DOI:
10.1039/C4RA11347K
(Paper)
RSC Adv., 2015,
5, 15778-15783
A novel coumarin derivative as a sensitive probe for tracing intracellular pH changes†
Received
27th September 2014
, Accepted 19th January 2015
First published on 19th January 2015
Abstract
A new pH sensitive probe, 7-diethylamino-3-[2-(4-piperidin-1-yl-phenyl)-vinyl]-chromen-2-one (CS-P), was designed and synthesized. This probe was constructed by introduction of piperidine to the coumarin stem. It exhibited high pH sensitivity ranging from pH 5.9 to pH 3.0 (pKa = 4.55), and in vitro cell imaging showed that its fluorescence intensity was distinctly related to the cellular pH changes induced by chloroquine or dexamethasone. Demonstrated as having good cell membrane permeability and high pH sensitivity, this probe is suitable for tracing the intracellular pH changes from neutral to acidic conditions.
Introduction
Intracellular pH plays key roles in modulating cell growth and differentiation, enzyme and tissue functions, such as cell cycle control,1 cellular proliferation and apoptosis,2 membrane potential and ion regulation,3 muscle contraction4 and multidrug resistance (MDR).5
Abnormal pH values are associated with inappropriate cell functions, growth, and division, which can cause many serious human diseases. An enhanced activity of Na(+)/Li(+) countertransport, studied as a surrogate of Na(+)/H(+) exchanger, has been described in red blood cells of patients with cardiac syndrome X.6 Mucolipidosis type IV (MLIV) is a neurodegenerative channelopathy and in MLIV the lysosomal pH is lower than normal.7 Eukaryotic cell contains some compartments with different acidity degrees, for example, the cytoplasma is slightly alkaline at about pH 7.2, whereas some organelles, such as lysosomes and endosomes, have intracompartmental pHs of 4.0–6.0.8 Lysosomes and endosomes which participate in the critical functions of endocytic and digestive processes have pH value of 4.7 and 5.5, respectively.9 Therefore, monitoring of pH changes in live cells is critical for studying cellular functions and further understanding physiological and pathological processes.
There are many popular techniques to measure intracellular pH, including microelectrodes, nuclear magnetic resonance (NMR), and molecular spectroscopy. Among these techniques, fluorescence spectroscopy has attracted much more attention due to its excellent sensitivity, fast response time, high signal-to-noise ratio and the ability to continuously monitor the rapid kinetic pH changes.10
Fluorescent probe offers a unique approach for visualizing morphological details of tissues with subcellular resolution and has become a powerful tool for manipulating and investigating living cells and even animals.11–14 There are different kinds of fluorescent probes with various skeleton structure, including rhodamine,15 BODIPY,16 fluorescein,17 tricarbocyanine,18 benzo[a]phenoxazine,19 coumarin20 etc. From these fluorophores, some special probes with pH sensitivity can be designed and synthesized by changing functional groups to these skeletons.
Coumarins are a group of good candidates, because they have very high fluorescence quantum yield,21 large stokes shift,22 excellent light stability, and low toxicity.23 At the same time, coumarin derivatives have expanded emission profiles tunable from the blue to near-infrared (NIR) region by simply changing donors at either the 6- or 7-position and the electron acceptor at either 3- or 4- position of the coumarin core structure.24 So far, based on the coumarin platform, a number of pH sensitive fluorescent probes with different functional groups had been constructed.25–27 However, connecting H+ binding sites to the coumarin skeleton by styrene bond for increasing conjugated system it has not been reported.
In this paper we developed a new coumarin derivative, termed 7-diethylamino-3-[2-(4-piperidin-1-yl-phenyl)-vinyl]-chromen-2-one (CS-P), and its photophysical properties and intracellular pH sensitivity were studied.
