DOI:
10.1039/C4RA13233E
(Paper)
RSC Adv., 2015,
5, 17623-17635
H2CO3 → CO2 + H2O decomposition in the presence of H2O, HCOOH, CH3COOH, H2SO4 and HO2 radical: instability of the gas-phase H2CO3 molecule in the troposphere and lower stratosphere†
Received
27th October 2014
, Accepted 20th January 2015
First published on 20th January 2015
Abstract
To understand the stability of the gas-phase carbonic acid (H2CO3) molecule, especially in the Earth's troposphere and lower stratosphere, here we report high level quantum chemistry calculations investigating the energetics for the H2CO3 → CO2 + H2O decomposition reaction via its shortest route in the presence of one to three water (H2O) molecules as well as in the presence of formic acid (FA), acetic acid (AA), sulfuric acid (SA) and hydroperoxide (HO2) radical. The calculations have been performed at the MP2/aug-cc-pVDZ, MP2/aug-cc-pVTZ, MP2/6-311++G(3df,3pd) and CCSD(T)/aug-cc-pVTZ levels of theory. The comparison of the reaction rates including tunneling corrections according to the unsymmetrical Eckart potential barriers suggests that at 0 km altitude in the clean environment of the Earth's atmosphere, the gaseous H2CO3 molecule becomes an unstable species in the presence of the H2O monomer, dimer, FA and AA. This follows as the FA- and AA-assisted H2CO3 → CO2 + H2O decomposition reactions are effectively near-barrierless processes and the reaction rates for the H2O monomer-, dimer-, FA- and AA-assisted H2CO3 decomposition reactions are comparable within a factor of ∼15. Similarly, at 0 km altitude in a polluted environment and also in the 5 to 15 km altitude range, only the FA- or AA-assisted H2CO3 decomposition is the dominant reaction pathway, especially, among all the pathways that have been considered here. It is seen from the CCSD(T)/aug-cc-pVTZ level prediction results that at altitudes of 5, 10, and 15 km in the Earth's atmosphere, the reaction rates for the FA-assisted H2CO3 decomposition depending upon the average concentrations of FA are respectively ∼102, 105 and 106 times higher than the reaction rates associated with the water monomer-assisted H2CO3 decomposition. Moreover, it is thought that the catalytic efficiencies of FA, AA and SA upon the H2CO3 → CO2 + H2O decomposition reaction are similar to each other, but nevertheless, SA, because of its low concentration, does not play a significant role in the H2CO3 → CO2 + H2O decomposition reaction, especially in the 0 to 15 km altitude range of the Earth's atmosphere.
1. Introduction
Carbonic acid (H2CO3) has been considered a molecule of profound astrophysical as well as environmental significance as both the H2O and CO2 molecules coexist in various astrophysical environments such as in ice grain mantles in interstellar medium and/or in outer space.1–33 This molecule was long believed to be an unstable and elusive species as it decomposes rapidly into CO2 and H2O molecules.1,34,35 Indeed, it was also believed in the past that the isolated H2CO3 molecule cannot exist in a free state, and consequently, the existence of isolated H2CO3 molecules in the gas-phase was controversial for a long time.2 In 1987, Terlouw et al.1 first detected free H2CO3 molecule in the gas-phase from the thermolysis of the ammonium bicarbonate (NH4HCO3) molecule via mass spectrometry, and hence, thereafter, the evidence for the possible existence of the H2CO3 molecule in the gas-phase was established. Subsequently, in the next two and half decades, the gaseous H2CO3 molecule has also been characterized in the laboratory by means of its microwave3,4 and infrared5,6,36 spectra. Moreover, the H2CO3 molecule has also been synthesized in various experimental conditions in the laboratory similar to those encountered in the extraterrestrial space.7–15,37 It is worth noting here that the H2CO3 molecule is believed to be present in cirrus clouds of the Earth's atmosphere, on the surface and in the atmosphere of Venus and Mars, as well as in Comets and the Galilean satellites.2,5,6,21,28–30 As noted by Kohl et al.,28 a comparison of some spectra on Mars with the IR spectrum of β-H2CO3, which is known to date as the distinct polymorph of H2CO3,5,6,21,26–30,36 suggests that β-H2CO3 is present on the Martian surface.20
Given that the H2CO3 monomer has been detected in various experimental conditions in the laboratory similar to those encountered in the extraterrestrial space, it is surprising that this molecule has not been detected yet in the Earth's atmosphere or in outer space.2,6,15 Indeed, we note what has already been emphasized by Huber et al.,2 Bernard et al.6 and Hudson et al.22 that the detection of gas-phase H2CO3 molecule in the Earth's troposphere and as well as in outer space has become very challenging for a new generation of scientists.2,5,6,22 Recently, we have demonstrated that the primary mechanism for the decomposition of the H2CO3 molecule into its constituents CO2 and H2O molecules, especially at its source where the vapor phase concentration of the H2CO3 molecule reaches its highest level, is autocatalytic.38 In other words, the H2CO3 molecule decomposes in the presence of another H2CO3 molecule when the vapor phase concentration of H2CO3 reaches its highest level. However, this autocatalytic decomposition mechanism is not expected to be the primary decomposition mechanism in the Earth's atmosphere or in the surroundings away from the source points of H2CO3. This follows as the probability of bimolecular encounters between the two H2CO3 molecules is expected to fall off significantly due to dilution of the carbonic acid concentration resulting from the presence of other various species detected in the Earth's atmosphere or in the surroundings away from the source points of H2CO3. It is also worthwhile to note here that the results of recent experiments from Loerting et al.33 suggest undeniably that in our atmosphere some solid H2CO3 may be present in cirrus clouds or on mineral dust, as in the middle or upper troposphere where the reaction between mineral dust particles containing CaCO3 and acids like HCl can produce solid H2CO3, which remains intact at cold temperatures (210–260 K) and under high relative humidity.6,33 Also, as the wide range of temperature found in the troposphere matches with the range of temperature at which this solid H2CO3 sublimes, the gaseous H2CO3 molecules may indeed be present in the middle or upper troposphere via the sublimations of solid H2CO3.6,33 Moreover, H2CO3 may also form in the upper troposphere by the reaction of CO2 and H2O in a water cluster.21
Therefore, in view of the fact that the detection of gas-phase H2CO3 in the Earth's troposphere and in outer space is very challenging,2,5,6,22 it is important to investigate the stability of the H2CO3 molecule in the presence of other various species detected in the Earth's atmosphere and outer space. It is also important to note here that the decomposition of the H2CO3 molecule into its constituents CO2 and H2O molecules in the presence of one to three water molecules has been studied previously,39–41 but not in the scenario of potential atmospheric relevance. Very recently, the carboxylic acid (RCO2H)-assisted decomposition of the H2CO3 molecule into its constituents CO2 and H2O molecules has been studied by Kumar et al.,42,43 and the primary focus of this work was to explore the instability of the gaseous H2CO3 molecule in the presence of carboxylic acids in comparison to the water monomer-assisted H2CO3 decomposition, especially at the 0 km altitude of the Earth's atmosphere. However, as the gaseous H2CO3 molecule is also believed to be present in cirrus clouds of the Earth's atmosphere or in the middle and/or upper troposphere of the Earth's atmosphere, it is also important to study the stability of the gaseous H2CO3 molecule with respect to the concentrations of water and carboxylic acids present not only in the middle and/or upper troposphere but also in the lower stratosphere. It should be noted here that the cirrus clouds in the Earth's atmosphere are typically observed in the upper troposphere and in the lower stratosphere.44,45
Moreover, the shortest route for the gas-phase decomposition of the isolated H2CO3 molecule into its constituents CO2 and H2O molecules is the concerted mechanism that involves dehydroxylation of one particular OH functional group and a simultaneous dehydrogenation of other OH functional group among the two nonequivalent OH functional groups present in the cis–trans conformer of the H2CO3 molecule (Fig. 1A).39–41 Note here that the cis–trans conformer of H2CO3, as shown in Fig. 1A, is the second most stable conformer of carbonic acid, and it is the starting point for the decomposition of the H2CO3 molecule into its constituents CO2 and H2O molecules (see below).38–41 In addition, to visualize how the water (H2O), formic acid (HC(O)OH
FA) and H2CO3 molecule itself, because of their simultaneous hydrogen donor and acceptor capabilities promote this hydrogen transfer process, we also present the shortest routes for the water-, FA- and H2CO3-assisted decompositions of the H2CO3 molecule in the same figure (Fig. 1B to D).38–40,42 It is seen from the literature that the sulfuric acid (H2SO4
SA) and hydroperoxide (HO2) radical, which have also been detected in the Earth's atmosphere, are also effective catalysts like formic acid, especially for the hydrogen transfer reactions via the O–H bond breaking and making processes within the doubly or multiply hydrogen-bonded interfaces.46–48 Therefore, it is also important to investigate the energetics and kinetics of the SA- and HO2 radical-assisted H2CO3 decomposition reactions of potential atmospheric relevance, especially with respect to the concentrations of these two species in the Earth's troposphere and lower stratosphere where the carbonic acid is expected to exist. Thus, to understand the stability of the gaseous H2CO3 molecule, especially in the Earth's troposphere and lower stratosphere, here we focus upon the energetics of the potential energy diagrams as well as the simple relative kinetics for the decomposition of the H2CO3 molecule into its constituents CO2 and H2O molecules via the shortest route in the presence of one to three water molecules as well as in the presence of the formic acid (FA), acetic acid (AA), sulfuric acid (SA) and HO2 radical.
 |
| Fig. 1 Visualization of the shortest routes for the decomposition of isolated carbonic acid (A) and decomposition of carbonic acid in the presence of water, formic acid and carbonic acid (B to D) via either intramolecular or intermolecular hydrogen transfer processes. | |
2. Computational methods
The Gaussian-09 suite of program with “opt = tight” convergence criteria has been used to carry out all the quantum chemistry calculations presented here.49 Both the geometry optimizations and frequency calculations of the monomers and complexes have been performed using the second order Møller–Plesset (MP2) perturbation theory in conjunction with aug-cc-pVDZ, aug-cc-pVTZ and 6-311++G(3df,3pd) basis sets. It is worthwhile to note here that the geometry optimizations using the larger basis sets are required to reduce basis set superposition error (BSSE), even though full (100%) counterpoise corrections often underestimate binding energies of dimeric complexes.50–52 Transition states (TS) have been located using the QST2/QST3 and OPT = TS routines as implemented in the Gaussian-09 program. Furthermore, Intrinsic Reaction Coordinate (IRC) calculations were performed at the MP2/aug-cc-pVDZ level of theory to unambiguously verify that the transition states found connect with the desired reactants and products. In order to improve our estimates of the reaction energetics and reaction rate constants for the few selective reactions, we have also carried out single point energy calculations at the CCSD(T)/aug-cc-pVTZ level using the MP2/aug-cc-pVTZ level optimized geometries. Normal mode vibrational frequencies have been used to estimate the zero point vibration energy (ZPE) corrections for the reactants, products and TS. The computed total electronic energies (Etotal), ZPE corrected electronic energies [Etotal(ZPE)] for the monomers and complexes and the transition states are given in ESI Table 1.† In addition, normal mode vibrational frequency analyses have been performed for all the stationary points to verify that the stable minima have all positive vibrational frequencies and that the transition states have only one imaginary frequency (see ESI Table 2†).
