DOI:
10.1039/C5RA00936G
(Paper)
RSC Adv., 2015,
5, 37346-37352
Biomimetic multi-layered hollow chitosan–tripolyphosphate rod with excellent mechanical performance
Received
16th January 2015
, Accepted 9th April 2015
First published on 9th April 2015
Abstract
In this work, chitosan–tripolyphosphate hydrogel and dry rods were prepared, which possessed biomimetic features, i.e. multi-layered and hollow features. The ratio of internal to external diameter could be designed and controlled. The relationship between the biomimetic hierarchical structure and mechanical performance was also explored in this research. The resulting rods with three-dimensional, organized structure and excellent mechanical performance have a great potential application in bone tissue engineering.
Introduction
Chitosan (CS), a polysaccharide obtained by the deacetylation of chitin, has received considerable attention due to its intrinsic properties: biocompatibility, biodegradability, bacteriostatic effects and abundance in nature.1–4 With applications pervading advanced industry and research, CS has been utilized in the preparation of various materials.5–10 The field of hydrogels is a major branch and represents a very important form of CS materials.11,12 CS hydrogel also provides a vital approach for the preparation of dry CS materials such as scaffold and bone fracture internal fixation devices.13–21 Therefore, the design of CS hydrogel is significant.22,23
In the design of CS hydrogel materials, the structures of natural materials have always been a source of inspiration. Biomimetic features could endow materials with excellent mechanical and functional performance. Multi-layered and hollow features are two successful examples. The multi-layered feature originates from natural structures such as annual rings of trees and nacre shells.24,25 This feature can improve the bending strength by stopping crack propagation.26 Moreover, the hierarchical structure has applications in bio-related areas such as tissue engineering and controlled release of drugs.27–30 The design of the hollow rod was inspired by bamboo and straw. Apart from mechanical benefits, the hollow feature enabled applications in blood vessel tissue engineering, spinal cord injury repair and nerve regeneration.31–33
In the design and fabrication of CS hydrogel, tripolyphosphate (TPPS) is a very popular ionic crosslinking agent because of its non-toxic properties and mild gelation process.34,35 The incorporation of TPPS also introduced benefits in resulting chitosan–tripolyphosphate (CS–TPPS) composite materials such as stability,36,37 antibacterial property,38 and advantages in bone tissue engineering. CS–TPPS composite materials could improve cellular responses and support biomineralization with phosphate as the nucleation site.39,40 TPPS was used for the preparation of crosslinked CS beads, gels, nanoparticles, and films.41–43 CS–TPPS materials with layered structures were mainly fabricated by alternate processes such as layer-by-layer self-assembly.36,38,44 To the best of our knowledge, the incorporation of TPPS in 3D CS rods with organized structures has not been reported. In this work, multi-layered hollow CS–TPPS hydrogel and dry rods were prepared by a very simple process, and the relationship between the multi-layered hollow structure and mechanical performance was also explored in the following section.
Experimental section
1. Materials
CS was purchased from Zhejiang Gold Shell Pharmaceutical Co. Ltd. The average viscosity molecular weight (Mη) of CS was 5.63 × 105 Da, and the degree of deacetylation (DD) was 91%. Sodium tripolyphosphate was purchased from Sinopharm Chemical Reagent Co., Ltd. The other chemical reagents used in the work were all of analytical reagent grade.
2. Preparation of multi-layered hollow CS–TPPS rods
CS powder was dissolved in 2 vol.% acetic acid aqueous solution to prepare CS solution (5 wt%). A cylindrical semipermeable membrane was used as a mold. Subsequently, the cylindrical mold was filled with CS solution, followed by precipitation in 5% (w/v) NaOH aqueous solution to form a CS gel rod. The CS gel rods were washed with deionized water repeatedly to remove OH−. The pH of the CS gel rods was modulated to be 6.5 by sulphuric acid, and then the rods were immersed in TPPS aqueous solution with the desired concentration for 12 h. The resulting rods were washed with deionized water repeatedly to remove the salt. Dry CS–TPPS rods were prepared by air-drying in an oven at 60 °C for 24 h. For the preparation of pure dry CS rods, CS gel rods were washed with deionized water repeatedly until neutral and then dried.