Experimental
Materials and methods
All chemicals and solvents were at analytical grade and freshly distilled prior to use without further purification, except spectrographically pure dimethylsulfoxide (DMSO) used for biological evaluation. Chloroquine was commercially available from Aldrich (St. Louis, MO) and used without further purification. Double distilled water was used for preparation of the buffer solutions. 1H NMR and 13C NMR spectra were measured by BrukerAvance AV II-400 MHz spectrometer. FT-IR spectra were recorded on a Perkin-Elmer 2000 infrared spectrometer with KBr pellets under an ambient atmosphere. UV-Vis absorption spectra were performed on a Perkin Elmer Lambda 950 UV/VIS spectrometer. Fluorescence spectra were collected on a PerkinElmer LS55 fluorescence spectrophotometer using excitation wavelength of 400 nm. The pH values were determined with PHS-3E pH Meter calibrated at room temperature (25 ± 2 °C) with standard buffers of pH 6.86 and 4.00. Acetic acid and sodium acetate were used for tuning pH values. Fluorescence microscopic images were obtained from High-speed Widefield Live-cell System (Leica AF7000).
Synthesis
The detailed synthetic routes were shown in Scheme 1. 4-Piperidin-1-yl-benzaldehyde28 and 7-diethylamino-chromen-2-one29 were synthesized according to the literature procedures.
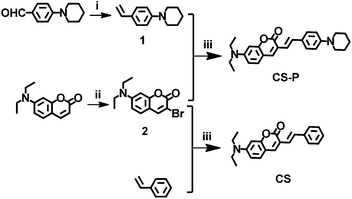 |
| Scheme 1 The synthetic routes of the objective compounds. (i) BuLi, Ph3PCH3I, THF, 0 °C; (ii) Br2, CH3COOH; (iii) Pd(OAC)2, P(O-toly)3, Et3N, DMF, 100 °C. | |
Synthesis of 1-(4-vinyl-phenyl)-piperidine (1). n-BuLi (3.2 mL of 2.5 M in n-hexane) was added dropwise to a stirred solution of Ph3PCH3I (3.2 g, 7.85 mmol) in THF (25 mL) at 0 °C under inert atmosphere. After stirring for 30 min, a solution of 4-piperidin-1-yl-benzaldehyde (5.23 mmol) in THF (10 mL) was added dropwise and the resulting mixture was stirred at room temperature for 10 h. Then, saturated ammonium chloride was added and followed by extracting with PE. The organic layer was washed with brine before being dried over anhydrous magnesium sulfate. After evaporating off the solvent, the residue was purified by column chromatography on silica gel to give a pure yellow solid (yield: 85.0%). 1H NMR (400 MHz, CDCl3) δ (ppm): 7.31 (d, J = 8.4 Hz, 2H, ArH), 6.89 (d, J = 8.4 Hz, 2H, ArH), 6.67–6.60 (m, 1H,
CH), 5.58 (d, J = 7.2 Hz, 1H,
CH2), 5.07 (d, J = 10.8 Hz, 1H,
CH2), 3.18 (t, J = 5.4 Hz, 4H, –CH2), 1.73–1.68 (m, 4H, –CH2), 1.61–1.58 (m, 2H, –CH2).
Synthesis of 3-bromo-7-diethylamino-chromen-2-one (2). Br2 (1.4 g, 9.2 mmol) was added dropwise to a solution of 7-diethylamino-chromen-2-one (2.0 g, 9.2 mmol) in acetic acid (40 mL), and the mixture was stirred at room temperature for 2 h. After completion of the reaction, the precipitate was filtered off and washed with acetic acid, then dried under vacuum and recrystallized in acetonitrile to afford a light yellow solid (yield: 80.6%). 1H NMR (400 MHz, DMSO-d6) δ (ppm): 8.34 (s, 1H, ArH), 7.44 (d, J = 8.8 Hz, 1H, ArH), 6.75 (dd, J = 8.8 Hz, J = 2.4 Hz, 1H, ArH), 6.56 (s, 1H, ArH), 3.45–3.41 (m, 4H, –CH2), 1.13 (t, J = 7.2 Hz, 6H, –CH3).