3. Results and discussion
It is seen from the high levels of theoretical calculations3,4,31,32,53–55 that the H2CO3 monomer has three conformers based on the different orientations of two OH functional groups present in the molecule, and among these three conformers, the cis–cis [(cc)] and cis–trans [(ct)] conformers, as shown in Fig. 2, are respectively the global minimum and the second most stable one.3,4 Moreover, the (ct)-conformer, which is slightly energetically disfavored over the (cc)-conformer, is the starting point for the decomposition of H2CO3 into its constituents CO2 and H2O molecules,38–43 as the direct decomposition of the isolated (cc)-H2CO3 into its constituents CO2 and H2O molecules is forbidden in the vapor phase. It is worthwhile to note here that though the direct decomposition of the isolated (cc)-H2CO3 into its constituents CO2 and H2O molecules is forbidden in the vapor phase, nevertheless, our recent study55 on the energetics of the double hydrogen transfer in carbonic acid homodimers suggests that the interconversion of the (cc)-conformer to its (ct)-conformer [(cc)-H2CO3 → (ct)-H2CO3] is also autocatalytic and potentially allowed, in particular, during the sublimation of pure H2CO3 polymorphs at cold temperatures. Therefore, taking note that the (ct)-conformer of H2CO3 is the starting point for the decomposition of H2CO3 into its constituents CO2 and H2O molecules [(ct)-H2CO3 → CO2 + H2O
H2CO3 → CO2 + H2O], we first investigate the energetics of potential energy diagrams for the H2CO3 decomposition in the presence of one to three water molecules with various mechanistic pathways of potential atmospheric relevance. Second, to compare the energetics of all these water-assisted H2CO3 → CO2 + H2O decompositions reactions, we investigate the energetics of the potential energy diagrams for the H2CO3 decomposition in the presence of FA, AA, SA and HO2 radical, which have been detected or are present in the Earth's atmosphere at significant trace levels.56–75
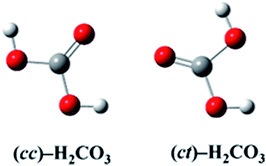 |
| Fig. 2 MP2/aug-cc-pVTZ level optimized geometries of the two most stable conformers of carbonic acid (H2CO3). | |
3A. H2CO3 decomposition in the presence of one to three water molecules
In Fig. 3, we present the MP2/aug-cc-pVTZ level calculated potential energy diagram for the direct decomposition of the isolated H2CO3 molecule into its constituents CO2 and H2O molecules [H2CO3 → CO2 + H2O]. The MP2/aug-cc-pVTZ level optimized geometries of the H2CO3 reactant, CO2⋯H2O product complex and transition state (TS) have also been shown in the potential energy diagram. It is worthwhile to note here that the H2CO3 reactant, which is shown in Fig. 3, is the (ct)-conformer of H2CO3, and also, the (ct)-conformer of H2CO3 is the starting point for the decomposition of the isolated H2CO3 molecule into its constituents CO2 and H2O molecules. From Fig. 3 and as noted above, the H2CO3 → CO2 + H2O decomposition occurs via the transfer of a hydrogen atom from a particular OH functional group to the oxygen atom of the other nonequivalent OH functional group present within the H2CO3 reactant (Fig. 1A). Note that the two OH functional groups present in the H2CO3 reactant are nonequivalent as their orientations are different with respect to the orientation of the C
O functional group present within the H2CO3 reactant. For comparison, in the same figure, we also present the potential energy diagram for the water monomer (H2O)-assisted H2CO3 → CO2 + H2O decomposition reaction. The MP2/aug-cc-pVTZ level optimized geometries of the entrance channel pre-reactive H2CO3⋯H2O complex (RC-I), exit channel CO2⋯H2O⋯H2O product complex (PC-I) and the transition state (TS-I) associated with the H2O-assisted H2CO3 decomposition reaction are also shown in Fig. 3. As expected, the water molecule, because of its simultaneous hydrogen donor and acceptor capability, promotes the above mentioned hydrogen transfer process (Fig. 1A). At the MP2/aug-cc-pVTZ level of calculations, it is seen that the effective barrier, defined as the difference between the zero point vibrational energy (ZPE) corrected energy of the transition state and the total energy of the isolated starting reactants in terms of bimolecular encounters, in the case of the H2O-assisted decomposition reaction is ∼13.5 kcal mol−1, and this value is approximately three times lower than the barrier height associated with the naked H2CO3 → CO2 + H2O decomposition reaction. The predicted barrier height for the naked H2CO3 → CO2 + H2O unimolecular decomposition reaction at the MP2/aug-cc-pVTZ level including ZPE correction is ∼36.8 kcal mol−1. The calculated binding energies of the various complexes as well as the barrier heights for the rate limiting unimolecular decomposition steps, as discussed in this article, are given in Tables 1 and 2. From Tables 1 and 2, it is seen that the predicted binding energies as well as barrier heights at the MP2 level of calculations are consistent for the three different basis sets used.
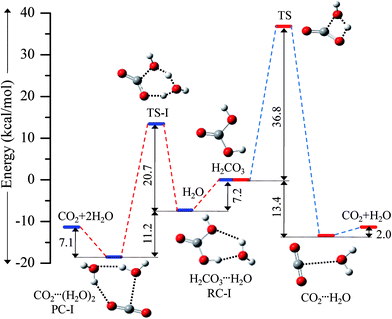 |
| Fig. 3 Potential energy profiles for the decomposition of the isolated H2CO3 molecule (red color) and for water monomer-assisted H2CO3 decomposition (blue color). The energy profiles have been calculated at the MP2/aug-cc-pVTZ level of theory including zero point vibration energy (ZPE) corrections. | |
Table 1 Zero point vibrational energy (ZPE) corrected binding energies (kcal mol−1) of all the complexes at the MP2 level of theory in conjunction with aug-cc-pVDZ, aug-cc-pVTZ and 6-311++G(3df,3pd) basis sets as well as at the CCSD(T)/aug-cc-pVTZ level of calculations. The binding energies of all the pre-reactive complexes (RC-I to RC-XIII) have been calculated in terms of bimolecular encounters between two reactants (monomers/complexes) as given in the 1st column of the table. As an example, in the case of RC-III, the binding energy has been calculated by subtracting the total electronic energies of the RC-I + H2O reactants from the calculated energy of the RC-III. In the case of exit channel product complexes (PC-I to PC-XIII), other than those labeled below, the binding energies have been calculated by subtracting the total electronic energies of all the monomers forming the complex from the calculated energy of that complex. Normal mode vibrational frequency calculations at the MP2/aug-cc-pVDZ, MP2/aug-cc-pVTZ and MP2/6-311++G(3df,3pd) levels have been performed to estimate the respective zero point energy (ZPE) corrections. ZPE correction in case of the CCSD(T)/aug-cc-pVTZ level of calculations has been done from the MP2/aug-cc-pVTZ level-predicted ZPE correction
Complexes |
MP2/aug-cc-pVDZ |
MP2/aug-cc-pVTZ |
MP2/6-311++G(3df,3pd) |
CCSD(T)/aug-cc-pVTZ |
Binding energy of the CO2⋯(H2O)3 complex when it is formed from bimolecular encounters between the CO2 + (H2O)3 reactants. Binding energy of the CO2⋯(H2O)4 complex when it is formed from bimolecular encounters between the CO2 + (H2O)4 reactants. Binding energy of the CO2⋯(H2O)4 complex when it is formed from ternary encounters between the CO2 + (H2O)3 + H2O reactants. |
RC-I (H2CO3 + H2O) |
7.33 |
7.25 |
7.23 |
7.22 |
RC-II [(H2CO3 + (H2O)2] |
11.36 |
11.33 |
11.26 |
11.16 |
RC-II (RC-I + H2O) |
7.18 |
7.16 |
7.12 |
7.06 |
RC-III (RC-I + H2O) |
9.64 |
9.68 |
9.64 |
— |
RC-IV (RC-I + H2O) |
6.56 |
6.42 |
6.40 |
— |
RC-V (RC-II + H2O) |
10.12 |
10.13 |
10.11 |
— |
RC-VI [(RC-I + (H2O)2] |
16.25 |
16.32 |
16.36 |
— |
RC-VII [(RC-I + (H2O)2] |
