DOI:
10.1039/C4RA16340K
(Paper)
RSC Adv., 2015,
5, 17615-17622
Root exudates as natural ligands that alter the properties of graphene oxide and environmental implications thereof†
Received
13th December 2014
, Accepted 4th February 2015
First published on 4th February 2015
Abstract
In the present work, we identified root exudates that were stimulated by pristine graphene oxide (PGO), including small-molecule acids, alcoholates, alkanes, amino acids and secondary metabolites. These exudates acted as ligands and became immobilized on the PGO to form ligand-GO complexes (LGO). Compared with PGO, LGO exhibited increased thickness, a higher C
:
O ratio, and reduced transparency and size. Nitrogen- and sulfur-containing groups were observed in LGO. LGO, with its decreased negative charges, was less stable than PGO. In addition, LGO exhibited more unpaired electrons and disordered structures and greater UV absorption compared with PGO. The above alterations in the properties of PGO that occurred after modification of the PGO by exudates induced significant malformations (abnormal tail flexure and pericardial edema) and the loss of mitochondrial membrane polarization in zebrafish as a model organism. This work revealed that root exudates act as natural ligands and significantly alter the properties of PGO.
Introduction
Graphene oxide (GO) is widely researched and applied in various fields, such as biology, chemistry, medicine, and contamination remediation.1–3 Reports have indicated that the market for GO-based products may reach %675 million by 2020.4 The rapidly increasing production and application of GO create a high potential for its environmental release at significant levels.5 Researchers are concerned about the phytotoxicity of pristine GO (PGO); for example, PGO has been found to inhibit growth, damage cellular structures, reduce chlorophyll content, and induce oxidative stress or genotoxicity in plants.6–9 However, PGO, with its thickness of approximately 1 nm, is not inert in the natural environment. Prior to the scientific evaluation of the environmental risks of GO, the modifications to PGO caused by environmental factors and organisms should be globally understood.10–12 Our recent work has demonstrated that the nanosheet morphology of graphene-based materials transforms into a ribbon morphology in water under visible-light irradiation. We have also demonstrated that natural organic matter acts as ligands that can spontaneously immobilize on graphene, resulting in changes to the environmental fate and risks of PGO.13,14 Previous studies also support the hypothesis that microorganisms can alter the surface chemistry of PGO via electron transfer.15–19 As primary producers, plants are essential components of the ecosystem. However, the effects of GO on the morphology, structure, and surface chemistry of plants and the related risks are unknown.5,20–22
The primary functions of the ‘hidden’ part of the plant, its root system, have traditionally been thought to anchor plants in the soil and to facilitate nutrient and water uptake. However, roots also excrete an enormous variety of compounds into the surrounding environment.23 Root exudation is a component of rhizodeposition and is now considered to be a key aspect of the complex interactions that occur in the rhizosphere, such as plant–pollutant interactions.24–26 For example, under stress conditions, such as exposure to heavy metals, plant roots can excrete electrolytes, sugars, organic acids, amino acids, enzymes, endogenous hormones, and some secondary metabolites as a defense mechanism.27 Despite recent advances in our understanding of how root exudates influence the bioavailability of arsenic, heavy metals and other traditional pollutants, the environment significance of root exudates in relation to nanomaterials remains unclear. Compared with other nanomaterials, PGO, with its specific nanosheet morphology, oxygen-containing groups and relative stability, is prone to binding small molecules.5 Consequently, the properties of and environmental risks associated with PGO could be altered if root exudates act as natural ligands and become immobilized on PGO.
To confirm the above hypotheses, we first identified the root exudates of rice (Oryza sativa L.), a model monocot plant, following stimulation with PGO. These root exudates acted as natural ligands, forming ligand-GO complexes (LGO). Subsequently, the morphological and surface chemical alterations of LGO with respect to PGO were characterized. The size distributions and surface charges of LGO and PGO were also investigated. To reveal alterations in chemical activity, the unpaired elections, disordered structures and UV-vis absorption of PGO and LGO were analyzed. Finally, zebrafish were used as a model organism; the effects of PGO and LGO on the hatching, survival, development and mitochondrial functions of zebrafish were analyzed to gain a preliminary understanding of the environmental implications of root exudates as natural ligands of nanomaterials.