General procedure for the synthesis of target compound
2 (0.3 g, 1.01 mmol), appropriate styrene (1.1 eq.), tris(o-tolyl) phosphine (12.28 mg, 0.0404 mmol), palladium acetate (4.52 mg, 0.0202 mmol) and Et3N (1.97 mL, 14.14 mmol) were added in 24 mL of argon degassed DMF in a flask. After stirring at 100 °C for 24 h under argon, the mixture was cooled to room temperature and poured into water. Resulting solid was filtered off and washed with water, then dried. The crude product residue was purified by column chromatograph over silica to yield the pure product.
7-Diethylamino-3-styryl-chromen-2-one (CS). Orange red solid. Recrystallized from ethanol. Yield: 46.9%; 1H NMR (400 MHz, CDCl3) δ (ppm): 7.68 (s, 1H, ArH), 7.52 (d, J = 7.6 Hz, 2H, ArH), 7.47 (d, J = 16.0 Hz, 1H,
CH), 7.36 (t, J = 7.2 Hz, 2H, ArH), 7.30 (d, J = 8.8 Hz, 1H, ArH), 7.24 (d, J = 7.2 Hz, 1H, ArH), 7.11 (d, J = 16.0 Hz, 1H,
CH), 6.60 (dd, J = 8.8 Hz, J = 2.4 Hz, 1H, ArH), 6.51 (d, J = 2.4 Hz, 1H, ArH), 3.45–3.39 (m, 4H, –CH2), 1.23 (t, J = 6.8 Hz, 6H, –CH3). 13C NMR (100 MHz, CDCl3): δ (ppm) 161.5, 155.6, 150.4, 138.0, 137.6, 130.0, 128.8, 128.7, 127.6, 126.6, 123.1, 117.8, 109.1, 109.0, 97.2, 44.9, 12.5. IR (KBr), cm−1: 3070, 3021, 2975, 2920, 1704, 1616, 1587, 1523, 1238, 1139, 975, 750. MS (ESI)+: m/z 320.1644 (M + H)+; calcd for (M + H)+: 320.1651.
7-Diethylamino-3-[2-(4-piperidin-1-yl-phenyl)-vinyl]-chromen-2-one (CS-P). Yellow solid. Yield: 56.1%; 1H NMR (400 MHz, CDCl3) δ (ppm): 7.63 (s, 1H, ArH), 7.42 (d, J = 8.4 Hz, 2H, ArH), 7.36 (d, J = 16.0 Hz, 1H,
CH), 7.28 (d, J = 8.0 Hz, 1H, ArH), 6.97 (d, J = 16.0 Hz, 1H,
CH), 6.90 (d, J = 8.4 Hz, 2H, ArH), 6.59 (dd, J = 8.8 Hz, J = 2.0 Hz, 1H, ArH), 6.51 (d, J = 1.6 Hz, 1H, ArH), 3.44–3.38 (m, 4H, –CH2), 3.22 (t, J = 5.2 Hz, 4H, –CH2), 1.73–1.67 (m, 4H, –CH2), 1.62–1.57 (m, 2H, –CH2), 1.23 (t, J = 7.2 Hz, 6H, –CH3). 13C NMR (100 MHz, CDCl3): δ (ppm) 161.7, 155.3, 151.5, 150.0, 136.3, 129.9, 128.5, 128.2, 127.6, 119.6, 118.6, 115.9, 109.3, 109.0, 97.2, 50.1, 44.8, 29.7, 25.7, 24.4, 12.5. IR (KBr), cm−1: 2971, 2925, 2849, 1704, 1594, 1511, 1232, 1128, 810. MS (ESI)+: m/z 403.2383 (M + H)+; calcd for (M + H)+: 403.2386.
Solvatochromic effects of CS and CS-P
CS, CS-P (8 μM) were prepared in cyclohexane, toluene, tetrahydrofuran, acetonitrile or dimethyl sulfoxide, respectively. Absorption spectra and fluorescence emission spectra of CS, CS-P in different solvents were recorded (λex = 400 nm).