13.36 |
13.13 |
13.16 |
— |
RC-VIII [(RC-I + (H2O)2] |
13.35 |
13.20 |
13.27 |
— |
RC-IX [(H2CO3 + (H2O)3] |
8.74 |
8.58 |
8.55 |
— |
RC-X (H2CO3 + FA) |
10.86 |
11.01 |
10.87 |
11.01 |
RC-XI (H2CO3 + AA) |
11.90 |
12.04 |
11.87 |
12.08 |
RC-XII (H2CO3 + SA) |
11.09 |
11.50 |
11.69 |
11.54 |
RC-XIII (H2CO3 + HO2) |
8.93 |
8.91 |
9.01 |
8.66 |
PC-I |
7.38 |
7.15 |
7.11 |
7.44 |
PC-II |
14.31 (3.29)a |
13.97 (3.11)a |
14.00 (3.11)a |
14.23 |
PC-III |
11.23 |
10.84 |
10.78 |
— |
PC-IV |
14.18 (3.16)a |
13.86 (3.00)a |
13.87 (2.99)a |
— |
PC-V |
19.44 (8.42)c |
18.96 (8.10)c |
18.97 (8.08)c |
— |
PC-VI |
19.44 |
18.96 |
18.97 |
— |
PC-VII |
24.28 (3.79)b |
23.74 (3.44)b |
23.89 (3.43)b |
— |
PC-VIII |
23.78 (3.28)b |
23.37 (3.07)b |
23.50 (3.04)b |
— |
PC-IX |
19.15 (8.13)c |
18.69 (7.84)c |
18.68 (7.79)c |
— |
PC-X |
9.92 |
9.80 |
9.71 |
10.21 |
PC-XI |
10.37 |
10.21 |
10.08 |
10.66 |
PC-XII |
13.84 |
11.01 |
11.18 |
11.48 |
PC-XIII |
9.29 |
8.98 |
9.09 |
9.03 |
Table 2 Zero point vibrational energy (ZPE) corrected barrier heights (kcal mol−1) for various unimolecular decomposition steps associated with the reaction pathways from R-I to R-XIII, as labeled in the 1st column of table, at the MP2/aug-cc-pVDZ, MP2/aug-cc-pVTZ and MP2/6-311++G(3df,3pd) levels of calculations as well as at the CCSD(T)/aug-cc-pVTZ level of calculations. The values in parentheses are the relative energies of the transition states (TS-I to TS-XIII) with respect to the reactants that are involved in bimolecular encounters as discussed in the text. For example, in the case of the reaction pathway R-I, the MP2/aug-cc-pVTZ level of calculation incorporating ZPE correction predicts that the TS-I is 13.50 kcal mol−1 higher in energy (indicated by positive sign) than the total energy of the H2CO3 + H2O reactants. Normal mode vibrational frequency calculations at the MP2/aug-cc-pVDZ, MP2/aug-cc-pVTZ and MP2/6-311++G(3df,3pd) levels have been performed to estimate the respective zero point energy (ZPE) corrections. The ZPE corrections in case of the CCSD(T)/aug-cc-pVTZ level of calculations have been done from the MP2/aug-cc-pVTZ level-predicted ZPE corrections
Transition states |
MP2/aug-cc-pVDZ |
MP2/aug-cc-pVTZ |
MP2/6-311++G(3df,3pd) |
CCSD(T)/aug-cc-pVTZ |
TS-I [R-I H2CO3 + H2O] |
20.93 (+13.60) |
20.69 (+13.50) |
21.25 (+14.02) |
23.36 (+16.14) |
TS-II [R-II H2CO3 + (H2O)2] |
17.52 (+6.16) |
16.97 (+5.64) |
17.25 (+5.99) |
19.55 (+8.39) |
TS-II [R-IIa RC-I + H2O] |
17.52 (+10.34) |
16.97 (+9.82) |
17.25 (+10.13) |
— |
TS-III [R-III RC-I + H2O] |
20.38 (+10.74) |
19.98 (+10.30) |
20.48 (+10.83) |
— |
TS-IV [R-IV RC-I + H2O] |
19.53 (+12.97) |
19.61 (+13.19) |
20.14 (+13.74) |
— |
TS-V [R-V RC-II + H2O] |
17.13 (+7.02) |
16.39 (+6.26) |
16.64 (+6.53) |
— |
TS-VI [R-VI RC-I + (H2O)2] |
19.89 (+3.64) |
19.34 (+3.02) |
19.77 (+3.41) |
— |
TS-VII [R-VII RC-I + (H2O)2] |
16.52 (+3.17) |
16.53 (+3.40) |
17.09 (+3.93) |
— |
TS-VIII [R-VIII RC-I + (H2O)2] |
16.83 (+3.48) |
16.84 (+3.64) |
17.37 (+4.10) |
— |
TS-IX [R-IX H2CO3 + (H2O)3] |
19.24 (+10.49) |
19.23 (+10.65) |
19.71 (+11.16) |
— |
TS-X [R-X H2CO3 + FA] |
12.46 (+1.60) |
12.24 (+1.23) |
12.47 (+1.60) |
14.37 (+3.36) |
TS-XI [R-XI H2CO3 + AA] |
11.98 (+0.08) |
11.89 (−0.15) |
12.05 (+0.18) |
13.92 (+1.84) |
TS-XII [R-XII H2CO3 + SA] |
12.05 (+0.96) |
11.56 (+0.06) |
11.75 (+0.06) |
13.85 (+2.31) |
TS-XIII [R-XIII H2CO3 + HO2] |
9.36 (+0.43) |
9.58 (+0.67) |
9.78 (+0.77) |
15.56 (+6.9) |
The H2CO3 → CO2 + H2O decomposition reaction in the presence of two water molecules has been considered to occur mainly in two different reaction pathways. First is the reaction pathway where the water dimer [(H2O)2] assists actively to facilitate the above mentioned hydrogen transfer process required for the decomposition of H2CO3. It is worthwhile to note here that the (H2O)2 is present in the Earth's atmosphere at significant trace levels.47,76 Second is the reaction pathway where the second water molecule among the two water molecules stabilizes either actively or passively the pre-reactive H2CO3⋯H2O complex (RC-I) and/or the TS-I, which have been identified for the H2O-assisted H2CO3 decomposition reaction. It is to be noted here that this second pathway, where the second water molecule among the two water molecules participates in the H2CO3 decomposition either actively or passively, is based on the role of the second water molecule if it assists either actively or passively the intermolecular hydrogen transfer process required for the H2O-assisted H2CO3 decomposition, as described earlier (Fig. 1B). Through these two main pathways, a total of three optimized geometries for the entrance channel H2CO3⋯H2O⋯H2O pre-reactive complexes (RC-II, III and IV), as shown in Fig. 4, were identified. In Fig. 4, we have also shown the optimized geometries of the corresponding transition states (TS-II, III and IV) and the exit channel CO2⋯H2O⋯H2O⋯H2O product complexes (PC-II, III and IV) associated with these three different H2CO3⋯H2O⋯H2O pre-reactive complexes (RC-II, III and IV). The MP2/aug-cc-pVTZ level calculated potential energy diagrams associated with these three different pre-reactive complexes (RC-II, III and IV), including their respective ZPE corrections, are shown in Fig. 5. Note here that the RC-II is a common pre-reactive complex in the case of pathways where the (H2O)2 participates in the H2CO3 decomposition reaction actively and where the second water molecule among the two water molecules stabilizes actively the pre-reactive H2CO3⋯H2O complex (RC-I) identified for the H2O-assisted decomposition reaction. Indeed, from our relative rate analysis (see below), we show that both channels are of near equal probabilities to occur in the Earth's atmosphere, and hence, a correction-factor ∼2 can roughly be taken into account for the (H2O)2-assisted H2CO3 decomposition reaction to emphasize the resultant impact of these two reaction channels together. Moreover, it is also worthwhile to note here that the H2CO3⋯H2O⋯H2O pre-reactive complex can also be formed through ternary collisions involving H2CO3 and two H2O molecules. Nevertheless, the probability of this three-body collision occurring is expected to be relatively low in the Earth's atmosphere. From Fig. 5, it is seen that the most dominant channel according to the lowest and effective potential energy barrier height, as defined above, is the pathway where the H2CO3 molecule hits the water dimer [(H2O)2] directly and when the (H2O)2 assists the hydrogen transfer process actively. At the MP2/aug-cc-pVTZ level of calculation including ZPE corrections, the effective barrier height for this (H2O)2-assisted decomposition pathway is only ∼5.6 kcal mol−1. This value is more than two times lower than the barrier height associated with the H2O-assisted H2CO3 decomposition pathway. As mentioned above, the MP2/aug-cc-pVTZ level predicted effective barrier height for the H2O-assisted H2CO3 decomposition reaction is ∼13.5 kcal mol−1. Moreover, the comparison of the potential energy profiles for the H2O- and (H2O)2-assisted decomposition reactions suggest that the successive addition of water molecules in the decomposition mechanism is not counterproductive when only the energetics of the potential energy diagrams are considered. Therefore, and next, we focus upon the H2CO3 decomposition in the presence of three water molecules with a variety of possible bimolecular encounters that are expected to be relevant in the Earth's atmosphere.
