), 2.17–2.24 (m, 2H), 3.10 (tt, 1H, J = 11.6/3.6), 7.28–7.48 (m, 2H), 7.84 (dd, 1H, J = 7.6/0.9 Hz), 7.97 (dd, 1H, J = 8.2/1.0 Hz). 13C NMR (CDCl3, 50 MHz): δ 25.7, 26.0, 33.4, 43.4, 121.5, 122.5, 124.4, 125.7, 134.5, 153.1, 177.5. IR (KBr): 3062, 2928, 2852, 1516, 1438, 1314, 1246, 992, 758, 730 cm−1. LCMS-IT-TOF calculated for C13H16NS [M + H]+: 218.0998, found: 218.0970.
Urease inhibition assay
The screening for identifying potential urease inhibitors was done using the indophenol method.68 Each BZT at final concentration of 1.6 mM was incubated in a medium containing 20 mM phosphate buffer (pH 7.0), 1 mM EDTA, 10 mM urea and 12.5 mU of Canavalia ensiformis type III urease. Reactions were maintained at 25 °C for 15 min, followed by addition of 0.5 volume of 1% w/v phenol/5 ppm sodium nitroprusside (SNP) and 0.7 volume of 0.5% w/v NaOH/0.1% v/v NaOCl solution to interrupt enzyme activity. Reactions were then incubated at 50 °C for 5 min prior the measurement of media absorbance at 630 nm to determine the amount of ammonium (NH4+) formed. Hydroxyurea (HU) and thiourea (TU) were used as reference of urease inhibitors. Urease inhibition was determined in terms of percentage of NH4+ formed in compound-test-containing reactions in relation to total urease activity in reactions devoid of inhibitor.
Kinetic assays with jack bean type III urease
The effect of BZTs synthesized on the kinetic parameters of jack bean type III urease was investigated monitoring NH4+ formation in reactions containing or not the BZT at varied concentration and increasing concentrations of urea (1–32 mM). The procedure was similar to that described for assessing the urease inhibition, except that the reaction time was set to 10 min. Kinetic parameters for urease in the absence or presence of BZT were obtained using Hyper32 software. Michaelis–Menten hyperbolas and Lineweaver–Burk plots were obtained using the OriginPro 8 software.
Soil ureases activity assay
The effect activity of soil ureases was assessed using the salicylate method.69 Clayey dystrophic Red Latosol (oxisol) soil was collected from Brazilian Cerrado (19°28′01.2′′S, 44°10′24.5′′W). The physical features of the collected soil were 6% coarse sand, 4% fine sand, 12% silt, and 78% clay and chemical analyses showed pH 6.3, 10 mg dm−3 PMehlich-1, 129 mg dm−3 K, 4.4 cmolc dm−3 Ca, 0.9 cmolc dm−3 Mg, 0.1 cmolc dm−3 Al, 2.6 cmolc dm−3 H + Al, sum of bases of 5.6 cmolc dm−3, 68% base saturation, organic matter of 2.5 dag kg−1. Sieved soil (0.5 g; < 2.0 mm particles) were incubated with 72 mM urea in the presence or absence of each BZT (1.6 mM) at 37 °C for 1 h. The activity of soil ureases was stopped by the addition of KCl 1 M/HCl 10 mM solution (5 mL). After 30 min incubation at 25 °C, a supernatant volume was collected and added to a mixture containing 3.4% sodium salicylate, 2.5% sodium citrate, 2.5% sodium tartrate and 120 ppm SNP. Systems were incubated for further 15 min at 25 °C and under darkness. Then, 0.1 volume of 3.0% NaOH/1.0% NaOCl solution was added to each system following incubation under darkness for 1 h at 25 °C and stirring (600 rpm). Spectrophotometric measurements were carried out at 660 nm to estimate NH4+ formed in the reaction media. The NBPT was used as a reference of urease inhibitor active on soil microbiota.
Acknowledgements
Authors are grateful to Dr Ivanildo E. Marriel (EMBRAPA Maize and Sorghum, Brazil) for providing soil samples and related physicochemical characterization data. This work was made possible by the Network for the Development of Novel Urease Inhibitors (www.redniu.org) which is financially supported by the Brazilian agencies CNPq, CAPES and FAPEMIG. LVM and AF are recipients of research fellowships from CNPq.