Fluorescence changes of CS-P under different pH values
CS and CS-P (4 μM) were prepared in DMSO–water (1
:
1, v/v) buffer solution. These solutions were modulated by acetic acid and sodium acetate to achieve different pH values (3.0–7.6) with maintaining Na+ concentration of 0.1 mol L−1. After the solutions were kept at ambient temperature for 1–2 h, the fluorescence emission spectra were recorded (λex = 400 nm).
The constant pKa of CS-P was calculated based on the following formula, Fmin and Fmax represented the fluorescence intensity at minimal and maximal H+, respectively, and n meant the stoichiometry of H+ binding to the probe.
In addition, absorption spectra of CS-P (4 μM) in a DMSO–water solution (1
:
1, v/v) with different pH values were recorded.
The selectivity of CS-P for H+
Considering that nitrogen and oxygen could bind many metal ions in solution, it was very important to determine whether other ions were potential interferents to CS-P. The metal ions and biologically relevant analytes, Zn(NO3)2, MgSO4, NaCl, MnSO4, BaCl2, KCl, CaCl2, CuSO4, FeCl3, Cr(NO3)3, FeSO4, NiSO4, HgCl2, Pb(NO3)2, L-cystine and reduced glutathione, with the same concentration of 40 μM, were added as interferents to the solution (DMSO
:
H2O = 1
:
1, v/v) of CS-P (ultimate concentration: 4 μM). Fluorescence emission spectra of these solutions were recorded with excitation at 400 nm.
Tracing intracellular pH changes
Mouse macrophages Raw 264.7 cells were cultured in 24-well plate in this paper and were internalized with 2 μM CS-P for 15 min at 37 °C, then washed three times with PBS buffer to remove excess probe, chloroquine (100 μM) was added to stimulate lysosomal pH change based on the reference.15 Cells were observed under High-speed Widefield Live-cell System (Leica AF7000) from 0 minute to 60 minute with a time interval of 3 minutes.
HeLa cells were co-cultured with CS-P (2 μM) in 24-well plate for 15 min, then washed with fresh DMEM medium and dexamethasone (2 μM) was added to decreasing the cellular pH. Cells were observed and recorded by High-speed Widefield Live-cell System (Leica AF7000) from 0 minute to 60 minute with a time interval of 3 minutes.
Furthermore, in order to determine the cellular location of CS-P, MG63 cells were co-stained with LysoTracker® Red (0.1 μM) (L7528, Invitrogen) or MitoTracker® Deep Red (0.1 μM) (M22426, Invitrogen), respectively.
Results and discussion
Synthesis
The new coumarin derivative, CS-P, was synthesized from 1-(4-vinyl-phenyl)-piperidine and 3-bromo-7-diethylamino-chromen-2-one shown in Scheme 1. Their structures were confirmed by 1H NMR, 13C NMR. 7-Diethylamino-3-styryl-chromen-2-one (CS) was synthesized and used as control to reveal the primary mechanism of fluorescence changes of CS-P in the different pH condition, the structure difference between CS and CS-P was that CS-P was constructed by piperidine group on the stem of coumarin. Piperidine derivatives were effective proton receptors with different pKa values depending on the skeletons and position of substituents on the piperdine group.30,31
Solvatochromic effects
Solvatochromism of CS, CS-P were investigated in several solvents. The photophysical properties of CS, CS-P were compiled in Table S1.† The absorption spectra and the fluorescence emission spectra of CS and CS-P in various solvents were shown in Fig. S1 and S2,† respectively. The λabs(max) and λem(max) values of CS-P were strongly affected by solvent polarity. These results showed that piperidine, the functional group on the CS-P, aroused of a bathochromic shift of 25–40 nm, compared with CS. A large stokes shift (>75 nm) and solvent-dependent emission spectra indicated that effective intramolecular charge transfer (ICT) had occurred in the excited state between the coumarin unit and the substituent at position 3.