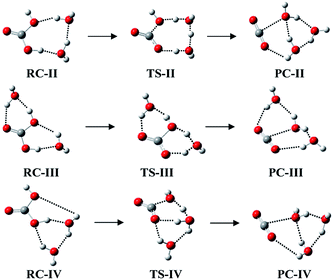 |
| Fig. 4 MP2/aug-cc-pVTZ level optimized geometries of the three different entrance channel pre-reactive H2CO3⋯H2O⋯H2O complexes (RC-II, III and IV) and their corresponding three exit channel CO2⋯H2O⋯H2O⋯H2O product complexes (PC-II, III and IV) and transition states (TS-II, III and IV) associated with the two water molecule-assisted H2CO3 → CO2 + H2O decomposition reaction. | |
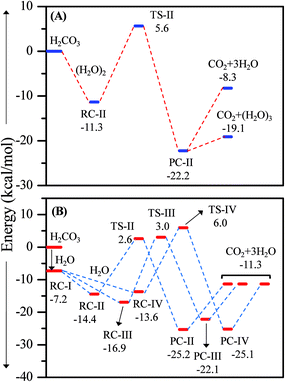 |
| Fig. 5 MP2/aug-cc-pVTZ level predicted and ZPE corrected four different potential energy profiles for the H2CO3 → CO2 + H2O decomposition reaction in the presence of two water molecules that are associated with the RC-II, III and IV. The potential energy profile associated with the H2CO3 + H2O⋯H2O reactant channel is shown in (A), and the three different potential energy profiles for the H2CO3⋯H2O + H2O reactants are shown in (B). | |
The H2CO3 → CO2 + H2O decomposition reaction in the presence of three water molecules can be considered as the participation of the third water molecule in all the reaction pathways which have been considered above in the case of the two water molecule-assisted H2CO3 → CO2 + H2O decomposition reactions. In other words, and like before, these are the reaction pathways in which the third water molecule among the three water molecules stabilizes either actively or passively the pre-reactive H2CO3⋯H2O⋯H2O complexes (RC-II, III and IV) or the transition states (TS-II, III and IV) obtained above for the two water molecule-assisted H2CO3 decomposition reactions. Moreover, the water trimer [(H2O)3], which is also expected to be present in the Earth's atmosphere at significant trace levels,76 can assist the H2CO3 → CO2 + H2O decomposition with the pathways in which either only one or two water subunits among the three water subunits present in the water trimer [(H2O)3] participate actively. It is worthwhile to note here that unlike the optimized structure of the water dimer [(H2O)2], which is the single hydrogen-bonded (H-bonded) open complex, the water trimer [(H2O)3] is the triple H-bonded cyclic complex.76 Therefore, and overall, we note that there are numerous bimolecular encounters with respect to the H2CO3 + (H2O)3, H2CO3⋯H2O + (H2O)2 and H2CO3⋯H2O⋯H2O + H2O reactant channels, which may result in the decomposition of the H2CO3 molecule into its constituents CO2 and H2O molecules. Having rationalized all these numerous bimolecular encounters, we identify various geometries of the H2CO3⋯H2O⋯H2O⋯H2O pre-reactive complexes. However, subsequent identification of the transition states and IRC calculations suggest that many of them are irrelevant, as the IRC calculations of the transition states do not correspond with many of the desired pre-reactive and exit-channel product complexes in terms of the shortest decomposition route as we have discussed above. Finally, in Fig. 6, we present five different pre-reactive H2CO3⋯H2O⋯H2O⋯H2O complexes (RC-V, VI, VII, VIII and IX) and their corresponding five different transition states (TS-V, VI, VII, VIII and IX) as well as their five exit channel CO2⋯H2O⋯H2O⋯H2O⋯H2O product complexes (PC-V, VI, VII, VIII and IX), which are connected via the IRC calculations. In Fig. 7, we present five different potential energy diagrams associated with the five different pre-reactive H2CO3⋯H2O⋯H2O⋯H2O complexes (RC-V, VI, VII, VIII and IX). From Fig. 7, we first point out that for the bimolecular encounters between H2CO3 and (H2O)3 reactants with the pathway in which only one water subunit among the three water subunits present in the water trimer [(H2O)3] participate actively or for the decomposition pathway associated with the RC-IX, the effective barrier is 10.7 kcal mol−1. This value is approximately two times higher than the effective barrier for the (H2O)2-assisted H2CO3 decomposition pathway. As mentioned above, the effective barrier height for the (H2O)2-assisted H2CO3 decomposition pathway is ∼5.6 kcal mol−1. Therefore, as the atmospheric concentration of (H2O)3 is substantially lower than the concentration of (H2O)2,76 we find that this channel is not important with respect to the main focus of this article, and hence, we ignore this channel in further discussions from here onwards. Second, the most effective pathways according to the lowest and effective potential energy barriers are the bimolecular encounters between H2CO3⋯H2O and (H2O)2 that result in the RC-VI, VII and VIII. This is because for these three pathways, the MP2/aug-cc-pVTZ level predicted effective barrier heights including ZPE correction, as defined above, are only ∼3, 3.4 and ∼3.6 kcal mol−1, respectively. These values are on an average ∼1.7 times lower than the barrier height associated with the (H2O)2-assisted H2CO3 decomposition pathway, as mentioned above. Therefore, and particularly, these three channels in the case of three water molecule-assisted H2CO3 decompositions are expected to have similar impacts to each other and also, expected to be more important in comparison to all the pathways which have been considered above for the water monomer- and two water molecule-assisted H2CO3 decompositions. However, below we show from the relative reaction rate analysis with atmospheric concentrations of the H2O monomer and dimer that among these three channels, the two channels, which are associated with the RC-VII and VIII, are actually less important in comparison to the H2O-, (H2O)2-, formic acid (FA)- and acetic acid (AA)-assisted H2CO3 → CO2 + H2O decomposition reactions.
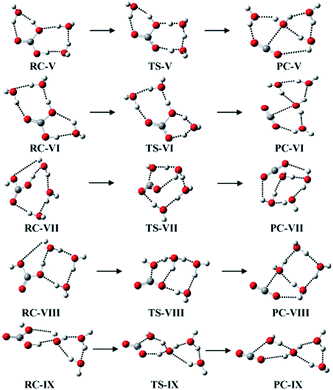 |
| Fig. 6 MP2/aug-cc-pVTZ level optimized geometries of the five different entrance channel pre-reactive H2CO3⋯H2O⋯H2O⋯H2O complexes (RC-V, VI, VII, VIII and IX) and their corresponding five exit channel CO2⋯H2O⋯H2O⋯H2O⋯H2O product complexes (PC-V, VI, VII, VIII and IX) and transition states (TS-V, VI, VII, VIII and IX) associated with the three water molecule-assisted H2CO3 → CO2 + H2O decomposition reaction. | |
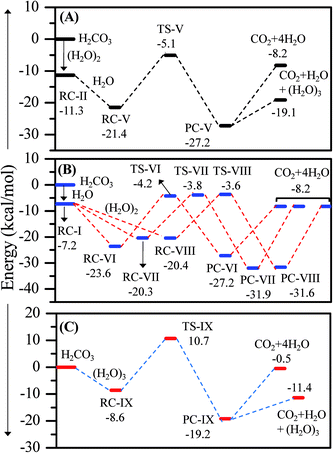 |
| Fig. 7 MP2/aug-cc-pVTZ level predicted and ZPE corrected five different potential energy profiles for the H2CO3 → CO2 + H2O decomposition reaction in the presence of three water molecules that are associated with the RC-V, VI, VII, VIII and IX. (A) Potential energy profile for the H2CO3⋯H2O⋯H2O + H2O reactants that results in the RC-V. (B) Three different potential energy profiles for the H2CO3⋯H2O + H2O⋯H2O reactants that result in RC-VI, VII, VIII. (C) Potential energy profile for the H2CO3 + (H2O)3 reactants that results in the RC-IX. | |
3B. H2CO3 decomposition in the presence of FA, AA, SA and HO2 radical
In this section, we explore the reaction energetics for the H2CO3 decomposition in the presence of the formic acid (FA), acetic acid (AA), sulfuric acid (SA) and hydroperoxy (HO2) radical. These species are not only present at significant trace levels in the Earth's atmosphere56–75 but also known as effective catalysts for hydrogen transfer reactions at the doubly or multiply hydrogen-bonded interfaces via the O–H bond breaking and making processes.46–48,77–83 Like before, for the FA-, AA-, SA- and HO2 radical-assisted H2CO3 decomposition reactions, the MP2/aug-cc-pVTZ level optimized geometries of transition states (TS-X, XI, XII and XIII), their IRC verified pre-reactive complexes (RC-X, XI, XII and XIII) and the exit channel product complexes (PC-X, XI, XII and XIII) are shown in Fig. 8. In Fig. 9, we present the MP2/aug-cc-pVTZ level calculated potential energy profiles for these FA-, AA-, SA- and HO2 radical-assisted H2CO3 → CO2 + H2O decomposition reactions. From Fig. 9, it is seen that the effective barriers, as defined before, for these FA-, AA-, SA- and HO2-assisted H2CO3 decomposition reactions are negligible or very small, with the transition states being at either slightly higher or lower energies with respect to the total energies of the isolated starting bimolecular reactants. Therefore, the catalytic efficiencies of FA, AA, SA and HO2 radical upon the H2CO3 → CO2 + H2O decomposition reaction are more or less equal to each other, especially at the MP2 level of theory. Moreover, the effective barriers for these decomposition reactions are similar to those we find in the case of the two autocatalytic H2CO3 → CO2 + H2O decomposition reactions as reported by us very recently with respect to the two most stable conformers of carbonic acid.38 Note that at the MP2/aug-cc-pVTZ level of calculations, the effective barriers for the two autocatalytic H2CO3 decomposition reactions are respectively ∼0.2 and ∼1.7 kcal mol−1 with the transition states being at lower energies.38 Therefore, the above results and especially the energetics of the potential energy diagrams for the FA- and AA-assisted H2CO3 decompositions are the expected ones, as these two carboxylic acids promote the H2CO3 decomposition by their hydrogen donor (O–H) and hydrogen acceptor (C
O) functional groups in a similar way to how carbonic acid does in the case of its autocatalytic decomposition (Fig. 1). Note here that the primary mechanism for the decomposition of the H2CO3 molecule into its constituents CO2 and H2O molecules, especially at its source where the vapor phase concentration of the H2CO3 molecule reaches its highest level, is autocatalytic,38 and this is based upon the experimental observation that the sublimation of pure H2CO3 polymorphs results in the decomposition of the H2CO3 molecule into its constituents CO2 and H2O in the water-restricted environment.5,6 Further detailed analysis of the potential energy profiles, as presented in Fig. 9, suggests that the most effective catalysts are the AA and SA when only the effective barriers of the potential energy profiles are considered. However, below we specifically show that the SA-assisted H2CO3 → CO2 + H2O decomposition reaction has no significant role in comparison to what we find in the cases of FA- and AA-assisted H2CO3 → CO2 + H2O decomposition reactions, especially in the Earth's troposphere and lower stratosphere. Thus, and finally, given that the FA-, AA-, SA- and HO2 radical-assisted H2CO3 decompositions are effectively near-barrierless reactions and the catalytic efficiencies of FA, AA, SA and HO2 radical upon the H2CO3 decomposition reaction are more or less equal to each other, we next explore the potential roles of all these pathways towards an understanding of the atmospheric instability of the gas-phase H2CO3 molecule up to a 15 km altitude from the Earth's surface. It is to be noted here that the carbonic acid is expected to be present in the Earth's troposphere and lower stratosphere.