Results and discussion
Identification of root exudates acting as natural ligands
GC-MS/MS was conducted to identify the root exudates, as shown in Tables S1–S3.† A total of 200 peaks from each sample were analyzed, and 62 root exudates were identified, as shown in Fig. 1. These root exudates included alcohols, phenols, terpenoids, organic acids, sugars, amino acids, esters, aldehydes, alkenes, alkanes, and other small molecules. In the control (without PGO exposure), 25 exudates were detected. In the aqueous phase from plants exposed to PGO and in the adsorbed phase (LGO), 31 and 47 exudates were detected, respectively. As is clearly indicated in Fig. 1, the contents of alanine, octadecenoic acid, ethanol, pentanoic acid, octanol, octadecynoic acid, ethanimidic acid, oleyl alcohol, isoborneol, and geranylgeraniol increased in the group exposed to PGO compared with the control group. The external stimulus provided by PGO exposure enhanced exudation from the roots, as expected from plant defense.28 Compared with the exudates identified in the aqueous phase from plants exposed to PGO, the adsorbed phase (LGO) exhibited high contents of thymol, D-mannitol, 2-monostearin, azelaic acid, phthalic acid, salicylic acid, myristic acid, 1,4-benzenedicarboxylic acid, coronene, and ethanedioic acid. In the PGO exposure group, the distribution of exudates between the aqueous phase and the adsorbed phase was determined by the chemical properties of both the exudates and PGO.29 The organic acids found in the adsorbed phase contained one or more carboxyl groups, and the secondary metabolites in the adsorbed phase had benzene rings. PGO is known to contain many oxygen groups, such as –OH, –COOH and –CHO, and specific nanostructures, such as nanopores, active edges, dangling carbons, and sp2 structures.30,31 The abovementioned chemical groups of the exudates could bind PGO via hydrogen bonds and π–π bonds. These data are the first to demonstrate that PGO exposure stimulates exudation from roots, leading to the immobilization of many small molecules on the PGO and resulting in the formation of LGO. The FTIR spectra of PGO and LGO were presented in Fig. S2.† Compared with PGO, the FTIR spectrum of LGO contained stronger peaks corresponding to the groups of ethers and epoxides, as well as the peak corresponding to the new functional group of methylene, suggesting the oxygen-containing molecules from exudates bonding to PGO. The oxygen-containing groups were attributed to the defects in graphene networks, particularly the defects located on the edge.14 The C
C groups of PGO shifted from 1621 to 1650 cm−1, suggesting that the small molecules contained C
C groups were located on the sp2 plane of graphene through π–π interactions. Recent studies also suggested that PGO can adsorb lysozyme by electrostatic interactions32 and benzene hydrocarbon by the planarity effect, which increased planarity between the planar congeners and the graphene surface.33 Plant roots can excrete organic acids, amino acids, enzymes, endogenous hormones, and some secondary metabolites.27 The adsorption of these exudates by PGO, may be the combined actions of abovementioned physical and chemical interactions.
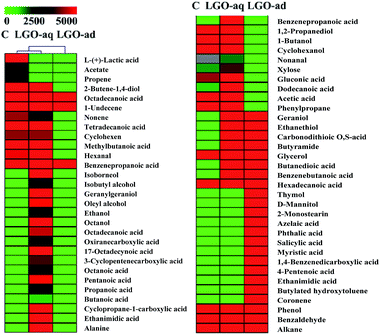 |
| Fig. 1 Relative contents of plant root exudates represented by heat maps. The cluster analysis of plant root exudates was performed using hierarchical clustering. C denotes the root exudates from the control without PGO exposure, LGO-aq denotes the exudates in the aqueous phase from plants exposed to PGO, and LGO-ad denotes the exudates in the adsorbed phase from plants exposed to PGO. All root exudates were detected in triplicate samples. Detailed data are provided in the ESI (Tables S1–S3†). | |
Exudate-induced alterations of the surface morphology of PGO
The morphology of a nanomaterial determines its environmental fate and nanotoxicity.5,34,35 SEM, TEM and AFM were used to study the morphology of PGO before and after its modification by exudates. As demonstrated by the SEM images presented in Fig. 2, both PGO and LGO exhibited a nanosheet morphology; however, LGO had a rougher texture than PGO. In the TEM images presented in Fig. 2, it is evident that the transparency of the LGO nanosheets is lower than that of the PGO nanosheets because of the modification by the exudates. As shown in the left-hand AFM image in Fig. 3, the PGO exhibited a layer morphology with a thickness of 0.862 nm, consistent with the 0.8–1.0 nm thickness previously reported for single-layer GO.36 In the right-hand AFM image presented in Fig. 3, the smaller and larger LGO nanosheets exhibited thicknesses of 1.591 nm and 3.856 nm, respectively. The average thickness of the LGO nanosheets was 2.265 nm. The thickness of the nanosheets after the immobilization of the exudates was markedly higher than that observed for PGO, as expected. The different sizes observed for the LGO nanosheets were attributed to the extensive ultrasonication applied during AFM pretreatment.37 The size distributions of the PGO and LGO nanosheets were accurately measured using the ZETAPALS/BI-200SM instrument, as described in the following section.
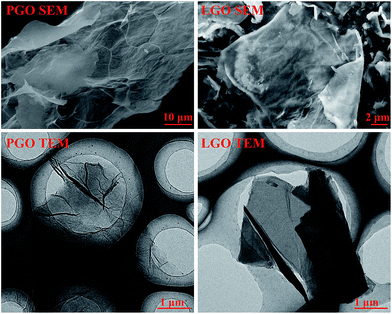 |
| Fig. 2 Scanning electron microscopy (SEM) and field-emission transmission electron microscopy (TEM) images of PGO (pristine graphene oxide) and LGO (root exudates as ligand-graphene oxide complexes). | |
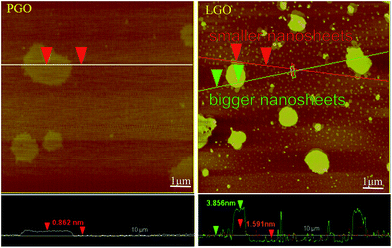 |
| Fig. 3 Atomic force microscope (AFM) images of PGO (pristine graphene oxide) and LGO (root exudates as ligand-graphene oxide complexes). | |
Exudate-induced alteration of the size distribution and surface charge of PGO
The size distribution and the surface charge play dominant roles in the environmental behavior of nanomaterials and their associated ecological risks.5,38,39 As shown in Fig. 4a, the size distribution of the PGO ranged between 342 nm and 450 nm, and the size distribution of the LGO ranged between 142 nm and 220 nm. These data demonstrated that the root exudates reduced the size of the PGO, although the detailed mechanisms driving this change in size distribution remain unclear. The zeta potential as a function of pH is presented in Fig. 4b. The surface charges of PGO and LGO were both negative at environmentally relevant pH values (pH 5–9). The zeta potential results also supported the hypothesis that PGO, with its greater negative charge, was more stable than LGO at the tested pH. In general, the surfaces of cells carry negative charges; this finding therefore implies that LGO binds to cells more readily than PGO via electrostatic interactions.40 The observed decrease in negative charges directly related to the presence of exudates with a high ratio of carbon to oxygen.