Spectral studies of CS-P
The absorption spectra, excitation spectra and the fluorescence spectra of CS-P at different pH values were shown in Fig. S3(A), S3(C)† and 1(A), respectively. Along with decreasing of the pH value from 7.6–3.0, the absorbance was significantly enhanced at 443 nm. In the meanwhile, the color of the solution changed from dark yellow to bright yellow-green, which indicated that CS-P could serve as a visual indicator for H+ concentration (Fig. S3(B)†). Fig. 1(A) showed that only very weak fluorescence intensity was observed in pH = 5.9–7.6 solution. However, the fluorescence intensity was significantly enhanced with pH values decreased from pH 5.9 to 3.0. The intensity of CS-P in pH 3.0 showed more than 47 fold higher compared with in pH 5.9, while the fluorescent intensity of the control compound, CS, did not changed in different pH values (Fig. S4.†). The analysis of emission intensities changed at 515 nm as a function of pH obtained a pKa of 4.55 (Fig. 1(B)), the pKa value almost matched with the typical pH value of the acidic organelles (such as lysosomes and endosomes), which indicated that CS-P could be suitable for monitoring pH variations in the acidic environments (pH 4–6) in vitro.
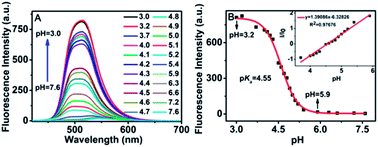 |
| Fig. 1 (A) Fluorescence spectra of CS-P (4 μM) in various pH (7.6–3.0) in DMSO–water (1 : 1, v/v) solution (λex = 400 nm). (B) Sigmoidal fitting of the pH-dependent fluorescence intensity at 515 nm. Inset: the good linearity in the pH range of 3.2–5.9. | |
The switching mechanism
CS-P and its protonated form of HCl were prepared to study the proton-binding behavior by 1H NMR spectroscopy. As shown in Fig. 2(A), Hb and Hc displayed a slight downfield shift of 0.05 and 0.04 ppm, respectively, suggesting that the diethylamine on the CS-P did not bind H+. However Ha displayed significant downfield shifts of 0.74 ppm, indicating that the protonation occurred at the piperidine site. The possible mechanism of pH sensitivity of CS-P was illustrated in Fig. 2(B). Under neutral pH condition (pH = 7.2), CS-P existed intramolecular charge transfer (ICT) process from piperidine part to coumarin matrix and weak fluorescence was due to strongly affected by solvent polarity. In contrast, under acidic pH condition (pH = 4.3), the nitrogen atom of piperidine group was protonated, which inhibited the ICT process and led to fluorescence enhancement.
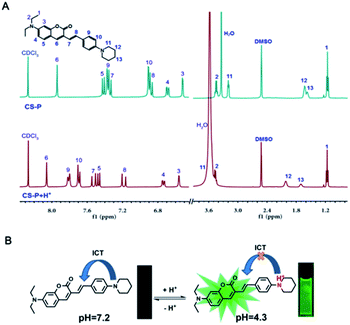 |
| Fig. 2 (A) 1H NMR spectra of CS-P and CS-P HCl in (CD3)2SO–CDCl3 (4 : 1, v/v), (B) mechanism of pH response by protonation and deprotonation of CS-P. | |
Testing of the selectivity, reversibility and photostability
Considering that nitrogen and oxygen could bind many metal ions in solution, it was very important to determine whether other ions were potential interferents. Upon addition of Na+, K+, Mg2+, Ca2+, Ba2+, Mn2+, Cr3+, Ni2+, Cu2+, Pb2+, Zn2+, Fe3+ and Fe2+ to the 4 μM CS-P solutions while keeping the experimental condition unchanged at pH 7.2, the results (Fig. 3) showed these cations did not increase the fluorescent intensity of CS-P. Moreover, amino acids (Cys and GSH) also exhibited negligible effects on the activity of CS-P, only a certain amount of H+ could lead to remarkable fluorescence enhancement. The competition experiments of CS-P revealed that the H+-depended fluorescence response was unaffected in the presence of background ions (Fig. S5†). The fluorescent intensity of CS-P could be reversibly selected by protonation/deprotonation of the piperidine moiety under acidic or alkaline condition (Fig. S6†). In addition, it exhibited high photostability (Fig. S7†). The high selectivity of CS-P over interferents, along with its photostability and reversibility, indicated CS-P had considerable potential as practical pH probe.