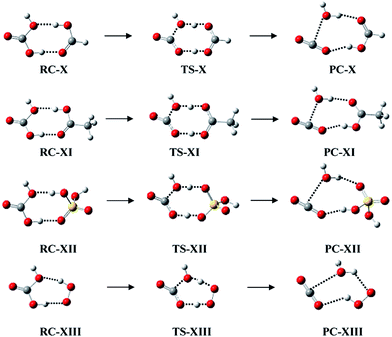 |
| Fig. 8 MP2/aug-cc-pVTZ level optimized geometries of the entrance channel pre-reactive complexes (RC-X, XI, XII and XIII), exit channel product complexes (PC-X, XI, XII and XIII) and transition states (TS-X, XI, XII and XIII) associated with formic acid (FA)-, acetic acid (AA)-, sulfuric acid (SA)- and hydroperoxide (HO2) radical-assisted H2CO3 → CO2 + H2O decomposition reactions. | |
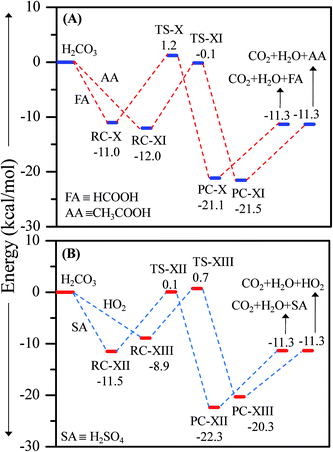 |
| Fig. 9 (A) Potential energy profiles for formic acid (FA)- and acetic acid (AA)-assisted H2CO3 → CO2 + H2O decomposition reactions. (B) Potential energy profiles for the sulfuric acid (SA)- and hydroperoxide (HO2) radical-assisted H2CO3 → CO2 + H2O decomposition reactions. The energy profiles have been calculated at the MP2/aug-cc-pVTZ level of theory including zero point vibration energy (ZPE) corrections. | |
3C. Relative rates and atmospheric implications of the above results
It has been mentioned earlier that the carbonic acid has not been detected yet in the Earth's atmosphere or in outer space either in the solid or gas phase. Therefore, as the concentration of carbonic acid is not known in the Earth's atmosphere, we point out that the relative rate analysis is only the method by which one can explore the relative impacts of the various species upon the H2CO3 → CO2 + H2O decomposition reaction in the Earth's atmosphere. It is worthwhile to note that in order to assess the impacts of various pathways discussed above, it is necessary to compare reaction rates rather than reaction rate constants. This follows as the various catalytic species may have similar catalytic efficiencies upon a reaction of potential atmospheric relevance, but they may not have similar concentrations in the Earth's atmosphere. We also point out here that it is not the goal of the present work to calculate absolute rates but to only examine relative rates associated with reagent energetics computed at the same level of theory. The rate constants for various pathways, as discussed here, have been calculated by the conventional transition state theory (TST) including tunneling correction according to the unsymmetrical Eckart potential barriers.84–91 The calculated rate constants for various pathways and the atmospheric concentrations of the water monomer, dimer, FA, AA, SA and HO2 radical have been tabulated in the ESI.† Also, the computational methodology and equations used to estimate the reaction rates of various pathways with respect to rate of the water monomer (H2O)-assisted H2CO3 → CO2 + H2O decomposition reaction are given in detail in the ESI.† Here we only provide and focus upon the final results of relative rates for all the decomposition pathways by taking the H2O-assisted H2CO3 decomposition reaction as the reference. The relative rates for all the decomposition pathways are given in Tables 3 and 4. In Tables 3 and 4, the reaction rates (νI to νVIII) for the various reaction pathways have been labeled according to the various pre-reactive complexes (RC). For example, the H2CO3 decomposition reaction associated with the ‘RC-I’ corresponds to the reaction path ‘R-I’ and the reaction rate ‘νI’. Similarly, the H2CO3 decomposition reaction associated with the RC-XIII corresponds to the reaction path ‘R-XIII’ and reaction rate ‘νXIII’.
Table 3 MP2/aug-cc-pVTZ level predicted reaction rates for the various reaction pathways in the presence of one to three water molecules with respect to the rate estimated for the H2O-assisted H2CO3 decomposition reaction (νI). The reaction rates (νI to νVIII) for the various reaction pathways have been labeled according to the various pre-reactive complexes (RC-I to RC-VIII), as mentioned in the text. The values given in parentheses correspond to the results predicted at the CCSD(T)/aug-cc-pVTZ level of calculations. Temperatures at different altitudes of the Earth's atmosphere have been taken from ref. 92
Altitude (km) |
T (K) |
(νI/νI) |
(νII/νI)a |
(νIIa/νI)a |
(νIII/νI) |
(νIV/ν1) |
(νV/νI) |
(νVI/νI) |
(νVII/νI) |
(νVIII/νI) |
νII and νIIa correspond to the rates of the reaction pathways in which the water dimer and two water molecules actively assist the H2CO3 decomposition by a common pre-reactive complex (RC-II). |
0 |
298.15 |
1.0 (1.0) |
5.0 × 10−1 (4.4 × 10−1) |
3.9 × 10−1 |
2.5 × 10−1 |
3.2 × 10−3 |
3.7 × 10−1 |
1.7 × 10−1 |
4.4 × 10−2 |
3.8 × 10−2 |
5 |
259.3 |
1.0 (1.0) |
2.3 × 10−1 (1.9 × 10−1) |
1.8 × 10−1 |
1.5 × 10−1 |
5.9 × 10−4 |
1.2 × 10−1 |
0.5 × 10−1 |
0.9 × 10−2 |
0.7 × 10−2 |
10 |
229.7 |
1.0 (1.0) |
3.5 × 10−2 (2.7 × 10−2) |
2.8 × 10−2 |
3.4 × 10−2 |
3.8 × 10−5 |
5.5 × 10−3 |
2.6 × 10−3 |
2.9 × 10−4 |
2.2 × 10−4 |
15 |
212.6 |
1.0 (1.0) |
5.0 × 10−3 (0.3 × 10−2) |
4.1 × 10−3 |
7.2 × 10−3 |
3.1 × 10−6 |
2.2 × 10−4 |
1.0 × 10−4 |
8.5 × 10−6 |
0.6 × 10−5 |
Table 4 MP2/aug-cc-pVTZ level predicted reaction rates for the various reaction pathways in the presence of H2O, formic acid (FA), acetic acid (AA), sulfuric acid (SA) and hydroperoxide (HO2) radical with respect to the rate estimated for the H2O-assisted H2CO3 decomposition reaction (νI). The reaction rates (νX to νXIII) for the various reaction pathways have been labeled according to the various pre-reactive complexes (RC-X to RC-XIII), and νX, νXI, νXII and νXIII are the rates for the FA-, AA-, SA- and HO2 radical-assisted H2CO3 decomposition reactions, respectively. The values given in parentheses correspond to the results predicted at the CCSD(T)/aug-cc-pVTZ level of calculations. Temperatures at different altitudes of the Earth's atmosphere have been taken from ref. 92
Altitude (km) |
T (K) |
(νI/νI) |
(νX/νI) |
(νXI/νI) |
(νXII/νI) |
(νXIII/νI) |
To estimate the relative reaction rate, the concentration of sulfuric acid at the Earth's surface has been taken ∼3.7 × 108 molecules per cm3, but the average value of the measured concentration is usually expected to be around one order of magnitude less (see ESI Table 9). |
0 |
298.15 |
1.0 (1.0) |
1.5 (4.8) |
11.2 (60.6) |
0.03 (0.2)a |
0.015 (8.6 × 10−5) |
5 |
259.3 |
1.0 (1.0) |
8.4 × 101 (3.4 × 102) |
7.4 × 102 (5.4 × 103) |
1.4 (9.4) |
1.6 (4.9 × 10−3) |
10 |
229.7 |
1.0 (1.0) |
1.4 × 104 (6.9 × 104) |
1.4 × 105 (1.3 × 106) |
7.7 × 101 (6.7 × 102) |
0.9 × 103 (1.7) |
15 |
212.6 |
1.0 (1.0) |
5.3 × 105 (2.9 × 106) |
5.6 × 106 (5.9 × 107) |
2.2 × 102 (2.2 × 103) |
1.4 × 104 (1.8 × 101) |
In order to keep the discussion simple here, we first discuss only the MP2/aug-cc-pVTZ level predicted relative rates at 0 km altitude in the Earth's environment for all the H2CO3 decomposition pathways that have been considered in the presence of one to three water molecules. From the data presented in Table 3, it is seen that the most predominant pathway is the H2O-assisted H2CO3 decomposition reaction, even though the effective barrier height for this channel is substantially higher than the effective barrier heights associated with all the pathways considered for the two and three water molecule-assisted H2CO3 decomposition reactions. Moreover, the reaction rates for two and three water molecule-assisted decomposition reactions, especially, those associated with the RC-II, III, V and VI, are comparable with the rate calculated for the H2O-assisted H2CO3 decomposition reaction within a factor 10. Therefore, according to the MP2/aug-cc-pVTZ level of calculations, and if we consider the H2CO3 → CO2 + H2O decomposition reaction in the presence of water molecules only, it is though that the H2O-assisted H2CO3 decomposition is the most predominant channel, nevertheless, the decomposition of H2CO3 in the presence of the two and three water molecules cannot be ignored completely. Indeed, below we show that at the 0 km altitude in a clean environment of the Earth's atmosphere, especially the H2O- and (H2O)2-assisted H2CO3 decomposition are more or less equally important with the FA-assisted H2CO3 decomposition reaction.