3. Relationship between gel thickness and precipitation time
CS solution (5 wt%) was poured into a single, open glass tube, and immersed in 5% (w/v) NaOH aqueous solution. The thickness of the precipitated CS gel was observed and recorded with time.
4. Scanning electron microscopy (SEM)
The fracture surface of the CS rod was observed by SEM. Samples were air-dried in an oven at 60 °C for 6 h to remove the remaining moisture and then gold-sprayed for conductance. A HITACHI S-4800 SEM was used in this study.
5. Fourier transform infrared spectroscopy (FTIR)
FTIR spectra of samples were obtained with a VECTOR 22 spectrophotometer (Bruker, Germany) in the wavenumber range of 2000–800 cm−1.
6. Thermogravimetric-derivative thermogravimetry (TG-DTG)
Thermal analyses were carried out by a Pyris-6 Thermo Analyser (Perkin-Elmer). Samples were heated from 50 to 600 °C at a heating rate of 20 °C min−1 under a constant flow of nitrogen (40 mL min−1).
7. Mechanical properties
The bending strength and modulus of CS or CS–TPPS rods were tested by the three point bending test on a universal mechanical testing apparatus (Shenzhen Reger Company, China). The span length was 40 mm and the loading rate was 2 mm min−1, and the ultimate bending strength (σb) and bending modulus (Eb) of the rods were calculated according to eqn (1) and (2), respectively45,46 |
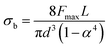 | (1) |
|
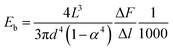 | (2) |
where Fmax is the maximum bending force recorded (N), L is the support span (mm), d is the external diameter of the rod (mm), α is the ratio of internal to external diameter, and ΔF/Δl is the slope of the force–deflection curve of the initial linear section (N mm−1).
Results and discussion
1. Formation of a multi-layered and hollow structure
The multi-layered, hollow CS–TPPS rod had a regular shape and smooth surfaces (Fig. 1). The hollow feature can be observed in Fig. 1b to d. Furthermore, the hollow rod possessed multi-layered features, and some of the layers were visible to the naked eye (Fig. 1b and c). During the drying process, swollen macromolecules started to shrink. This effect contributed to a more compact structure. As a result, multi-layers in the dry, hollow rod cannot be observed directly by visual inspection.
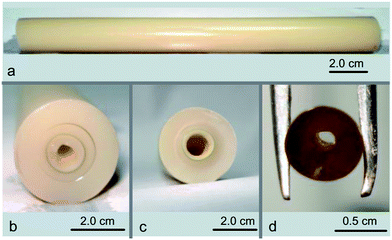 |
| Fig. 1 Digital images of a multi-layered hollow CS–TPPS rod. (a) Overall view of hydrogel rod; (b–d) cross section of rod: (b) hydrogel rod, (c) hydrogel rod after drying for 8 h, (d) dried rod. | |
CS became soluble in acetic acid aqueous solution because of the protonation of –NH2 groups.47–50 Moreover, the CS solution could be turned into hydrogel when it came in contact with alkali. The gelation process of this system possessed a layer-wise characteristic.51 As shown in Fig. 2a, when OH− diffused into the CS solution along a one-dimensional direction, the thickness of the hydrogel increased with time. The resulting hydrogel had multi-layers, which were parallel to the equipotential surface of c(OH−) (Fig. 2b). The formation of layers could be explained by the Liesegang ring phenomenon, caused by the encounter of an inner and an outer electrolyte and their periodical precipitation in the supporting medium. In this system, CS played the dual roles of the inner electrolyte and the supporting medium.
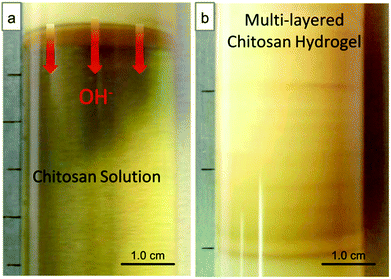 |
| Fig. 2 Layer-wise precipitation of CS solution: (a) diffusion direction of OH− to chitosan solution and (b) multi-layered chitosan hydrogel. | |
The design and construction of multi-layers in the rod is demonstrated below and is schematically shown in Scheme 1. The diffusion direction of OH− was arranged to be three-dimensional and was from the outer surface of the semipermeable membrane to the central axis. The equipotential surfaces of c(OH−) were cylindrical, and the resulting hydrogel layers were in the form of concentric cylinders, which formed multi-layered rods.