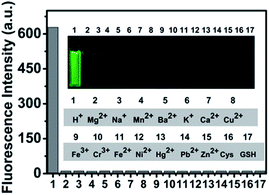 |
| Fig. 3 Fluorescence response (515 nm) of CS-P interfered by different ions and amino acids in DMSO–water (1 : 1, v/v) solution. | |
Tracing intracellular pH changes
The cytotoxicity of CS-P was evaluated by the MTT assays. CS-P showed no cytotoxicity (Fig. S8†).
In vitro cell imaging was carried by employing CS-P to monitor intracellular pH changes induced by chloroquine in Raw 264.7 cells. Chloroquine could increase intracellular pH in macrophage cells.32 CS-P exhibited strong yellow fluorescence in these cells before treated with chloroquine, the fluorescence intensities reduced distinctly in the cells by addition of chloroquine according to continuous observation within 60 min (Fig. 4).
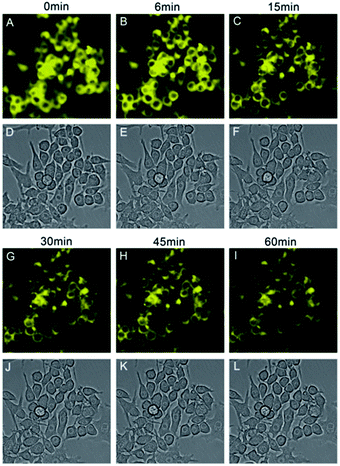 |
| Fig. 4 Fluorescent intensities (yellow) were decreased distinctly along with pH changes induced by chloroquine for mouse macrophage Raw 264.7. | |
Dexamethasone could induce cell apoptosis and intracellular acidification was happened spontaneously.15 After treatment with dexamethasone, the yellow fluorescence intensities in Hela cells increased gradually which indicated that the intracellular pH (especially these areas adjacent to the cell nuclei) became more and more acidic. Fig. 5 showed that the addition of dexamethasone caused apoptosis of Hela cells and time-dependent increasing of fluorescence intensity, which in accordance with previous report on the apoptosis process and the pH changes in HeLa cells.26 These results suggested that intracellular acidifications might be possibly due to the release of lysosomal proton in the apoptosis process. The increasing of fluorescence intensity in HeLa cells induced by dexamethasone was coincided with the results observed from RAW264.7 cells induced by chloroquine.
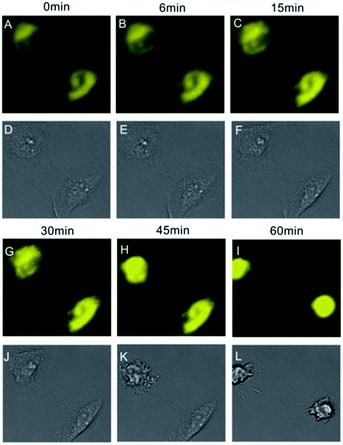 |
| Fig. 5 Fluorescent intensities of CS-P (yellow) were increased gradually along with pH changes in HeLa cells induced by dexamethasone which was caused to apoptosis status. | |
The pH value of cells would be changed in many cases, such as cancer and Alzheimer's disease,33 weakly basic substances can cause the leakage of protons out of lysosomal in the form of protonated bases,32 while a parts of compounds could decreased the pH value.15 However it was difficult to determine the intracellular pH changes in a single cell. CS-P proved a potential way to realize the detection of the cell pH changes.