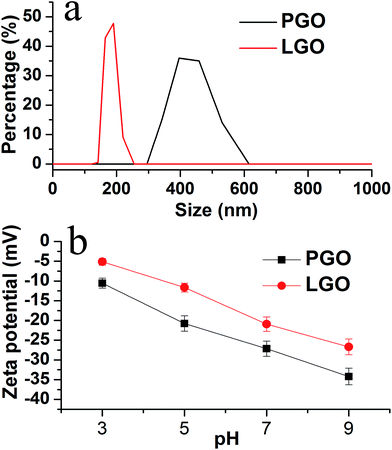 |
| Fig. 4 Effects of exudate ligands on the size distribution (a) and surface charge (b) of the nanomaterials. The size distributions and zeta potentials of the nanosheets were measured using a ZETAPALS/BI-200SM instrument equipped with a 30 mW, 635 nm laser (Brookhaven, USA). The surface charge was evaluated by measuring the zeta potential at various pH values. | |
Exudate-induced alterations of the surface chemical groups of PGO
The alterations in the surface chemistry of PGO were investigated via XPS. The full spectra of PGO and LGO are presented in Fig. S1.† The spectrum of PGO consisted of 69.73% C1s and 30.27% O1s, whereas the spectrum of LGO consisted of 71.49% C1s, 25.06% O1s, 3.30% N1s, and 0.15% S2p. The observed increase in the ratio of carbon to oxygen (C
:
O) from PGO to LGO was caused by the immobilization of ligands with a high carbon content, such as coronene, azelaic acid, monostearin and thymol, as shown in Fig. 1. Furthermore, methylene was detected from the FTIR spectra presented in Fig. S2,† demonstrating the introduction of a carbon chain on LGO, which supports the increased ratio of C/O. Fig. 5 presents the specific groups for the above components. The C1s spectrum of PGO revealed that 44.98% of the carbon signal (C–C/C
C) originated from sp2 structures and that 55.02% of the carbon signal (C–O/C
O) originated from sp3 structures such as hydroxyl and epoxide groups.41 The C1s spectrum of LGO was a combination of C–C/C
C (28.25%), C–O (29.74%), C
O (14.98%) and C–N (27.03%). Compared with PGO, the observation of N1s and S2p signals suggested the presence of nitrogen-containing and sulfur-containing groups on the LGO surface, consistent with the immobilization of exudate ligands described in Fig. 1 and the groups detected using FTIR spectra presented in Fig. S2.† Nitrogen-containing compounds, such as alanine and ethanimidic acid, were detected in the analysis of the exudate ligands. Sulfur-containing exudates, such as carbonodithioic O,S-acid and benzenesulfonic acid, were found in the exposure group. The N1s spectrum of LGO exhibited two peaks at 399.6 eV and 401.6 eV, corresponding to pyrrolic N and graphitic N, respectively.42 The observation of 11.32% graphitic N suggested that a small amount of nitrogen was incorporated into the graphene networks, whereas the presence of 88.68% pyrrolic N suggested that most of the nitrogen was immobilized on the planes, edges or defects in the graphene networks. The specific components of the S2p signals of LGO at 163.7 eV and 168.7 eV were ascribed to S–H and S–O bonds, respectively.43,44 The XPS results agreed with the results of the exudate analysis, and confirmed the immobilization of the exudate ligands. These results indicated that nitrogen- and sulfur-containing groups were present on the LGO, consistent with the thickness and surface charges observed for LPO.
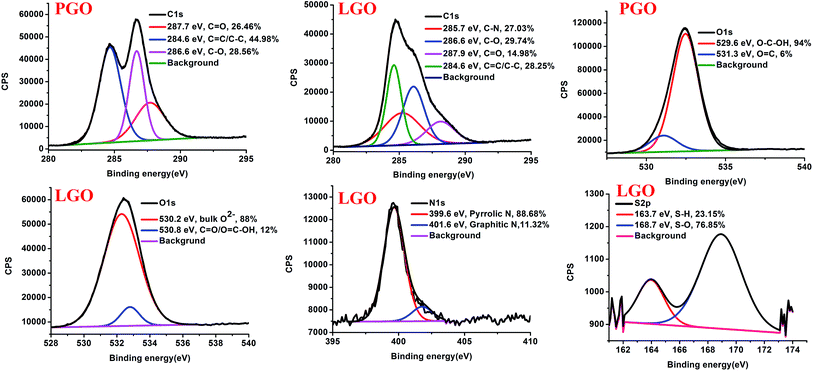 |
| Fig. 5 X-ray photoelectron spectra (XPS) of PGO (pristine graphene oxide) and LGO (root exudates as ligand-graphene oxide complexes), including C1s, O1s, N1s, and S2p spectra. | |
Exudate-induced alterations of the chemical activity of PGO
The unpaired electrons on the surfaces of nanomaterials, which are associated with defects, directly affect the chemical activity and nanotoxicity of the nanomaterials.45–47 To measure the unpaired electrons on the surfaces of the investigated nanomaterials, EPR was performed, using TEMPO as the unpaired electron probe. As shown in Fig. 6a, both PGO and LGO exhibited more intense signals than did the control, suggesting that PGO and LGO have the potential to produce unpaired electrons. The LGO exhibited lightly more unpaired electrons than did the PGO, suggesting that the immobilization of exudate ligands may enhance the chemical activity of PGO. Unpaired electrons are known to be associated with disordered structures (such as dangling bonds, turns and kinks on the edges or inner planes) in carbon-based nanomaterials.5,48 The disordered structures of PGO and LGO were assessed based on the Raman spectra, as shown in Fig. 6b. The D band at 1363 cm−1 and the G band at 1593 cm−1 reflect the disordered structure and the ordered sp2 carbon system of graphene, respectively.49 The intensity ratios of the D band to the G band (D/G) for PGO and LGO were 1.15 and 1.86, respectively, suggesting that the exudate ligands enhanced the disorder in the structure of PGO. This result was consistent with the observation of the enhancement of unpaired electrons in LPO. The UV-vis absorption spectra reflect the photochemical activity of PGO and LGO and are presented in Fig. 6c. The largest peaks in these spectra for PGO and LGO, located at approximately 202–204 nm, reflect the n–π* and π–π* (E2 band) transitions.50 The peak in the LGO spectrum at 202–204 nm was markedly higher than that in the PGO spectrum; this peak corresponds to –COOH, –NH2–, –S–, unsaturated ketone groups and unpaired electrons from the ligands. Compared with that of PGO, the UV-vis absorption of LGO decreased remarkably in the region between 240 and 800 nm, suggesting that the electronic conjugation within the graphene sheets was disrupted upon the immobilization of the exudates.51 LGO, with its high rate of n–π*/π–π* transitions, exhibited weak absorption under visible light and strong absorption under UV irradiation compared with PGO.