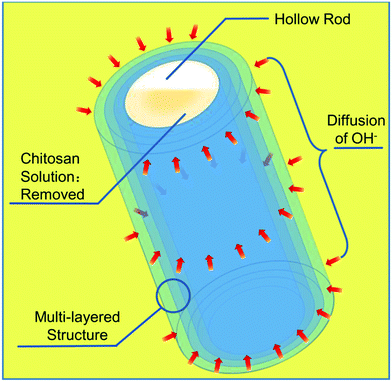 |
| Scheme 1 Preparation and structural features of multi-layered hollow CS–TPPS rod. | |
A multi-layered structure could be observed in the pure CS rod and the CS–TPPS rod by SEM (Fig. 3a and b). TPPS contains the P–O− moiety and could create ionic crosslinking points with the protonated amino moiety −NH3+. However, when the CS hydrogel was neutral, the proportion of −NH3+ was low, preventing the formation of effective crosslinks. On the other hand, a lower pH could increase the proportion of −NH3+ and its combination with TPPS; however, it may also destroy the multi-layered structure. Therefore, a proper pH was selected to make sure that the CS hydrogel was only slightly swollen, while being reactive with TPPS.52 The results showed that this method did not affect the shape of the hydrogel, and the multi-layered feature was successfully maintained in the CS–TPPS rod (Fig. 3b). Moreover, the interaction between CS and TPPS is discussed in the next section.
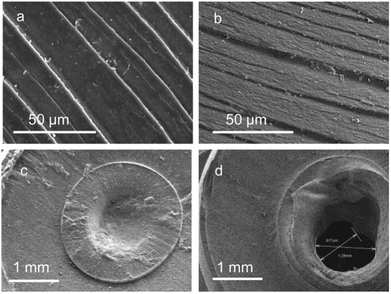 |
| Fig. 3 SEM images of CS rods: (a and b) multi-layered structure of CS and CS–TPPS rods, respectively; (c and d) cross section view of the central area in solid and hollow CS–TPPS rods, respectively. | |
The hollow feature of the CS–TPPS rod was constructed by utilizing the layer-wise gelation process, i.e. the relationship between gel thickness and time. The thickness of the gel did not grow linearly with time all along (Fig. 4). In the first hour, the gel thickness increased from 0 to 7 mm. In first 7 hours, the rate of increase of the gel thickness declined gradually, and the average rate was 2.71 mm h−1. The rate of increase of the gel thickness remained constant (0.33 mm h−1) after 7 h. This was because the rate of increase of the gel thickness was determined by the diffusion rate of OH− to the gel–sol interface. When the thickness of the gel was small (<10 mm), the c(OH−) in the gel had not reached equilibrium with that in the coagulation bath. Therefore, the coagulation bath was the major source of OH−.51 Thus, the thickness of the gel had a significant effect on the diffusion of OH−. With a longer precipitation time, the thickness of the gel increased and the c(OH−) in the upper part of the gel had reached the value in the coagulation bath.53 The upper part of the gel gradually became the source of OH− for the gel–sol interface. As a result, the distance between the coagulation bath and the interface had a slight impact on the increasing rate of gel thickness.
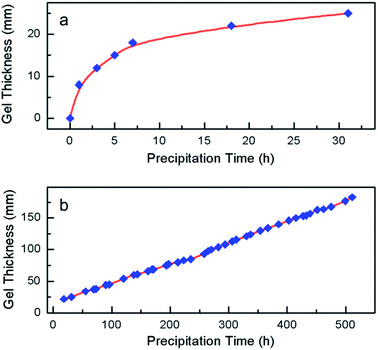 |
| Fig. 4 Gel thickness for different precipitation times: (a) 0–30 h, (b) 30–500 h. | |
An understanding of the relationship between gel thickness and precipitation time was crucial in the design of the hollow structure. When immersed in the NaOH coagulation bath for a sufficient time, all the CS solution finished precipitating. There was no space in the central area of the rod (Fig. 3c). However, if the precipitation was terminated before the gel thickness reached the radius of the cylindrical mold, a hollow rod was formed (Fig. 3d). The internal diameter of the hollow rod was the difference between the external radius and the gel thickness. Thus, by controlling the precipitation time, the ratio of internal diameter to external diameter (α) could be modulated.