CS-P showed high pH sensitive ranged from pH 5.9 to pH 3.0, which was suitable for tracing the intracellular pH changes. Furthermore, in order to determine the intracellular organelle distribution of CS-P (2 μM), we co-stained the MG63 cells with LysoTracker® Red (0.1 μM) (L7528, Invitrogen) or MitoTracker® Deep Red, respectively. The results were shown in Fig. 6, the yellow fluorescence was observed in cytoplasm (Fig. 6(A) and (D)), but in the merged figure, CS-P did not existed in lysosome (Fig. 6(C)) or mitochondria (Fig. 6(F)). These results proved that CS-P was localized in cytoplasm.
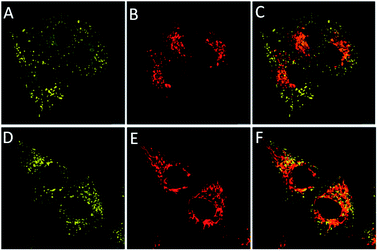 |
| Fig. 6 Cellular location experiment of CS-P. (A and D): Yellow emission for CS-P; (B) red emission for LysoTracker® Red; (E) MitoTracker® Deep Red. (C) Merged A and B. (F) Merged C and D. | |
Conclusions
In summary, a new coumarin derivative as pH sensitive probe was designed and synthesized. CS-P exhibited high pH sensitive ranged from pH 5.9 to pH 3.0 and its pKa = 4.55. In vitro cell culture results showed that the fluorescence intensity of CS-P could indicate the cellular pH changes distinctly. Furthermore, CS-P was localized in cytoplasm after entered in cells. These preliminary works demonstrated that CS-P was a promising candidate for tracing cellular pH changes.
Acknowledgements
The authors acknowledge the financial support for this work by Research Fund for the Doctoral Program of Higher Education of China (20130181110089), the National Natural Science Foundation of China (NSFC) (Grants no. 21372168) and National Key Technology R&D Program (2012BAI42G01).
Notes and references
- N. Grandin and M. Charbonneau, J. Cell Sci., 1991, 99, 5 CAS.
- S. Matsuyama and J. C. Reed, Cell Death Differ., 2000, 7, 1155 CrossRef CAS PubMed.
- D. G. Nicholls and I. D. Scott, Biochem. J., 1980, 186, 833 CAS.
- H. Karaki, S. Kitajima and H. Ozaki, Jpn. J. Clin. Med., 1992, 50, 2106 CAS.
- A. J. Janecki, M. H. Montrose, P. Zimniak, A. Zweibaum, C. M. Tse and S. Khurana, J. Biol. Chem., 1998, 273, 8790 CrossRef CAS PubMed.
- E. De Candia, G. A. Lanza, E. Romagnoli, G. Ciabattoni, A. Sestito and P. Pasqualetti, Int. J. Cardiol., 2005, 100, 371 CrossRef PubMed.
- A. Kogot-Levin, M. Zeigler, A. Ornoy and G. Bach, Pediatr. Res., 2009, 65, 686 CrossRef CAS PubMed.
- I. N. Rich, I. Brackmann, D. Worthington-White and M. J. Dewey, J. Cell. Physiol., 1998, 177, 109 CrossRef CAS.
- J. R. Casey, S. Grinstein and J. Orlowski, Nat. Rev. Mol. Cell Biol., 2010, 11, 50 CrossRef CAS PubMed.
- J. Han and K. Burgess, Chem. Rev., 2010, 110, 2709 CrossRef CAS PubMed.
- L. Yuan, W. Y. Lin, L. Tan, K. B. Zheng and W. M. Huang, Angew. Chem., Int. Ed., 2013, 52, 1628 CrossRef CAS PubMed.
- Y. M. Xiang, B. Y. He, X. F. Li and Q. Zhu, RSC Adv., 2013, 3, 4876 RSC.