Notes and references
- P. Hrobárik, I. Sigmundová, P. Zahradník, P. Kasák, V. Arion, E. Franz and K. Clays, J. Phys. Chem. C, 2010, 114, 22289–22302 Search PubMed.
- V. Facchinetti, R. R. Reis, C. R. B. Gomes and T. R. A. Vasconcelos, Mini-Rev. Org. Chem., 2012, 9, 44–53 CrossRef CAS.
- M. N. Noolvi, H. M. Patel and M. Kaur, Eur. J. Med. Chem., 2012, 54, 447–462 CrossRef CAS PubMed.
- M. Henary, S. Paranjpe and E. A. Owens, Heterocycl. Commun., 2013, 19, 1–11 CrossRef CAS.
- R. S. Keri, M. R. Patil, S. A. Patil and S. Budagumpi, Eur. J. Med. Chem., 2015, 89, 207–251 CrossRef CAS PubMed.
- I. Fishtner, A. Monks, C. Hose, M. F. G. Stevens and T. D. Bradshaw, Breast Cancer Res. Treat., 2004, 87, 97–107 CrossRef PubMed.
- C. G. Mortimer, G. Wells, J.-P. Crochard, E. L. Stone, T. D. Bradshaw, M. F. G. Stevens and A. D. Westwell, J. Med. Chem., 2006, 49, 179–185 CrossRef CAS PubMed.
- S. Aiello, G. Wells, E. L. Stone, H. Kadri, R. Bazzi, D. R. Bell, M. F. G. Stevens, C. S. Matthews, T. D. Bradshaw and A. D. Westwell, J. Med. Chem., 2008, 51, 5135–5139 CrossRef CAS PubMed.
- A. Cifra, G. L. Mazzone and A. Nistri, Neuroscience, 2012, 19, 137–144 CrossRef PubMed.
- W. Huang, P.-L. Zhao, C.-L. Liu, Q. Chien, Z.-M. Liu and G.-F. Yang, J. Agric. Food Chem., 2007, 55, 3004–3010 CrossRef CAS PubMed.
- Y. Gull, N. Rasool, M. Noreen, F.-H. Nasim, A. Yaqoob, S. Kousar, U. Rashid, I. H. Bukhari, M. Zubair and M. S. Islam, Molecules, 2013, 18, 8845–8857 CrossRef CAS PubMed.
- N. P. Prajapati, R. H. Vekariya, M. A. Borad and H. D. Patel, RSC Adv., 2014, 4, 60176–60208 RSC.
- A. Gupta and S. Rawat, Int. J. Curr. Pharm. Res., 2010, 3, 13–23 Search PubMed.
- H. Y. Guo, J. C. Li and Y. L. Shang, Chin. Chem. Lett., 2009, 20, 1408–1410 CrossRef CAS.
- P. S. Chandrachood, D. R. Garud, T. V. Gadakari, R. C. Torane, N. R. Deshpande and R. V. Kashalkar, Acta Chim. Slov., 2011, 58, 367–371 CAS.
- K. Bahrami, M. M. Khodaei and F. Naali, J. Org. Chem., 2008, 73, 6835–6837 CrossRef CAS PubMed.
- X. Fan, Y. Wang, Y. He, X. Zhang and J. Wang, Tetrahedron Lett., 2010, 51, 3493–3496 CrossRef CAS.
- A. J. Blacker, M. M. Farah, M. I. Hall, S. P. Marsden, O. Saidi and J. M. J. Williams, Org. Lett., 2009, 11, 2039–2042 CrossRef CAS PubMed.
- L. Y. Fan, Y. H. Shang, X. X. Li and W. J. Hua, Chin. Chem. Lett., 2015, 26, 77–80 CrossRef CAS.
- G.-F. Chen, H.-M. Jia, L.-Y. Zhang, B.-H. Chen and J.-T. Li, Ultrason. Sonochem., 2013, 20, 627–632 CrossRef CAS PubMed.