2. Determination of ionic crosslinks between CS and TPPS
FTIR spectra of CS and CS–TPPS rods were analysed to determine the interaction between CS and TPPS (Fig. 5a). The absorption band in the high wavenumber region (>3000 cm−1) corresponded to the –O–H and –N–H stretching vibrations of CS, which was an overlapped broad band. Therefore, the analyzer focused on the region of 2000–800 cm−1. For a pure CS rod, the characteristic absorption peaks of CS were observed: 1655 cm−1 (ṼN–H, –CO–NH amide band I), 1598 cm−1 (δN–H, amide band II), 1423 cm−1 and 1378 cm−1 (ṼN–H, amide band III), 1155 cm−1 (ṼC–O), 1074 cm−1 (ṼC–O, –CH–OH), and 1030 cm−1 (ṼC–O, –CH2–OH). For a CS–TPPS rod, the characteristic absorption peaks of TPPS were observed at 1210–1082 cm−1 (ṼP–OṼP
O) and 879 cm−1(δP–O). Moreover, the characteristic absorption bands of CS between 1155 cm−1 and 1082 cm−1 merged with the absorption peaks of TPPS. These confirmed the successful incorporation of TPPS into the rod. In addition, stretching frequencies at 1655 cm−1 and 1598 cm−1 merged to become one peak with enhanced intensity, indicating that the reaction had occurred on the amino groups. Altogether, the FTIR spectra indicated that ionic crosslinks had formed between the protonated amine moiety −NH3+ of CS and the P–O− moiety of TPPS.
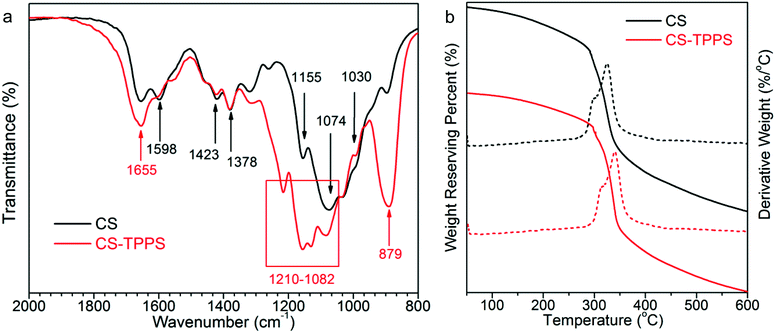 |
| Fig. 5 FTIR spectra (a) and TG/DTG curves (b) of CS and CS–TPPS rods. | |
To demonstrate the reaction between CS and TPPS, a thermal analysis on CS and CS–TPPS rods was conducted. The TG and DTG curves of CS and CS–TPPS rods are shown in Fig. 5b. The major weight losses observed at 250–450 °C were attributed to the decomposition of the CS. Compared with the CS rod, the TG curves of the CS–TPPS rod shifted to higher temperatures. As can be seen from the DTG curves, peaks of the maximum decomposition rates were observed at 324 and 338 °C for CS and CS–TPPS rods, respectively. This fact provided an additional evidence for the formation of ionic crosslinks.54
3. The relationship between biomimetic structure and mechanical performance
The mechanical properties of the CS–TPPS multi-layered hollow rods were tested. The influence of TPPS is demonstrated in Fig. 6a. Results showed that for samples with α = 0.17, the bending strength was in the range of 108.0–154.8 MPa, and the bending modulus was in the range of 4.1–5.6 GPa. These values were higher than those for pure CS rods. Moreover, with the increase of c(TPPS) in the crosslinking bath, the bending strength and modulus both increased. It can be concluded that the incorporation of TPPS enhanced the mechanical performance. Ionic crosslinks formed between CS and TPPS in addition to inter-/intro-molecular hydrogen bonds and improved the mechanical performance on a molecular level.55
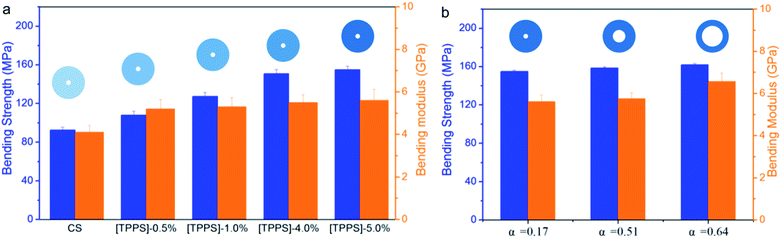 |
| Fig. 6 Mechanical properties of CS–TPPS multi-layered hollow rods. (a) The influence of the concentration of TPPS in the cross-linking bath, α = 0.17, (b) the influence of the ratio of internal diameter to external diameter, c(TPPS) = 5% in cross-linking bath; all samples had the same external diameter and length. | |