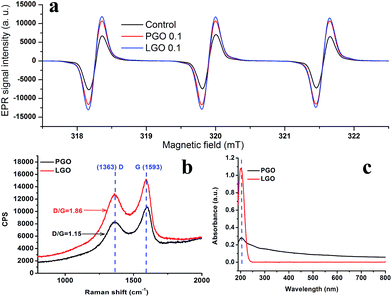 |
| Fig. 6 Effects of exudate ligands on the chemical activity of PGO: electron paramagnetic resonance spectra (a), Raman spectra (b), and UV-vis spectra (c). For the acquisition of the electron paramagnetic resonance spectra, 2,6,6-tetramethyl-1-piperidinyloxy (TEMPO) was employed as an unpaired electron scavenger. The group exposed to TEMPO was used as the control. In the Raman spectra, the D and G peaks denote the typical Raman spectral peaks of PGO. D/G denotes the intensity ratio of the D band to the G band. | |
Environmental implications
There is no doubt that changes in the properties of materials may alter their ecological risks. For example, the morphology, structure and surface chemistry of carbon nanotubes regulate their stability in environmental media and under oxidative stress in vivo.52–54 As shown in Fig. 4, the exudate ligands reduced the negative charges on the surface and the stability of PGO at environmentally relevant pH values. Nanotoxicity is the comprehensive result of nanomaterial properties. To reveal alterations in the nanotoxicity of PGO caused by the immobilization of the exudates, the effects of PGO and LGO on the development of zebrafish were investigated; the findings are presented in Fig. 7. Compared with the control, which demonstrated a hatching rate of 83.3%, both PGO and LGO caused significantly delayed hatching: hatching rates of 63.4–68.5% were observed at 72 hpf following treatment with PGO and LGO at 1 and 10 mg L−1. There were no significant differences in hatching observed between the PGO and LGO groups. Compared with the control, treatment with PGO and LGO did not lead to any remarkable death of embryos. However, LGO induced significant tail flexure abnormalities and pericardial edema; malformations were observed at rates of up to 4.2–5.8% at LGO concentrations of 1–10 mg L−1. When a low concentration of nanomaterials (0.1 mg L−1) was used, no significant differences were observed among all groups. Mitochondria are particularly susceptible to nanoparticles, and ZnO and silver nanoparticles have been found to induce significant losses in mitochondrial membrane potential.55,56 As shown in Fig. 8, alterations in mitochondrial membrane potential were investigated using JC-1 dye staining; a reduction in membrane potential was observed in the embryos treated with PGO and LGO at 96 hpf. Compared with the control, a significant increase in green fluorescence intensity was observed in embryos exposed to PGO and LGO at 0.1–10 mg L−1, indicating that the mitochondria experienced a loss of membrane polarization, in turn corresponding to a loss of function. The ratio of red to green fluorescence decreased by 17.9–75.5% in the LGO compared with the PGO. These results indicate that PGO can enhance the rate of malformation and induce a loss of membrane polarization at the tested concentrations; however, environmentally relevant concentrations are unknown. In general, modifications caused by aging and environmental factors play a passivating role in nanomaterials such as TiO2, ZnO and nanotubes, enhancing their stability and reducing oxidative stress in vivo.20,21,57 In the present work, the exudate ligands from rice reduced the stability and increased the chemical activity of PGO; these changes triggered higher malformation rates and the loss of mitochondrial membrane polarization in zebrafish.