It has been mentioned before that we have also carried out single point energy calculations at the CCSD(T)/aug-cc-pVTZ level using the MP2/aug-cc-pVTZ level optimized geometries for a few selective reactions to improve our estimates regarding the reaction energetics as well as the corresponding reaction rate constants. To compare the H2O- and (H2O)2-assisted H2CO3 decompositions with the FA-, AA-, SA- and HO2 radical-assisted H2CO3 decompositions on equal footing, the CCSD(T)/aug-cc-pVTZ level of calculations have been performed for the H2O, (H2O)2-, FA-, AA-, SA- and HO2 radical-assisted H2CO3 decomposition reactions. The CCSD(T)/aug-cc-pVTZ level estimated binding energies for the pre-reactive and exit channel complexes as well as the effective barrier heights associated with the H2O-, (H2O)2-, FA-, AA-, SA- and HO2 radical-assisted decomposition reactions are given in Tables 1 and 2. The CCSD(T)/aug-cc-pVTZ level predicted rate constants for the decompositions of the H2CO3 molecule in the presence of H2O, (H2O)2, FA, AA, SA and HO2 radical are given in the ESI,† and the calculated relative rates at the same level of theory for these reactions with respect to the H2O-assisted H2CO3 decomposition are given in Tables 3 and 4. From the results at the CCSD(T)/aug-cc-pVTZ level of calculations, it is seen that the effective barriers, as defined above, are sensitive to some extent to the level of calculations used, especially and specifically, in the case of the HO2-assisted H2CO3 decomposition reaction. For the HO2-assisted decomposition, it is though that there is a substantial discrepancy between the MP2/aug-cc-pVTZ and CCSD(T)/aug-cc-pVTZ level predicted barrier heights and/or reaction rates, nevertheless, the results predicted at the CCSD(T)/aug-cc-pVTZ level offer a similar conclusion with respect to that we find from the results predicted at the MP2/aug-cc-pVTZ level of theory. In calculations at both levels, we find that the HO2-assisted H2CO3 decomposition does not play any significant role in the Earth's troposphere and lower stratosphere. This follows as the concentration of the HO2 radical is substantially lower than the concentration of FA at the 0 to 15 km altitude of the Earth's atmosphere (see ESI†). The comparison of the CCSD(T)/aug-cc-pVTZ level predicted relative rates for the H2O-, (H2O)2-, FA-, AA-, and SA-assisted H2CO3 decomposition reactions, as presented in Tables 3 and 4, suggest that the AA-assisted decomposition reaction is the predominant channel at 0 km altitude and the rate for the AA-assisted decomposition reaction is respectively ∼13 and 61 times higher than the reaction rates obtained for the FA- and water monomer-assisted H2CO3 decompositions. It is worthwhile to note here that this prediction is based on the results obtained at the CCSD(T)/aug-cc-pVTZ level of theory. At the MP2/aug-cc-pVTZ level of theory, the rate for the AA-assisted H2CO3 decomposition reaction is respectively ∼7.5 and 11 times higher than the reaction rates obtained for the FA- and H2O-assisted H2CO3 decompositions. Furthermore, as has been pointed out in the ESI,† the average concentration of the FA or AA (8.9 × 1010 molecules per cm3 ≡ 3.56 ppbv) at 0 km altitude, which we have taken into account in our rate calculations, is approximately and at least 4 to 5 times higher than the measured concentrations of FA or AA present in the clean environment of the Earth's atmosphere.57 This follows as the clean and polluted environments of the Earth's atmosphere are defined according to the typical surface mixing ratios74 of FA or AA respectively at less than 1 ppbv and more than 10 ppbv.57 Therefore, in polluted areas, where the mixing ratios of FA and AA reach ∼10 ppbv (∼2.5 × 1011 molecules per cm3) or higher,57 the RCO2H-assisted H2CO3 decomposition is only the dominant channel (RCO2H
carboxylic acids). However, if we consider the measured concentrations of FA and AA in the clean environment57 or when the FA and AA mixing ratios in the rural, urban, semi urban and forest areas,56–58,62–69 are less than 1 ppbv (∼2.5 × 1010 molecules per cm3), the H2O-assisted H2CO3 decomposition has an impact similar to that obtained especially in the case of FA-assisted H2CO3 decomposition reaction. For example, when we consider the CCSD(T)/aug-cc-pVTZ predicted rates and the mixing ratio of ∼0.75 ppbv (∼1.9 × 1010 molecules per cm3) for both FA and AA, the reaction rates for the H2O- and FA-assisted decomposition reactions are similar to each other and the reaction rate for the FA-assisted H2CO3 decomposition is ∼13 times lower than the rate estimated for the AA-assisted decomposition. Similarly, based on the MP2/aug-cc-pVTZ level predicted results, it is seen that the rate for the H2O-assisted decomposition reaction is 3 times higher and only 2.5 times lower, respectively, with respect to the rates estimated for the FA- and AA-assisted decompositions. Furthermore, if the temperature at 0 km altitude is 310 or 320 K instead of 298.15 K and the mixing ratio of FA or AA is ∼3.56 ppbv (which is the average mixing ratio of FA and AA and is equivalent to 8.9 × 1010 molecules per cm3), it is seen that both the H2O- and (H2O)2-assisted H2CO3 decompositions have relatively greater and comparable impacts with both the FA- and AA-assisted decomposition reactions. Note that these are the predictions from the results obtained at the CCSD(T)/aug-cc-pVTZ level of theory. It is also worthwhile to note here that at the MP2/aug-cc-pVTZ level of theory and at 320 K, the rates for the H2O- and AA-assisted H2CO3 decompositions are similar to each other and the rate for the H2O-assisted H2CO3 decomposition is ∼9 times higher than the rate for the FA-assisted decomposition reaction, especially, when we take the concentration of FA or AA to be ∼8.9 x 1010 molecules per cm3 (≡3.56 ppbv). Therefore, and finally, the H2O-assisted H2CO3 → CO2 + H2O decomposition reaction in comparison to the FA- and AA-assisted H2CO3 → CO2 + H2O decomposition reactions is also expected to be important, especially, in the clean environment of the Earth's atmosphere and/or in extremely hot and humid conditions. The calculated values of the relative rates for the H2O-, (H2O)2-, FA-, AA-, SA- and HO2 radical-assisted H2CO3 decompositions at the temperatures 310 and 320 K are given in the ESI.† Similarly, in the higher altitude and up to 15 km, only the FA- and/or AA-assisted H2CO3 decompositions are the dominant channels among the H2O-, (H2O)2-, FA-, AA-, SA- and HO2 radical-assisted H2CO3 → CO2 + H2O decomposition reactions. For example, with respect to the average concentrations of FA (see ESI†), it is seen from the CCSD(T)/aug-cc-pVTZ level predicted results that at the 5, 10 and 15 km altitudes of the Earth's atmosphere, the rates for the FA-assisted H2CO3 decompositions are respectively ∼102, 105 and 106 times higher than the rates associated with the H2O-assisted decomposition. It is also worth noting here that the OH radical-initiated atmospheric degradation of the gas-phase H2CO3 molecule, which might be another important channel to look at the atmospheric loss of H2CO3 molecules, has not been focused on in this article.
Finally, as mentioned earlier, the RCO2H-assisted H2CO3 decomposition has been studied very recently by Kumar et al.,42,43 and the primary focus of this work was to explore the instability of the gaseous H2CO3 molecule in the presence of carboxylic acids, especially at the 0 km altitude of the Earth's atmosphere. It has been pointed out from their M06-2X/aug-cc-pVTZ level predicted results including tunneling corrections that the formic acid (FA)-assisted H2CO3 decomposition is ∼10 times faster than the H2O-assisted H2CO3 decomposition at 298.15 K. To reproduce this relative reaction rate, we also performed the M06-2X/aug-cc-pVTZ level of calculations for the water monomer-, FA- and AA-assisted H2CO3 decomposition reactions. It is seen from our calculations that although the M06-2X/aug-cc-pVTZ level optimized geometries of the pre-reactive complexes and transition states match well between their calculations and our calculations, there is some inconsistency between their data and our data predicted at the same level of theory, especially, when we consider the comparison of calculated free energy values of the pre-reactive complexes and transition states with respect to the total free energies of isolated starting bimolecular reactants. It is worthwhile to note here that the dissimilarity in the optimized geometries of the exit channel complexes, which we have found when we have compared the optimized geometries of existing channel complexes, is not important here, as the reaction rate calculation in the forward direction does not depend upon the energetics of the exit channel complexes. Therefore, in the ESI,† we have provided a comparison between their data and our data associated with the pre-reactive complexes and transition states as well as the equations by which one can easily reproduce the values of reaction rates that we have given for the water monomer- and FA-assisted H2CO3 decomposition reactions. From our calculations including tunneling corrections according to Gaussian-09 outputs, it is seen that at 298.15 K the FA-assisted H2CO3 decomposition is only ∼2.7 times faster than the H2O-assisted H2CO3 decomposition. It is to be noted here that this prediction is based on the concentrations of water and FA that have been reported by Kumar et al. and not considering the corrections with respect to reaction degeneracy.
4. Summary and conclusion
Carbonic acid (H2CO3), a small molecule of six atoms involving three elements in the periodic table, is right at the interface between organic and inorganic chemistry. We note what has already been noted by other research groups that the detection of gas-phase interstellar H2CO3 as well as H2CO3 in Earth's troposphere has become very challenging for a new generation of scientists, and it is only hoped that one day, in near future, it will be detected as scientists achieve success in measuring the infrared spectra of the vapor phase H2CO3 resulting from its polymorphs via sublimation at cold temperatures.2,5,6,22 Therefore, to understand the stability of the H2CO3 molecule, especially in the Earth's troposphere and lower stratosphere, we focus upon the energetics of the potential energy diagrams as well as the simple relative kinetics for the decomposition of the H2CO3 molecule into its constituents CO2 and H2O molecules (H2CO3 → CO2 + H2O) via the shortest route in the presence of various species which are present not only at significant trace levels in the Earth's atmosphere but also known as effective catalysts in hydrogen atom transfer reactions at the doubly or multiply hydrogen-bonded interfaces via the O–H bond breaking and making processes.46–48,77–83 We have considered the H2CO3 → CO2 + H2O decomposition reaction in the presence of one to three water (H2O) molecules as well as in the presence of formic acid (FA), acetic acid (AA), sulfuric acid (SA) and hydroperoxide (HO2) radical. Overall, 14 different reaction pathways in terms of bimolecular encounters have been considered, and the quantum chemistry calculations have been performed at the MP2/aug-cc-pVDZ, MP2/aug-cc-pVTZ, MP2/6-311++G(3df,3pd) and CCSD(T)/aug-cc-pVTZ levels of theory.