The influence of structural characteristics was also studied. The influence of the ratio of internal to external diameter (α) is demonstrated in Fig. 6b. The bending strength and modulus of the multi-layered hollow rod showed a tendency to increase with the rise of α. The shape of the cross section is very important for the mechanical properties of materials. Solid and hollow rods with the same cross sectional areas have different section modulus. The section modulus (WZ) is critical in the bending behaviour of rod materials, and a higher value of this parameter favours an improved bending strength.56 The section modulus ratio of a solid to a hollow rod is:
|
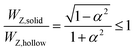 | (3) |
This indicated that the hollow rod always had a higher section modulus than the solid rod, which led to a higher bending strength. However, the discussion mentioned above was based on the premise that the two types of rods had the same cross sectional area, i.e. the same mass for a certain length. If hollow and solid rods had the same external diameter, the advantage of the hollow feature was in conflict with the decrease in effective cross sectional area (Scheme 2). The ratio of the bending modulus (σb) of a hollow rod to that of a solid rod is demonstrated in eqn (4):
|
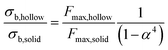 | (4) |
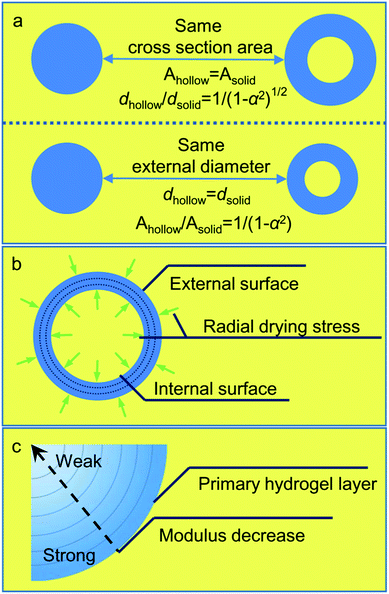 |
| Scheme 2 The influence of structural characteristics on chitosan rod. (a) Relationship between solid and hollow rods; (b) compression of drying stress in CS–TPPS multi-layered hollow rod; and (c) modulus gradient in CS hydrogel rod. | |
Although an increase in α is favourable in consideration of cross section shape, the maximum bending force (Fmax) may decrease due to the reduced cross sectional area. This situation resembles the decay of tree trunks, which results in an increased risk of failure.56
According to the analysis mentioned above, an increase in α was not favourable when the external diameter was fixed. However, it was noteworthy that a rise in α was actually advantageous, as demonstrated in Fig. 6b. If the mass of the rod was also taken into consideration, the advantage was evident as demonstrated by eqn (5).
|
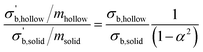 | (5) |
This phenomenon had two contributing factors: (1) the compression caused by drying stress and (2) the gradient of the modulus in CS hydrogel. (1) During the drying process, there was a difference in water content between the surface and the interior parts of the rod, thus creating drying stress towards the interior part.57 The radial drying stress was perpendicular to the cylindrical surface of the rod.58 Because the hollow rod had both external and internal surfaces, the radial stress created a compression effect (Scheme 2b) and led to a more compact structure. (2) CS hydrogel prepared by precipitation from an acidic solution was not a homogeneous material. The modulus of the hydrogel decreased with the increase of distance to the primary hydrogel layer.51 For the rod material, the primary hydrogel layer corresponded to the external surface of the cylinder, and thus the centre was the most distant point. Therefore, the modulus of the hydrogel decreased along the radial direction, as shown in Scheme 2c. The average modulus of the hydrogel in the 0–10 mm distance range was approximately twice the value of that in the 10–20 mm distance range.51 In the case of the present work, although the area of the cross section was decreased, the hydrogel near the external surface was preserved and had higher modulus than the average modulus of the solid rod.