- M. Mohammadi, G. R. Bardajee and N. N. Pesyan, RSC Adv., 2014, 4, 62888–62894 RSC.
- J. N. Sangshetti, N. D. Kokare, S. A. Kotharkar and D. B. Shinde, Monatsh. Chem., 2008, 139, 125–127 CrossRef CAS.
- H. Wang, X. Zhu, Y. Lu, Y. Li and X. Gao, Chin. J. Chem., 2011, 29, 1180–1184 CrossRef CAS.
- J. L. Yu, H. Wang, K. F. Zou, J. R. Zhang, X. Gao, D. W. Zhang and Z. T. Li, Tetrahedron, 2013, 69, 310–315 CrossRef CAS.
- J. N. Sangshetti, A. R. Chabukswar and D. B. Shinde, Bioorg. Med. Chem. Lett., 2011, 21, 444–448 CrossRef CAS PubMed.
- S. N. Darandale, J. N. Sangshetti and D. B. Shinde, J. Korean Chem. Soc., 2012, 56, 328–333 CrossRef CAS.
- Z. Z. Huang, S. Ye, W. Xia and Y. Tang, Chem. Commun., 2001, 1384–1385 RSC.
- H. F. Ridley, R. G. W. Spickett and G. M. Timmis, J. Heterocycl. Chem., 1965, 2, 453–456 CrossRef CAS.
- C. Follmer, Phytochemistry, 2008, 69, 18–28 CrossRef CAS PubMed.
- H. L. Mobley and R. P. Hausinger, Microbiol. Mol. Biol. Rev., 1995, 59, 451–480 CAS.
- R. A. Burne and Y. Y. M. Chen, Microbes Infect., 2000, 2, 533–542 CrossRef CAS PubMed.
- B. Krajewska, J. Mol. Catal. B: Enzym., 2009, 59, 9–21 CrossRef CAS.
- M. J. Maroney and S. Ciurli, Chem. Rev., 2014, 114, 4206–4228 CrossRef CAS PubMed.
- J. L. Boer, S. B. Mulrooney and R. P. Hausinger, Arch. Biochem. Biophys., 2014, 544, 142–152 CrossRef CAS PubMed.
- H. M. S. Algood and T. L. Cover, Clin. Microbiol. Rev., 2006, 19, 597–613 CrossRef CAS PubMed.
- T.-W. Woo, M.-S. Chang, Y.-K. Chung, K.-B. Kim, S.-K. Sohn, S.-G. Kim and W.-S. Choi, Arch. Pharmacal Res., 1998, 21, 6–11 CrossRef CAS.
- J. S. Williamson, Curr. Pharm. Des., 2001, 7, 357–394 CrossRef.
- C. Follmer, J. Clin. Pathol., 2010, 63, 424–430 CrossRef CAS PubMed.
- H. Azizian, F. Nabati, A. Sharifi, F. Siavoshi, M. Mahdavi and M. Amanlou, J. Mol. Model., 2012, 18, 2917–2927 CrossRef CAS PubMed.
- L. V. Modolo, A. X. Souza, L. P. Horta, D. P. Araujo and A. de Fátima, J. Adv. Res., 2015, 6, 35–44 CrossRef CAS PubMed.
- E. Artola, S. Cruchaga, I. Ariz, J. F. Moran, M. Garnica, F. Houdusse, J. M. G. Mina, I. Irigoyen, B. Lasa and P. M. A. Tejo, Plant Growth Regul., 2011, 63, 73–79 CrossRef CAS.
- K. C. Cameron, H. J. Di and J. L. Moir, Ann. Appl. Biol., 2013, 162, 145–173 CrossRef CAS.
- F. Langa, P. L. Cruz, A. L. Hoz, A. Díaz-Ortiz and E. Díaz-Ortiz, Contemp. Org. Synth., 1997, 4, 373–386 RSC.
- M. Nüchter, B. Ondruschka, W. Bonrath and A. Gum, Green Chem., 2004, 6, 128–141 RSC.
- C. O. Kappe, Chem. Soc. Rev., 2008, 37, 1127–1139 RSC.
- M. D. Bowman, J. L. Holcomb, C. M. Kormos, N. E. Leadbeater and V. A. Williams, Org. Process Res. Dev., 2008, 12, 41–57 CrossRef CAS.