In conclusion, it is seen from our results that the most effective catalysts in the H2CO3 → CO2 + H2O decomposition reaction are FA, AA and SA, as the H2CO3 → CO2 + H2O decomposition reaction in the presence of these species is effectively a near-barrierless process. However, by taking the atmospheric concentrations of water monomer (H2O), dimer [(H2O)2], FA, AA, SA and HO2 radical, we find that the H2O- and (H2O)2-assisted H2CO3 decomposition reactions are important channels to make the gas-phase H2CO3 molecule unstable, especially at 0 km altitude in a clean environment of the Earth's atmosphere. This follows as the reaction rates for the H2O-, (H2O)2-, FA- and AA-assisted H2CO3 decompositions are comparable to each other within a factor of ∼15. Similarly, at the 0 km altitude in a polluted environment of the Earth's atmosphere and in the 5 to 15 km altitude range, only the FA- and/or AA-assisted decompositions are important. Therefore, up to 15 km altitude from the Earth's surface, both SA and HO2 radical do not play any significant role in the H2CO3 → CO2 + H2O decomposition reaction, even though SA is also an effective catalyst like FA and AA. It is worth noting here that the OH radical-initiated atmospheric degradation of the gas-phase H2CO3 molecule, which might be another important channel to understand the atmospheric loss of H2CO3, has not been studied in this article. Moreover, we have focused on H2CO3 → CO2 + H2O decomposition mainly in the lower atmosphere of the Earth and not in the outer space, where especially the H2SO4-assisted H2CO3 → CO2 + H2O decomposition reaction may have an important impact.
Acknowledgements
Financial support from the BARD project (PIC no: 12-R&D-SIN-5.04-0103), Department of Atomic Energy, Government of India, is gratefully acknowledged.
References
- J. K. Terlouw, C. B. Lebrilla and H. Schwarz, Angew. Chem., Int. Ed., 1987, 26, 354–355 CrossRef.
- S. E. Huber, S. Dalnodar, W. Kausch, S. Kimeswenger and M. Probst, AIP Adv., 2012, 2, 032180 CrossRef PubMed.
- T. Mori, K. Suma, Y. Sumiyoshi and Y. Endo, J. Chem. Phys., 2009, 130, 204308 CrossRef PubMed.
- T. Mori, K. Suma, Y. Sumiyoshi and Y. Endo, J. Chem. Phys., 2011, 134, 044319 CrossRef PubMed.
- J. Bernard, M. Seidl, I. Kohl, K. R. Liedl, E. Mayer, O. Galvez, H. Grothe and T. Loerting, Angew. Chem., Int. Ed., 2011, 50, 1939–1943 CrossRef CAS PubMed.
- J. Bernard, R. G. Huber, K. R. Liedl, H. Grothe and T. Loerting, J. Am. Chem. Soc., 2013, 135, 7732–7737 CrossRef CAS PubMed.
- N. DelloRusso, R. K. Khanna and M. H. Moore, J. Geophys. Res.: Planets, 1993, 98, 5505–5510 CrossRef.
- M. H. Moore, R. Khanna and B. Donn, J. Geophys. Res.: Planets, 1991, 96, 17541–17545 CrossRef CAS.
- M. H. Moore and R. K. Khanna, Spectrochim. Acta, Part A, 1991, 47, 255–262 CrossRef.
- J. R. Brucato, M. E. Palumbo and G. Strazzulla, Icarus, 1997, 125, 135–144 CrossRef CAS.
- G. Strazzulla, G. Leto, F. Spinella and O. Gomis, Astrobiology, 2005, 5, 612–621 CrossRef CAS PubMed.
- P. A. Gerakines, M. H. Moore and R. L. Hudson, Astron. Astrophys., 2000, 357, 793–800 CAS.
- C. Y. R. Wu, D. L. Judge, B.-M. Cheng, T.-S. Yih, C. S. Lee and W. H. Ip, J. Geophys. Res.: Planets, 2003, 108, 5032 CrossRef.
- M. Garozzo, D. Fulvio, O. Gomis, M. E. Palumbo and G. Strazzulla, Planet. Space Sci., 2008, 56, 1300–1308 CrossRef CAS PubMed.
- Y. Oba, N. Watanabe, A. Kouchi, T. Hama and V. Pirronello, Astrophys. J., 2010, 722, 1598–1606 CrossRef CAS.
- J. S. Lewis and D. H. Grinspoon, Science, 1990, 249, 1273–1275 CAS.
- H. A. Al-Hosney and V. H. Grassian, J. Am. Chem. Soc., 2004, 126, 8068–8069 CrossRef CAS PubMed.
- R. K. Khanna, J. A. Tossell and K. Fox, Icarus, 1994, 112, 541–544 CrossRef CAS.
- W. Hage, A. Hallbrucker and E. Mayer, J. Chem. Soc., Faraday Trans., 1995, 91, 2823–2826 RSC.
- G. Strazzulla, J. R. Brucato, G. Cimino and M. E. Palumbo, Planet. Space Sci., 1996, 44, 1447–1450 CrossRef CAS.
- W. Hage, K. R. Liedl, A. Hallbrucker and E. Mayer, Science, 1998, 279, 1332–1335 CrossRef CAS.
- R. L. Hudson, J. Chem. Educ., 2006, 83, 1611–1616 CrossRef CAS.
- Z. Peeters, R. L. Hudson, M. H. Moore and A. Lewis, Icarus, 2010, 210, 480–487 CrossRef CAS PubMed.
- H. Falcke and S. H. Eberle, Water Res., 1990, 24, 685–688 CrossRef CAS.
- K. Adamczyk, M. Premont-Schwarz, D. Pines, E. Pines and E. T. J. Nibbering, Science, 2009, 326, 1690–1694 CrossRef CAS PubMed.
- W. Hage, A. Hallbrucker and E. Mayer, J. Am. Chem. Soc., 1993, 115, 8427–8431 CrossRef CAS.
- K. Winkel, W. Hage, T. Loerting, S. L. Price and E. Mayer, J. Am. Chem. Soc., 2007, 129, 13863–13871 CrossRef CAS PubMed.
- I. Kohl, K. Winkel, M. Bauer, K. R. Liedl, T. Loerting and E. Mayer, Angew. Chem., Int. Ed., 2009, 48, 2690–2694 CrossRef CAS PubMed.
- C. Mitterdorfer, J. Bernard, F. Klauser, K. Winkel, I. Kohl, K. R. Liedl, H. Grothe, E. Mayer and T. Loerting, J. Raman Spectrosc., 2012, 43, 108–115 CrossRef CAS.
- S. K. Reddy, C. H. Kulkarni and S. Balasubramanian, J. Phys. Chem. A, 2012, 116, 1638–1647 CrossRef CAS PubMed.
- J. Murillo, J. David and A. Restrepo, Phys. Chem. Chem. Phys., 2010, 2, 10963–10970 RSC.
- T. Loerting and J. Bernard, ChemPhysChem, 2010, 11, 2305–2309 CrossRef CAS PubMed.
- J. Bernard, M. Seidl, E. Mayer and T. Loerting, ChemPhysChem, 2012, 13, 3087–3091 CrossRef CAS PubMed.
- A. G. Galinos and A. A. Carotti, J. Am. Chem. Soc., 1961, 63, 752 CrossRef.
- G. Gattow and U. Gerwarth, Angew. Chem., Int. Ed., 1965, 4, 149 CrossRef.
- H. P. Reisenauer, J. P. Wagner and P. R. Schreiner, Angew. Chem., Int. Ed., 2014, 53, 11766–11771 CrossRef CAS PubMed.
- Y. Oba, N. Watanabe, A. Kouchi, T. Hama and V. Pirronello, Phys. Chem. Chem. Phys., 2011, 13, 15792–15797 RSC.
- S. Ghoshal and M. K. Hazra, J. Phys. Chem. A, 2014, 118, 2385–2392 CrossRef CAS PubMed.
- T. Loerting, C. Tautermann, R. T. Kroemer, I. Kohl, A. Hallbrucker, E. Mayer and K. R. Liedl, Angew. Chem., Int. Ed., 2000, 39, 891–894 CrossRef.
- C. S. Tautermann, A. F. Voegele, T. Loerting, I. Kohl, A. Hallbrucker, E. Mayer and K. R. Liedl, Chem.–Eur. J., 2002, 8, 66–73 CrossRef CAS.
- S. A. de Marothy, Int. J. Quantum Chem., 2013, 113, 2306–2311 CrossRef.
- M. Kumar, D. H. Busch, B. Subramaniam and W. H. Thompson, J. Phys. Chem. A, 2014, 118, 5020–5028 CrossRef CAS PubMed.
- M. Kumar, D. H. Busch, B. Subramaniam and W. H. Thompson, J. Phys. Chem. A, 2014, 118, 10155–10156 CrossRef CAS.
- S. G. Lakkis, M. Lavorato and P. O. Canziani, Atmos. Res., 2009, 92, 18–26 CrossRef PubMed.
- R. Spang, G. Günther, M. Riese, L. Hoffmann, R. Müller and S. Griessbach, Atmos. Chem. Phys. Discuss., 2014, 14, 12323–12375 CrossRef.
- R. J. Buszek, A. Sinha and J. S. Francisco, J. Am. Chem. Soc., 2011, 133, 2013–2015 CrossRef CAS PubMed.
- J. S. Francisco, M. Torrent-Sucarrat and J. M. Anglada, J. Am. Chem. Soc., 2012, 134, 20632–20644 CrossRef PubMed.
- J. Gonzalez, M. Torrent-Sucarrat and J. M. Anglada, Phys. Chem. Chem. Phys., 2010, 12, 2116–2125 RSC.