Conclusion
In summary, a CS–TPPS rod with biomimetic multi-layered and hollow features was prepared. Moreover, these features were achieved in addition to excellent mechanical performance. The preparation was simple and was based on the controlled precipitation of CS solution. The external and internal diameters could both be designed and controlled. TPPS was successfully introduced into the 3D CS rod via ionic crosslinks. The inclusion of TPPS improved the mechanical performance while endowing properties such as benefits in bone tissue engineering. The multi-layered, hollow features greatly improved the mechanical performance of CS–TPPS rods. This material has potential applications in bio-related fields, especially in cases where both good mechanical performance and a sophisticated 3D structure are required.
Acknowledgements
This work was financially supported by the National Natural Science Foundation of China (nos 21104067, 21274127, 21374099 and 51473144), the Key Basic Research Development Plan (973 Program) of China (nos 2009CB930104 and 2011CB606203), the Science and Technology Project of Zhoushan City (no. 2011C12054), and the Key Science Technology Innovation Team of Zhejiang Province (no. 2013TD02).
Notes and references
- M. Rinaudo, Prog. Polym. Sci., 2006, 31, 603–632 CrossRef CAS PubMed.
- L. Chen, C. Y. Tang, N. Y. Ning, C. Y. Wang, Q. Fu and Q. Zhang, Chin. J. Polym. Sci., 2009, 27, 739–746 CrossRef CAS.
- J. W. Jia, Z. K. Wang, W. T. Lu, L. Yang, Q. W. Wu, W. Qin, Q. L. Hu and B. Z. Tang, J. Mater. Chem. B, 2014, 2, 8406–8411 RSC.
- K. Zhang, M. Zhao, L. Cai, Z. K. Wang, Y. F. Sun and Q. L. Hu, Chin. J. Polym. Sci., 2010, 28, 555–561 CrossRef CAS.
- M. Li, Y. N. Hong, Z. K. Wang, S. J. Chen, M. Gao, R. T. K. Kwok, W. Qin, J. W. Y. Lam, Q. C. Zheng and B. Z. Tang, Macromol. Rapid Commun., 2013, 34, 767–771 CrossRef CAS PubMed.
- Y. L. Li, P. Y. Zhuang, Y. F. Zhang, Z. K. Wang and Q. L. Hu, Mater. Lett., 2012, 84, 73–76 CrossRef CAS PubMed.
- Y. F. Sun, Y. L. Li, J. Y. Nie, Z. K. Wang and Q. L. Hu, Chem. Lett., 2013, 42, 838–840 CrossRef CAS.
- Z. K. Wang, S. J. Chen, J. W. Y. Lam, W. Qin, R. T. K. Kwok, N. Xie, Q. L. Hu and B. Z. Tang, J. Am. Chem. Soc., 2013, 135, 8238–8245 CrossRef CAS PubMed.
- K. Zhang, Z. K. Wang, Y. L. Li, Z. Q. Jiang, Q. L. Hu, M. Y. Liu and Q. X. Zhao, Carbohydr. Polym., 2013, 92, 662–667 CrossRef CAS PubMed.
- K. Zhang, P. Y. Zhuang, Z. K. Wang, Y. L. Li, Z. Q. Jiang, Q. L. Hu, M. Y. Liu and Q. X. Zhao, Carbohydr. Polym., 2012, 90, 1515–1521 CrossRef CAS PubMed.
- A. El Kadib, M. Bousmina and D. Brunel, J. Nanosci. Nanotechnol., 2014, 14, 308–331 CrossRef CAS PubMed.
- S. D. Ray, Acta Pol. Pharm., 2011, 68, 619–622 CAS.
- T. S. Anirudhan and A. M. Mohan, RSC Adv., 2014, 4, 12109–12118 RSC.
- H. Liu and C. Y. Wang, RSC Adv., 2014, 4, 3864–3872 RSC.