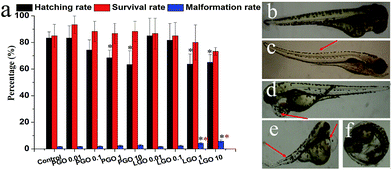 |
| Fig. 7 Effects of PGO and LGO on the hatching, survival and malformation rates of zebrafish: a histogram describing the effects of PGO and LGO on zebrafish development (a); the control, without exposure to PGO or LGO (b); tail flexure in zebrafish exposed to PGO (c); pericardial edema in zebrafish exposed to LGO (d); tail flexure and pericardial edema in zebrafish exposed to LGO (e); and hatching delay in zebrafish exposed to LGO, at 72 hpf (f). The red arrows indicate malformations, including tail flexure and pericardial edema. The black and red asterisks represent significant differences at a level of p < 0.05 compared with the control and PGO groups, respectively. | |
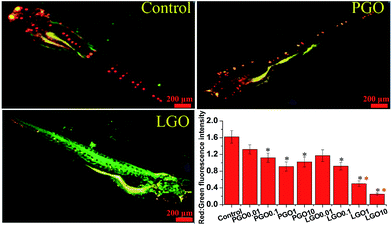 |
| Fig. 8 Effects of PGO and LGO on the mitochondrial membrane potential of zebrafish. The black and red asterisks represent significant differences at a level of p < 0.05 compared with the control and PGO groups, respectively. | |
Conclusions
In summary, we found that GO could stimulate roots to excrete specific compounds that act as natural ligands. These compounds became immobilized on the GO to form ligand-GO complexes (LGO). LGO exhibited smaller size, increased thickness, slightly lower stability, less negative charge and more unpaired electrons on its surface compared with pristine GO (PGO). These alterations of the properties of the material have significant environmental implications; for example, LGO induced remarkable malformations and caused greater damage to mitochondria during zebrafish development than did PGO.
Experimental
Rice experiments
Rice (Oryza sativa L.) seeds were sterilized for 30 min using 5% H2O2. Subsequently, the seeds were thoroughly rinsed using sterilized ultrapure water (18.2 Ω cm−1). Double-layer gauze was placed in a culture tray (30 × 40 cm). Ultrapure water was added to the tray to wet the gauze. In the culture tray, 150 seeds of the same diameter and shape were germinated. The culture tray was placed in a climate chamber at 3000 Lx irradiation, a temperature of 25 °C, and 80% humidity. Rice seedlings were obtained after five days of culturing. GO (purity >99%) was obtained from Suzhou OST Advanced Materials Co., Ltd, China. Five rice seedlings of uniform growth status were transferred into a 50 mL centrifuge tube filled with a PGO suspension (n = 20, 20 parallel treatments). The rice seedlings were fixed in the centrifuge tube using a sponge. To achieve a sufficient amount of LGO, the rice roots were exposed to a PGO suspension at 100 mg L−1 (5 mg of PGO was suspended in 50 mL of ultrapure water under ultrasonication for 10 min at 400 W). The hydroponic centrifuge tube was placed in a climate chamber that was maintained at 3000 Lx irradiation, a temperature of 25 °C, and 80% relative humidity. The interaction between the root exudates and the PGO was allowed to proceed for fifteen days. During this interaction, the system was supplemented with ultrapure water to maintain a volume of 40–50 mL. To reduce the precipitation of the PGO and to simulate simple turbulence, the PGO suspension was gently stirred three times per day at 150 rpm for 10 min. A centrifuge tube filled with ultrapure water and rice seedlings served as the exudate control group. After fifteen days, LGO was collected from the hydroponic suspension using a 0.1 μm filter. The LGO captured by the membrane was lyophilized to obtain an LGO powder. The separated aqueous phase and the obtained LGO powder were used for chemical property characterization and nanotoxicity analysis.
Identification of the root exudates on LGO
Using 1 mL of solution (with a volume ratio of methanol
:
chloroform
:
water = 2.5
:
1
:
1; methanol and chloroform: analytical reagent, water: 18.2 Ω cm−1) as a solvent, the exudates were extracted from 0.01 g of LGO. The exudates were intensively extracted using microwave-assisted extraction (at 40 °C for 15 min), followed by centrifugation for 10 min at 11
000g. The supernatant was collected. The sediment was extracted a second time using the same solvent and procedure described above. The second supernatant was mixed with the first supernatant. Water (500 μL) was added to the supernatant mixture, which was then centrifuged at 5000g for 3 min. The lower layer was evaporated under nitrogen flux. For the upper layer, the methanol was evaporated under nitrogen flux, and the water was then lyophilized. Methoxamine hydrochloride (20 mg mL−1, 50 μL) and N-methyl-N-(trimethylsilyl) trifluoroacetamide (80 μL) were used as derivatives. A sample (1 μL) was injected into a gas chromatography (GC) column in a split mode (1
:
5). GC (Agilent 6890N, Agilent Technologies, USA) combined with quadrupole mass spectrometry (MS) (Agilent 5973, Agilent Technologies, USA) was used to analyze the root exudates. GC separation was achieved on a DB-5 MS capillary column (30 m, 0.25 mm i.d., 0.1 μm film thickness). The injection temperature was 230 °C, and both the transfer line and the ion source were maintained at 250 °C. The spectrometer was operated in electron-impact mode. The detection voltage was 2100 V. The full scan range was from 60 to 800 amu. Helium, the carrier gas, was maintained at a constant flow rate of 2 mL min−1. The oven temperature was maintained at 80 °C for 2 min and was then increased to 320 °C at a rate of 15°C min−1 and held for 6 min. The root exudates were identified using the NIST 08 library.
Identification of the root exudates in the aqueous phase
The root exudates in the aqueous phase were extracted using ethyl acetate. Via liquid–liquid extraction, the ethyl acetate phase was collected from the aqueous phase using a separatory funnel. The collected ethyl acetate phase was filtered through a 0.22 μm membrane and concentrated to 1 mL using a rotary evaporator under vacuum (35 °C). The root exudates in the aqueous phase were detected via gas chromatography-mass spectrometry/mass spectrometry (GC-MS/MS) as described above.