- S. Caddick and R. Fitzmaurice, Tetrahedron, 2009, 65, 3325–3355 CrossRef CAS.
- S. E. López, J. Restrepo, B. Pérez, S. Ortiz and J. Salazar, Bull. Korean Chem. Soc., 2009, 30, 1628–1630 CrossRef.
- C. O. Kappe, Angew. Chem., Int. Ed., 2004, 43, 6250–6284 CrossRef CAS PubMed.
- P. Lidström, J. Tierney, B. Wathey and J. Westman, Tetrahedron, 2001, 57, 9225–9283 CrossRef.
- L. Y. Fan, Y. H. Shang, X. X. Li and W. J. Hua, Chin. Chem. Lett., 2014, 26, 77–80 CrossRef.
- U. R. Pratap, J. R. Mali, D. V. Jawale and R. A. Mane, Tetrahedron Lett., 2009, 50, 1352–1354 CrossRef CAS.
- Z. Wang, R. Tang and J. Li, Chin. J. Chem., 2011, 29, 314–320 CrossRef CAS.
- K. M. Khan, F. Rahim, S. A. Halim, M. Taha, M. Khan, S. Perveen, Z. Haq, M. A. Mesaik and M. I. Choudhary, Bioorg. Med. Chem., 2011, 19, 4286–4294 CrossRef CAS PubMed.
- I. H. Segel, Enzyme kinetics: behavior and analysis of rapid equilibrium and steady-state enzyme systems, John Wiley and Sons, New York, 1975 Search PubMed.
- S. Sinha, P. Chattopadhyay, I. Pan, S. Chatterjee, P. Chanda, D. Bandyopadhyay, K. Das and S. K. Sen, Afr. J. Biotechnol., 2009, 8, 6016–6027 CAS.
- B. S. Londhe, U. R. Pratap, J. R. Mali and R. A. Mane, Bull. Korean Chem. Soc., 2010, 31, 2329–2332 CrossRef CAS.
- A. A. Weekes, M. C. Dix, M. C. Bagley and A. A. Westwell, Synth. Commun., 2010, 40, 3027–3032 CrossRef CAS.
- N. Park, Y. Heo, M. R. Kumar, Y. Kim, K. H. Song and S. Lee, Eur. J. Org. Chem., 2012, 1984–1993 CrossRef CAS.
- K. Serdons, T. Verduyckt, D. Vanderghinste, J. Cleynhens, P. Borghgraef, P. Vermaelen, C. Terwinghe, F. Van Leuven, K. Van Laere, H. Kung, G. Bormans and A. Verbruggen, Bioorg. Med. Chem. Lett., 2009, 19, 602–605 CrossRef CAS PubMed.
- H. Sharghi and O. Asemani, Synth. Commun., 2009, 39, 860–867 CrossRef CAS.
- B. George and E. P. Papadopoulos, J. Org. Chem., 1977, 42, 441–443 CrossRef CAS.
- P. B. Gorepatil, Y. D. Mane and V. S. Ingle, Synlett, 2013, 24, 2241–2244 CrossRef CAS.
- T. G. Deligeorgiev, Dyes Pigm., 1990, 12, 243–248 CrossRef CAS.
- J. S. Baum and T. M. Chen, US Pat. 4556411, 1985.
- D. Shi, S. Rong and G. Dou, Synth. Commun., 2010, 40, 2302–2310 CrossRef CAS.
- G. H. Sung, I. Lee, B. R. Kim, D. Shin, J. Kim, S. Lee and Y. Yoon, Tetrahedron, 2013, 69, 3530–3535 CrossRef CAS.
- M. W. Weatherburn, Anal. Chem., 1967, 39, 971–974 CrossRef CAS.
- E. Kandeler and H. Gerber, Biol. Fertil. Soils, 1988, 6, 68–72 CrossRef CAS.
Footnote |
† Electronic supplementary information (ESI) available. See DOI: 10.1039/c5ra01081k |
|
This journal is © The Royal Society of Chemistry 2015 |
Click here to see how this site uses Cookies. View our privacy policy here.