- X. M. Pu, Z. Z. Sun, Z. Q. Hou, Y. Yang, Q. Q. Yao and Q. Q. Zhang, J. Biomed. Mater. Res., Part B, 2012, 100, 1179–1189 CrossRef PubMed.
- J. Z. Zhang, J. Y. Nie, Q. R. Zhang, Y. L. Li, Z. K. Wang and Q. L. Hu, J. Biomater. Sci., Polym. Ed., 2014, 25, 61–74 CrossRef CAS PubMed.
- J. H. Ke, Z. K. Wang, Y. Z. Li, Q. L. Hu and J. Feng, Chin. J. Polym. Sci., 2012, 30, 436–442 CrossRef CAS PubMed.
- X. Wang, Y. Gao, H. Zhao, X.-Q. Liu, Z. Wang, A. Qin, Q. Hu, J. Z. Sun and B. Z. Tang, Polym. Chem., 2014, 5, 6216–6224 RSC.
- Z. K. Wang and Q. L. Hu, Biomed. Mater., 2010, 5, 045007 CrossRef PubMed.
- Z. K. Wang, Q. L. Hu, X. G. Dai, H. Wu, Y. X. Wang and J. C. Shen, Polym. Compos., 2009, 30, 1517–1522 CrossRef CAS PubMed.
- Z. K. Wang, H. Zhao, L. Fan, J. Lin, P. Y. Zhuang, W. Z. Yuan, Q. L. Hu, J. Z. Sun and B. Z. Tang, Carbohydr. Polym., 2011, 84, 1126–1132 CrossRef CAS PubMed.
- J. Berger, M. Reist, J. M. Mayer, O. Felt and R. Gurny, Eur. J. Pharm. Biopharm., 2004, 57, 35–52 CrossRef CAS.
- J. Berger, M. Reist, J. M. Mayer, O. Felt, N. A. Peppas and R. Gurny, Eur. J. Pharm. Biopharm., 2004, 57, 19–34 CrossRef CAS.
- M. Suzuki and H. Nagasawa, Can. J. Zool., 2013, 91, 349–366 CrossRef CAS PubMed.
- S. S. Wang, Y. Q. Shu, B. L. Liang, L. C. Gao, M. Gao, P. G. Yin and L. Guo, Chin. J. Polym. Sci., 2014, 32, 675–680 CrossRef CAS PubMed.
- Z. K. Wang, Q. L. Hu and Y. X. Wang, Sci. China: Chem., 2011, 54, 380–384 CrossRef CAS.
- K. Lee, G. Jin, C. H. Jang, W. K. Jung and G. Kim, J. Mater. Chem. B, 2013, 1, 5831–5841 RSC.
- T. J. Levingstone, A. Matsiko, G. R. Dickson, F. J. O'Brien and J. P. Gleeson, Acta Biomater., 2014, 10, 1996–2004 CrossRef CAS PubMed.
- B. B. Hsu, S. R. Hagerman, K. Jamieson, J. Veselinovic, N. O'Neill, E. Holler, J. Y. Ljubimova and P. T. Hammond, Biomacromolecules, 2014, 15, 2049–2057 CrossRef CAS PubMed.
- B. Mu, P. Liu, Y. Dong, C. Y. Lu and X. L. Wu, J. Polym. Sci., Part A: Polym. Chem., 2010, 48, 3135–3144 CrossRef CAS PubMed.
- K. Haastert-Talini, S. Geuna, L. B. Dahlin, C. Meyer, L. Stenberg, T. Freier, C. Heimann, C. Barwig, L. F. V. Pinto, S. Raimondo, G. Gambarotta, S. R. Samy, N. Sousa, A. J. Salgado, A. Ratzka, S. Wrobel and C. Grothe, Biomaterials, 2013, 34, 9886–9904 CrossRef CAS PubMed.
- X. G. Li, Z. Y. Yang, A. F. Zhang, T. L. Wang and W. C. Chen, Biomaterials, 2009, 30, 1121–1132 CrossRef CAS PubMed.
- J. R. Zhang, L. K. Yi and J. C. Zhang, Neural Regener. Res., 2010, 5, 668–672 Search PubMed.