Characterization of PGO and LGO
The structures and surface morphologies of PGO and LGO were characterized via scanning electron microscopy (SEM), field-emission transmission electron microscopy (TEM) and atomic force microscopy (AFM). The PGO and LGO were dispersed in absolute ethanol, and SEM, TEM and AFM observations were then acquired using an LEO-1530VP instrument, a JEM-2010 FEF instrument and a Veeco Nanoscope 4, respectively. The suspensions (1.0 mg L−1) of PGO and LGO were prepared in water for the analysis of zeta potential and size distribution. The size distribution and zeta potential of the nanosheets were measured using a ZETAPALS/BI-200SM instrument equipped with a 30 mW, 635 nm laser (Brookhaven Instruments Corporation, Holtsville, NY, USA). The surface chemistry was analyzed via X-ray photoelectron spectroscopy (XPS) and Fourier transform infrared spectroscopy (FTIR). XPS measurements were performed using an Axis Ultra XPS system (Kratos) with a monochromatic Al Kα X-ray source (1486.6 eV). The spectra were analyzed using a Casa-XPS V2.3.13 software. FTIR spectra were recorded with a Bruker Tensor 27 (Germany) infrared spectrometer with a resolution of 2 cm−1 at 4000–400 cm−1. Raman spectra were obtained using a Renishaw inVia Raman spectrometer (Renishaw plc, UK) with a 514 nm laser (Thermo Scientific, DXR). UV-vis spectra were collected using a TU-1901 spectrophotometer and were subsequently analyzed using UVWin5 software.
EPR measurements
To measure unpaired electrons, which are related to the chemical activity and toxicity of nanomaterials, electron paramagnetic resonance (EPR) was performed. All X-band EPR spectra were collected at room temperature (296 K) using a Magnettech MiniScope 400 EPR spectrometer operated at a microwave frequency of 9.4 GHz and a magnetic field modulation frequency of 100 kHz. The spectrometer was controlled using MiniScope Control software. 2,6,6-tetramethyl-1-piperidinyloxy (TEMPO) was employed as an unpaired electron scavenger. PGO and LGO at 0.01 mg L−1 were placed in individual quartz EPR tubes. Before the samples were loaded, the quartz EPR tubes were washed thoroughly using ultrapure water and then dried.
Hatching, malformation, and mortality in zebrafish
Developing zebrafish embryos (strain AB) were cultured in a 96-well plate in E3 medium (5 mM NaCl, 0.17 mM KCl, 0.33 mM CaCl2, 0.33 mM MgSO4; pH 7.4). The volume of E3 culture medium in each well was 200 μL with 0.01, 0.1, 1 and 10 mg L−1 PGO or LGO, respectively; the media were replaced every 24 h until 120 hours post-fertilization (hpf). Each trial was performed in triplicate, and 20 embryos were analyzed in each parallel sample. The emergence of juveniles from the chorion was considered to be a successful hatching. The juveniles were transferred into a solution of 0.016 M tricaine for anesthetization. The developing embryos and the juveniles were imaged, and the percentages of malformation (abnormal tail flexure and pericardial edema) were recorded on a light microscopy (Olympus ZL 61, Olympus, Tokyo). The mortality rates of the embryos and juveniles were determined by examining their movement, heartbeat and blood circulation using light microscopy (Olympus ZL 61, Olympus, Tokyo); data were recorded daily.
Mitochondrial membrane potential
The mitochondrial membrane potential was measured using the lipophilic cationic dye 5,5,6,6-tetrachloro-1,1,3,3-tetraethylbenzimidazolylcarbocyanine iodide (JC-1). This dye selectively enters mitochondria, and the color of the dye changes from red to green if the membrane potential decreases. After treatment, the embryos at 120 hpf were washed with E3 medium and incubated with 10 mM JC-1 dye for 15 min at 37 °C. The embryos were analyzed using an Olympus BX-41 light microscope equipped with an X-Cite 120Q excitation light source; the images were collected and processed using CellSens Standard 1.6 software. The double-excitation wavelengths were 475 nm and 520 nm.
All experiments were performed in compliance with the relevant laws and institutional guidelines, and approved by Animal Ethics Committee for the Use of Human or Animal Subjects of Nankai University.
Statistical analysis
All treatments were performed in triplicate unless otherwise specified in the figure and table legends. The error bars presented in the results represent standard deviations. Differences were regarded as statistically significant at the level of p < 0.05. Using IBM Statistics SPSS 19 software, the data were analyzed using a one-way analysis of variance (ANOVA) and were compared using a post hoc Tukey's test. A heat map was generated using a Multiple Experiment Viewer 4.8.1 software. The cluster analysis was conducted via hierarchical clustering (HCL) on a Multiple Experiment Viewer 4.8.1 software. The default distance metric for HCL was Pearson correlation, and the linkage method selection was average linkage clustering.
Acknowledgements
This work was financially supported by the Ministry of Education of China (Grant no. IRT 13024), the National Natural Science Foundation of China (Grant no. 31170473, U1133006, 21037002, 21307061 and 21407085), the Central Public Research Institutes Basic Funds for Research and Development (Agro-Environmental Protection Institute, Ministry of Agriculture, 2014-SZJJ-muli-03), the Doctoral Program of Higher Education of China (Grant no. 2013003112016), the Tianjin Natural Science Foundation (Grant no. 14JCQNJC08900), and the Postdoctoral Science Foundation of China (Grant no. 2014M550138).
Notes and references
- K. S. Novoselov, V. Fal, L. Colombo, P. Gellert, M. Schwab and K. Kim, Nature, 2012, 490, 192–200 CrossRef PubMed.
- R. Joshi, P. Carbone, F. Wang, V. Kravets, Y. Su, I. Grigorieva, H. Wu, A. Geim and R. Nair, Science, 2014, 343, 752–754 CrossRef PubMed.