- K. M. Wang, J. Huang, X. H. Yang, X. X. He and J. B. Liu, Analyst, 2013, 138, 62 RSC.
- T. Terai and T. Nagano, Eur. J. Physiol., 2013, 465, 347 CrossRef CAS PubMed.
- H. Zhu, J. Fan, Q. Xu, H. Li, J. Wang and P. Gao, Chem. Commun., 2012, 48, 11766 RSC.
- J. Han, A. Loudet, R. Barhoumi, R. C. Burghardt and K. Burgess, J. Am. Chem. Soc., 2009, 131, 1642 CrossRef CAS PubMed.
- Y. H. Chan, C. Wu, F. Ye, Y. Jin, P. B. Smith and D. T. Chiu, Anal. Chem., 2011, 83, 1448 CrossRef CAS PubMed.
- T. Myochin, K. Kiyose, K. Hanaoka, H. Kojima, T. Terai and T. Nagano, J. Am. Chem. Soc., 2011, 133, 3401 CrossRef CAS PubMed.
-
(a) R. Sun, W. Liu, Y. J. Xu, J. M. Lu, J. F. Ge and M. Ihara, Chem. Commun., 2013, 49, 10709 RSC;
(b) W. Liu, R. Sun, J. F. Ge, Y. J. Xu, Y. Xu, J. M. Lu, I. Itoh and M. Ihara, Anal. Chem., 2013, 85, 7419 CrossRef CAS PubMed.
- M. Lee, N. G. Gubernator, D. Sulzer and D. Sames, J. Am. Chem. Soc., 2010, 132, 8828 CrossRef CAS PubMed.
- E. Herz, T. Marchincin, L. Connelly, D. Bonner, A. Burns and S. Switalski, J. Fluoresc., 2010, 20, 67 CrossRef CAS PubMed.
- H. E. Katerinopoulos, Curr. Pharm. Des., 2004, 10, 3835 CrossRef CAS.
- A. O. Uwaifo, J. Toxicol. Environ. Health, 1984, 13, 521 CrossRef CAS PubMed.
- S. S. Zhu, W. Y. Lin and L. Yuan, Dyes Pigm., 2013, 99, 465 CrossRef CAS PubMed.
- H. S. Peng, J. A. Stolwijk, L. N. Sun, J. Wegener and O. S. Wolfbeis, Angew. Chem., Int. Ed., 2010, 49, 4246 CrossRef CAS PubMed.
- X. F. Zhou, F. Y. Su, H. G. Lu, P. Senechal-Willis, Y. Q. Tian and R. H. Johnson, Biomaterials, 2012, 33, 171 CrossRef CAS PubMed.
- X. D. Liu, Y. Xu, R. Sun, Y. J. Xu, J. M. Lu and J. F. Ge, Analyst, 2013, 138, 6542 RSC.
- P. Magdolen, M. Meciarova and S. Toma, Tetrahedron, 2001, 57, 4781 CrossRef CAS.
- D. Ray and P. K. Bharadwaj, Inorg. Chem., 2008, 47, 2252 CrossRef CAS PubMed.
- X. P. Chen, J. S. Jin, N. N. Wang, P. Lu and Y. G. Wang, Eur. J. Org. Chem., 2012, 824 CrossRef CAS.
- A. K. Leslie, D. D. Li and K. Koide, J. Org. Chem., 2011, 76, 6860 CrossRef CAS PubMed.
- B. Poole and S. Ohkuma, J. Cell Biol., 1981, 90, 665 CrossRef CAS.
- D. Ibarreta, E. Urcelay, R. Parrilla and M. S. Ayuso, Ann. Neurol., 1998, 44, 216 CrossRef CAS PubMed.
Footnotes |
† Electronic supplementary information (ESI) available. See DOI: 10.1039/c4ra11347k |
‡ These two authors contributed equally to this work. |
|
This journal is © The Royal Society of Chemistry 2015 |