- P. Sun, P. Li, Y. M. Li, Q. Wei and L. H. Tian, J. Biomed. Mater. Res., Part B, 2011, 97, 175–183 CrossRef PubMed.
- Y. L. Hu, W. Qi, F. Han, J. Z. Shao and J. Q. Gao, Int. J. Nanomed., 2011, 6, 3351–3359 CAS.
- S. W. Ali, M. Joshi and S. Rajendran, AATCC Rev., 2011, 11, 49–55 CAS.
- P. Sacco, M. Borgogna, A. Travan, E. Marsich, S. Paoletti, F. Asaro, M. Grassi and I. Donati, Biomacromolecules, 2014, 15, 3396–3405 CrossRef CAS PubMed.
- A. R. Shirvan, N. H. Nejad and A. Bashari, Fibers Polym., 2014, 15, 1908–1914 CrossRef CAS.
- M. Kucharska, K. Walenko, B. Butruk, T. Brynk, M. Heljak and T. Ciach, Mater. Lett., 2010, 64, 1059–1062 CrossRef CAS PubMed.
- F. Pati, H. Kalita, B. Adhikari and S. Dhara, J. Biomed. Mater. Res., Part A, 2013, 101, 2526–2537 CrossRef PubMed.
- L. Jin, X. Zeng, M. Liu and N. Y. He, Sci. Adv. Mater., 2013, 5, 2053–2057 CrossRef CAS PubMed.
- A. Rampino, M. Borgogna, P. Blasi, B. Bellich and A. Cesaro, Int. J. Pharm., 2013, 455, 219–228 CrossRef CAS PubMed.
- A. Anitha, N. Deepa, K. P. Chennazhi, S. V. Nair, H. Tamura and R. Jayakumar, Carbohydr. Polym., 2011, 83, 66–73 CrossRef CAS PubMed.
- S. Shenvi, A. F. Ismail and A. M. Isloor, Desalination, 2014, 344, 90–96 CrossRef CAS PubMed.
- A. Saikku-Backstrom, R. M. Tulamo, T. Pohjonen, P. Tormala, J. E. Raiha and P. Rokkanen, J. Mater. Sci.: Mater. Med., 1999, 10, 1–8 CrossRef CAS.
- K. Shen and Q. L. Hu, Mater. Lett., 2011, 65, 1503–1505 CrossRef CAS PubMed.
- Z. K. Wang, Q. L. Hu and L. Cai, Chin. J. Polym. Sci., 2010, 28, 801–806 CrossRef CAS.
- C. K. S. Pillai, W. Paul and C. P. Sharma, Prog. Polym. Sci., 2009, 34, 641–678 CrossRef CAS PubMed.
- Z. K. Wang and Q. L. Hu, Acta Phys.-Chim. Sin., 2010, 26, 2053–2056 CAS.
- Z. K. Wang, Q. L. Hu and L. Cai, Int. J. Polym. Sci., 2010, 2010, 369759 Search PubMed.
- J. Nie, W. Lu, J. Ma, L. Yang, Z. Wang, A. Qin and Q. Hu, Sci. Rep., 2015, 5, 7635 CrossRef PubMed.
- M. Rinaudo, G. Pavlov and J. Desbrieres, Polymer, 1999, 40, 7029–7032 CrossRef CAS.
- H. Zhang and W. Davison, Anal. Chim. Acta, 1999, 398, 329–340 CrossRef CAS.
- F. S. Pereira, D. L. D. Agostini, A. E. Job and E. R. P. Gonzalez, J. Therm. Anal. Calorim., 2013, 114, 321–327 CrossRef CAS.
- A. Aryaei, A. H. Jayatissa and A. C. Jayasuriya, J. Mech. Behav. Biomed. Mater., 2012, 5, 82–89 CrossRef CAS PubMed.
- A. Koizumi and T. Hirai, J. Wood Sci., 2006, 52, 213–219 CrossRef PubMed.
- B. Clair, Holzforschung, 2012, 66, 349–353 CrossRef CAS.
- W. Cheng, T. Morooka, Q. Wu and Y. Liu, For. Prod. J., 2007, 57, 39–43 Search PubMed.
Footnote |
† J. Y. Nie and Z. K. Wang contributed equally to this work. |
|
This journal is © The Royal Society of Chemistry 2015 |