- D. R. Dreyer, S. Park, C. W. Bielawski and R. S. Ruoff, Chem. Soc. Rev., 2010, 39, 228–240 RSC.
- F. Ahmed and D. F. Rodrigues, J. Hazard. Mater., 2013, 256, 33–39 CrossRef PubMed.
- X. Hu and Q. Zhou, Chem. Rev., 2013, 113, 3815–3835 CrossRef PubMed.
- J. Zhao, Z. Wang, J. C. White and B. Xing, Environ. Sci. Technol., 2014, 48, 9995–10009 CrossRef PubMed.
- N. A. Anjum, N. Singh, M. K. Singh, Z. A. Shah, A. C. Duarte, E. Pereira and I. Ahmad, J. Nanopart. Res., 2013, 15, 1–12 Search PubMed.
- N. A. Anjum, N. Singh, M. K. Singh, I. Sayeed, A. C. Duarte, E. Pereira and I. Ahmad, Sci. Total Environ., 2014, 472, 834–841 CrossRef PubMed.
- R. Nair, M. S. Mohamed, W. Gao, T. Maekawa, Y. Yoshida, P. M. Ajayan and D. S. Kumar, J. Nanosci. Nanotechnol., 2012, 12, 2212–2220 CrossRef PubMed.
- X. Qu, J. Brame, Q. Li and P. J. Alvarez, Acc. Chem. Res., 2012, 46, 834–843 CrossRef PubMed.
- P. J. Alvarez, V. Colvin, J. Lead and V. Stone, ACS Nano, 2009, 3, 1616–1619 CrossRef PubMed.
- J. Lee, S. Mahendra and P. J. Alvarez, ACS Nano, 2010, 4, 3580–3590 CrossRef PubMed.
- X. Hu and Q. Zhou, Sci. Rep., 2014, 4, 1–9 Search PubMed.
- X. Hu, L. Mu, J. Kang, K. Lu, R. Zhou and Q. Zhou, Environ. Sci. Technol., 2014, 6919–6927 CrossRef PubMed.
- T. Kuila, S. Bose, P. Khanra, A. K. Mishra, N. H. Kim and J. H. Lee, Carbon, 2012, 50, 914–921 CrossRef PubMed.
- O. Akhavan and E. Ghaderi, Carbon, 2012, 50, 1853–1860 CrossRef PubMed.
- G. Wang, F. Qian, C. W. Saltikov, Y. Jiao and Y. Li, Nano Res., 2011, 4, 563–570 CrossRef PubMed.
- E. C. Salas, Z. Sun, A. Luttge and J. M. Tour, ACS Nano, 2010, 4, 4852–4856 CrossRef PubMed.
- C. Zhu, Z. Feng, M. Fan, C. Chen, B. Ma, J. Yang and D. Sun, RSC Adv., 2014, 4, 40292–40295 RSC.
- P. A. Holden, R. M. Nisbet, H. S. Lenihan, R. J. Miller, G. N. Cherr, J. P. Schimel and J. L. Gardea-Torresdey, Acc. Chem. Res., 2012, 46, 813–822 CrossRef PubMed.
- P. A. Holden, F. Klaessig, R. F. Turco, J. H. Priester, C. M. Rico, H. Avila-Arias, M. Mortimer, K. Pacpaco and J. L. Gardea-Torresdey, Environ. Sci. Technol., 2014, 48, 10541–10551 CrossRef PubMed.
- C. M. Rico, S. Majumdar, M. Duarte-Gardea, J. R. Peralta-Videa and J. L. Gardea-Torresdey, J. Agric. Food Chem., 2011, 59, 3485–3498 CrossRef PubMed.
- L. Lyubenova, A. J. Kuhn, A. Höltkemeier and P. Schröder, Plant Soil, 2013, 370, 187–195 CrossRef.
- M. F. Quartacci, B. Irtelli, C. Gonnelli, R. Gabbrielli and F. Navari-Izzo, Environ. Pollut., 2009, 157, 2697–2703 CrossRef PubMed.
- W.-J. Jiang, Z.-J. Li, Z.-Y. Zhang, J. Zhang, T. Liu, M. Yu, Y.-L. Zhou and Z.-F. Chai, Acta Chim. Sin., 2008, 1740–1744 Search PubMed.
- G. H. LeFevre, R. M. Hozalski and P. J. Novak, Environ. Sci. Technol., 2013, 47, 11545–11553 CrossRef PubMed.
- Y. C. Huang, R. Fan, M. A. Grusak, J. D. Sherrier and C. Huang, Sci. Total Environ., 2014, 497, 78–90 CrossRef PubMed.
- U. Baetz and E. Martinoia, Trends Plant Sci., 2014, 19, 90–98 Search PubMed.
- M. A. Kiser, D. A. Ladner, K. D. Hristovski and P. K. Westerhoff, Environ. Sci. Technol., 2012, 46, 7046–7053 CrossRef PubMed.
- S. Kim, S. Zhou, Y. Hu, M. Acik, Y. J. Chabal, C. Berger, W. de Heer, A. Bongiorno and E. Riedo, Nat. Mater., 2012, 11, 544–549 CrossRef PubMed.
- H. Chang, Z. Sun, M. Saito, Q. Yuan, H. Zhang, J. Li, Z. Wang, T. Fujita, F. Ding and Z. Zheng, ACS Nano, 2013, 7, 6310–6320 CrossRef PubMed.
- S. C. Smith, F. Ahmed, K. M. Gutierrez and D. F. Rodrigues, Chem. Eng. J., 2014, 240, 147–154 CrossRef PubMed.