- M. J. Frisch, G. W. Trucks, H. B. Schlegel, G. E. Scuseria, M. A. Robb, J. R. Cheeseman, G. Scalmani, V. Barone, B. Mennucci, G. A. Petersson, H. Nakatsuji, M. Caricato, X. Li, H. P. Hratchian, A. F. Izmaylov, J. Bloino, G. Zheng, J. L. Sonnenberg, M. Hada, M. Ehara, K. Toyota, R. Fukuda, J. Hasegawa, M. Ishida, T. Nakajima, Y. Honda, O. Kitao, H. Nakai, T. Vreven, J. A. Montgomery Jr, J. E. Peralta, F. Ogliaro, M. Bearpark, J. J. Heyd, E. Brothers, K. N. Kudin, V. N. Staroverov, R. Kobayashi, J. Normand, K. Raghavachari, A. Rendell, J. C. Burant, S. S. Iyengar, J. Tomasi, M. Cossi, N. Rega, J. M. Millam, M. Klene, J. E. Knox, J. B. Cross, V. Bakken, C. Adamo, J. Jaramillo, R. Gomperts, R. E. Stratmann, O. Yazyev, A. J. Austin, R. Cammi, C. Pomelli, J. W. Ochterski, R. L. Martin, K. Morokuma, V. G. Zakrzewski, G. A. Voth, P. Salvador, J. J. Dannenberg, S. Dapprich, A. D. Daniels, Ö. Farkas, J. B. Foresman, J. V. Ortiz, J. Cioslowski and D. J. Fox, Gaussian 09, Revision B.01, Gaussian, Inc., Wallingford, CT, 2010 Search PubMed.
- J. R. Alvarez-Idaboy and A. Galano, Theor. Chem. Acc., 2010, 126, 75–85 CrossRef CAS.
- K. R. Liedl, S. Sekusak and E. Mayer, J. Am. Chem. Soc., 1997, 119, 3782–3784 CrossRef CAS.
- B. Reimann, K. Buchhold, H.-D. Barth, H.-D. Brutschy, P. Tarakeshwar and K. S. Kim, J. Chem. Phys., 2002, 117, 8805–8822 CrossRef CAS PubMed.
- P. P. Kumar, A. G. Kalinichev and R. J. Kirkpatrick, J. Chem. Phys., 2007, 126, 204315 CrossRef PubMed.
- P. Ballone, B. Montanari and R. O. Jones, J. Chem. Phys., 2000, 112, 6571–6575 CrossRef CAS PubMed.
- S. Ghoshal and M. K. Hazra, J. Phys. Chem. A, 2014, 118, 4620–4630 CrossRef CAS PubMed.
- A. Razavi, F. Karagulian, L. Clarisse, D. Hurtmans, P. F. Coheur, C. Clerbaux, J. F. Müller and T. Stavrakou, Atmos. Chem. Phys., 2011, 11, 857–872 CrossRef CAS.
- M. W. Shephard, A. Goldman, S. A. Clough and E. J. Mlawer, J. Quant. Spectrosc. Radiat. Transfer, 2003, 82, 383–390 CrossRef CAS.
- J. E. Lawrence and P. Koutrakls, Environ. Sci. Technol., 1994, 28, 957–964 CrossRef CAS PubMed.
- S. R. Souza and L. R. F. Carvalho, J. Braz. Chem. Soc., 2001, 12, 755–762 CrossRef CAS PubMed.
- M. Grutter, N. Glatthor, G. P. Stiller, H. Fischer, U. Grabowski, M. Höpfner, S. Kellmann, A. Linden and T. von Clarmann, J. Geophys. Res.: Planets, 2010, 115, D10303 CrossRef.
- G. González
Abad, P. F. Bernath, C. D. Boone, S. D. McLeod, G. L. Manney and G. C. Toon, Atmos. Chem. Phys., 2009, 9, 8039–8047 Search PubMed.
- S. R. Souza, P. C. Vasconcellos and L. R. F. Carvalho, Atmos. Environ., 1999, 33, 2563–2574 CrossRef CAS.
- R. W. Talbot, J. E. Dibb, B. L. Lefer, E. M. Scheuer, J. D. Bradshew, S. T. Sandholm, S. Smyth, D. R. Blake, N. J. Blake, G. W. Sachse, J. E. Collins and G. L. Gregory, J. Geophys. Res.: Planets, 1997, 102, 28303–28313 CrossRef CAS.
- E. Sanhueja, L. Figueroa and M. Santana, Atmos. Environ., 1996, 30, 1861–1873 CrossRef.
- A. Chebbi and P. Carlier, Atmos. Environ., 1996, 30, 4233–4249 CrossRef CAS.
- P. Khare, G. S. Satsangi, N. Kumar, K. M. Kumari and S. S. Srivastav, Atmos. Environ., 1997, 31, 3867–3875 CrossRef CAS.
- K. Granby and C. S. Christensen, Atmos. Environ., 1997, 31, 1403–1415 CrossRef CAS.
- T. Reiner, O. Möhler and F. Arnold, J. Geophys. Res.: Planets, 1999, 104, 13943–13952 CrossRef CAS.
- M. O. Andreae, R. W. Talbot, T. W. Andreae and R. C. Harriss, J. Geophys. Res., 1988, 93, 1616–1624 CrossRef CAS.
- R. P. Turco, P. Hamill, O. B. Toon, R. C. Whitten and C. S. Kiang, J. Atmos. Sci., 1979, 36, 699–717 CrossRef CAS.
- M. J. Mills, O. B. Toon, V. Vaida, P. E. Hintze, H. G. Kjaergaard, D. P. Schofield and T. W. Robinson, J. Geophys. Res.: Planets, 2005, 110, D08201 Search PubMed.
- J. Mao, D. J. Jacob, M. J. Evans, J. R. Olson, X. Ren, W. H. Brune, J. M. S. Clair, J. D. Crounse, K. M. Spencer, M. R. Beaver, P. O. Wennberg, M. J. Cubison, J. L. Jimenez, A. Fried, P. Weibring, J. G. Walega, S. R. Hall, A. J. Weinheimer, R. C. Cohen, G. Chen, J. H. Crawford, C. McNaughton, A. D. Clarke, L. Jaegle, J. A. Fisher, R. M. Yantosca, P. Le Sager and C. Carouge, Atmos. Chem. Phys., 2010, 10, 5823–5838 CrossRef CAS.
- D. Kubistin, H. Harder, M. Martinez, M. Rudolf, R. Sander, H. Bozem, G. Eerdekens, H. Fischer, C. Gurk, T. Klüpfel, R. Königstedt, U. Parchatka, C. L. Schiller, A. Stickler, D. Taraborrelli, J. Williams and J. Lelieveld, Atmos. Chem. Phys., 2010, 10, 9705–9728 CAS.
- G. P. Brasseur and S. Solomon, Aeronomy of the Middle Atmosphere, Springer, Netherlands, 2005 Search PubMed.
- W. B. DeMore, S. P. Sander, D. M. Golden, R. F. Hampson, M. J. Kurylo, C. J. Howard, A. R. Ravishankara, C. E. Kolb and M. J. Molina, Chemical Kinetics and Photochemical Data for Use in Stratospheric Modeling, JPL Publication, Jet Propulsion Laboratory, California Institute of Technology, Pasadena, CA, 1997, pp. 97–4 Search PubMed.
- J. M. Anglada, G. J. Hoffman, L. V. Slipchenko, M. M. Costa, M. F. Ruiz-López and J. S. Francisco, J. Phys. Chem. A, 2013, 117, 10381–10396 CrossRef CAS PubMed.
- M. K. Hazra and A. Sinha, J. Am. Chem. Soc., 2011, 133, 17444–17453 CrossRef CAS PubMed.
- B. Long, Z.-w. Long, Y.-b. Wang, X.-f. Tan, Y.-h. Han, C.-y. Long, S.-j. Qin and W.-j. Zhang, ChemPhysChem, 2012, 13, 323–329 CrossRef CAS PubMed.
- M. K. Hazra, J. S. Francisco and A. Sinha, J. Phys. Chem. A, 2013, 117, 11704–11710 CrossRef CAS PubMed.
- M. K. Hazra, J. S. Francisco and A. Sinha, J. Phys. Chem. A, 2014, 118, 4095–4105 CrossRef CAS PubMed.
- G. da Silva, Angew. Chem., Int. Ed., 2010, 49, 7523–7525 CrossRef CAS PubMed.
- M. K. Hazra and T. Chakraborty, J. Phys. Chem. A, 2005, 109, 7621–7625 CrossRef CAS PubMed.
- M. K. Hazra and T. Chakraborty, J. Phys. Chem. A, 2006, 110, 9130–9136 CrossRef CAS PubMed.
- H. Eyring, J. Chem. Phys., 1935, 3, 107–115 CrossRef CAS PubMed.
- M. G. Evans and M. Polanyi, Trans. Faraday Soc., 1935, 31, 875–894 RSC.
- D. G. Truhlar, W. L. Hase and J. T. Hynes, J. Phys. Chem., 1983, 87, 2664–2682 CrossRef CAS.
- C. Eckart, Phys. Rev., 1930, 35, 1303–1309 CrossRef CAS.
- R. L. Brown, J. Res. Natl. Bur. Stand., 1981, 86, 357–359 CrossRef CAS.
- H. S. Johnston and J. Heicklen, J. Phys. Chem., 1962, 66, 532–533 CrossRef.
- L. Arnaut, S. Formosinho and H. Burrows, Chemical Kinetics from Molecular Structure to Chemical Reactivity, Elsevier, Netherlands, 2007 Search PubMed.
- D. A. McQuarrie, Statistical Thermodynamics, Harper and Row, New York, 1973 Search PubMed.
- G. J. Frost and V. Vaida, J. Geophys. Res.: Planets, 1995, 100, 18803–18809 CrossRef.
Footnote |
† Electronic supplementary information (ESI) available: The computed total electronic energies (Etotal), ZPE corrected electronic energies [Etotal(ZPE)], imaginary frequencies of various transition states (TS), rate constants as well as the other data as mentioned in the text. See DOI: 10.1039/c4ra13233e |
|
This journal is © The Royal Society of Chemistry 2015 |
Click here to see how this site uses Cookies. View our privacy policy here.