- B. Beless, H. S. Rifai and D. F. Rodrigues, Environ. Sci. Technol., 2014, 48, 10372–10379 CrossRef PubMed.
- W. Fan, Z. Shi, X. Yang, M. Cui, X. Wang, D. Zhang, H. Liu and L. Guo, Water Res., 2012, 46, 5981–5988 CrossRef PubMed.
- A. J. Martinolich, G. Park, M. Y. Nakamoto, R. E. Gate and K. E. Wheeler, Environ. Sci. Technol., 2012, 46, 6355–6362 CrossRef PubMed.
- L. Zhang, X. Li, Y. Huang, Y. Ma, X. Wan and Y. Chen, Carbon, 2010, 48, 2367–2371 CrossRef PubMed.
- Y. Li, R. Umer, Y. A. Samad, L. Zheng and K. Liao, Carbon, 2013, 55, 321–327 CrossRef PubMed.
- L. Wu, Y. Zhang, C. Zhang, X. Cui, S. Zhai, Y. Liu, C. Li, H. Zhu, G. Qu and G. Jiang, ACS Nano, 2014, 8, 2087–2099 CrossRef PubMed.
- K.-H. Liao, Y.-S. Lin, C. W. Macosko and C. L. Haynes, ACS Appl. Mater. Interfaces, 2011, 3, 2607–2615 Search PubMed.
- I. Shomer, A. J. Novacky, S. M. Pike, U. Yermiyahu and T. B. Kinraide, Plant Physiol., 2003, 133, 411–422 CrossRef PubMed.
- A. M. Dimiev and J. M. Tour, ACS Nano, 2014, 8, 3060–3068 CrossRef PubMed.
- Z.-H. Sheng, L. Shao, J.-J. Chen, W.-J. Bao, F.-B. Wang and X.-H. Xia, ACS Nano, 2011, 5, 4350–4358 CrossRef PubMed.
- Z. Yang, Z. Yao, G. Li, G. Fang, H. Nie, Z. Liu, X. Zhou, X. a. Chen and S. Huang, ACS Nano, 2011, 6, 205–211 CrossRef.
- S. Fischer, A. C. Papageorgiou, M. Marschall, J. Reichert, K. Diller, F. Klappenberger, F. Allegretti, A. Nefedov, C. Wöll and J. V. Barth, J. Phys. Chem. C, 2012, 116, 20356–20362 Search PubMed.
- K. Yang, Y. Li, X. Tan, R. Peng and Z. Liu, Small, 2013, 9, 1492–1503 CrossRef PubMed.
- S. George, S. Lin, Z. Ji, C. R. Thomas, L. Li, M. Mecklenburg, H. Meng, X. Wang, H. Zhang and T. Xia, ACS Nano, 2012, 6, 3745–3759 CrossRef PubMed.
- S. Rao, S. N. Jammalamadaka, A. Stesmans, V. Moshchalkov, J. v. Tol, D. Kosynkin, A. Higginbotham-Duque and J. Tour, Nano Lett., 2012, 12, 1210–1217 CrossRef PubMed.
- X. Hu, L. Mu, K. Lu, J. Kang and Q. Zhou, ACS Appl. Mater. Interfaces, 2014, 9220–9227 Search PubMed.
- H. Yin, H. Tang, D. Wang, Y. Gao and Z. Tang, ACS Nano, 2012, 6, 8288–8297 CrossRef PubMed.
- Z. Luo, Y. Lu, L. A. Somers and A. C. Johnson, J. Am. Chem. Soc., 2009, 131, 898–899 CrossRef PubMed.
- C. Zhu, S. Guo, Y. Fang and S. Dong, ACS Nano, 2010, 4, 2429–2437 CrossRef PubMed.
- J. Muller, F. Huaux, A. Fonseca, J. B. Nagy, N. Moreau, M. Delos, E. Raymundo-Piñero, F. Béguin, M. Kirsch-Volders and I. Fenoglio, Chem. Res. Toxicol., 2008, 21, 1698–1705 CrossRef PubMed.
- Q. Wang, S. Lee and H. Choi, J. Phys. Chem. C, 2010, 114, 2027–2033 Search PubMed.
- I. Fenoglio, G. Greco, M. Tomatis, J. Muller, E. Raymundo-Pinero, F. Béguin, A. Fonseca, J. B. Nagy, D. Lison and B. Fubini, Chem. Res. Toxicol., 2008, 21, 1690–1697 CrossRef PubMed.
- M. C. Stensberg, R. Madangopal, G. Yale, Q. Wei, H. Ochoa-Acuña, A. Wei, E. S. Mclamore, J. Rickus, D. M. Porterfield and M. S. Sepúlveda, Nanotoxicology, 2014, 8, 833–842 CrossRef PubMed.
- T. Buerki-Thurnherr, L. Xiao, L. Diener, O. Arslan, C. Hirsch, X. Maeder-Althaus, K. Grieder, B. Wampfler, S. Mathur and P. Wick, Nanotoxicology, 2013, 7, 402–416 CrossRef PubMed.
- J. Labille, J. Feng, C. Botta, D. Borschneck, M. Sammut, M. Cabie, M. Auffan, J. Rose and J.-Y. Bottero, Environ. Pollut., 2010, 158, 3482–3489 CrossRef PubMed.
Footnote |
† Electronic supplementary information (ESI) available: Fig. S1 presenting the wide spectra of XPS of PGO and LGO. Fig. S2 presenting the FTIR spectra of PGO and LGO. Tables S1–S3 listing the data of root exudates detected by GC-MS/MS. See DOI: 10.1039/c4ra16340k |
|
This journal is © The Royal Society of Chemistry 2015 |