DOI:
10.1039/C4QI00169A
(Research Article)
Inorg. Chem. Front., 2015,
2, 254-262
Two new members of the niobium-substituted polytungstophosphate family based on hexalacunary [H2P2W12O48]12− building blocks†
Received
18th October 2014
, Accepted 17th December 2014
First published on 26th December 2014
Abstract
Two structurally novel nanoscale clusters [{Nb6(O2)4P2W12O57}2]20− (1) and [{P2W12Nb7O63(H2O)2}4{Nb4O4(OH)6}]30− (2) were synthesized under specific reaction conditions. Cluster 1 displays an unprecedented di-Nb–O–Nb-linked Wells–Dawson dimer, whilst cluster 2 contains the highest nuclearity niobium containing heteropolyoxometalate to date. Furthermore, 31P and 183W NMR spectra indicate that the polyanion 2 remains stable in solution. The photocatalytic activities of the respective salts 1a, 2a and K6[α-P2W18O62]·14H2O for H2 evolution were evaluated. The enhanced photocatalytic activity of 2a may be attributed to the incorporation of Nb into the polyoxotungstate framework.
Introduction
Polyoxometalates (POMs)1 are an exceptional family of metal–oxo clusters, primarily constructed of Mo, W, V and Nb in their high oxidation states.2 This class of compounds has attracted great attention during the last two decades, not only due to their intriguing structural diversity3 and remarkable chemical properties,4 but also due to their various potential applications ranging from catalysis, magnetism, biomedicine, and materials science, to nanotechnology.5 The sustained interest in POM chemistry is reflected by the near exponential increase in the number of publications pertaining to new POM species over the last decade.2–5
Among the vast POM family, transition-metal-substituted POMs constitute the most expanding subfield, chiefly due to well-established synthetic strategies, which utilize various lacunary POM species as secondary building units to construct target compounds with various properties.6 In particular, the hexalacunary [H2P2W12O48]12− (P2W12) fragment has shown tremendous potential for the assembly of larger transition-metal-substituted POMs.7–11 To date, a considerable number of polyoxotungstate architectures have been synthesized, such as the monomeric, dimeric and tetrameric aggregates reported by Gouzerh and co-workers,7a,b and the P2W12-based trimeric family prepared by Wang and co-workers.8a,9b Fang and co-workers also recently communicated a spectacular structure, which can be described as the assembly of 16 Wells–Dawson subunits.8c A comprehensive literature survey of transition-metal-substituted POMs synthesized from P2W12 precursors is shown in Table 1.
Table 1 Survey of coordination chemistry of the hexavacant tungstophosphate
Polyanion |
The nuclearity of Ma |
Reference |
The number of transition metal ions encapsulated within the polyanion structure.
|
Iron-substituted derivatives |
[H4P2W12Fe9O56(OAc)7]6− |
9 |
Gouzerh et al. (2005)7a |
[H55P8W49Fe27O248]26− |
27 |
Gouzerh et al. (2005)7a |
[H12P4W28Fe8O120]16− |
8 |
Gouzerh et al. (2005)7b |
[(H2Fe2P2W16O60)3]24− |
6 |
Cronin et al. (2010)7c |
Manganese-substituted derivatives |
[{Mn(H2O)4}2{WO2(H2O)2}2{WO(H2O)}3(P2W12O48)3]22− |
2 |
Wang et al. (2008)8a |
[Ce3Mn2O6(OAc)6(H2O)9]2[Mn2P2W16O60]320− |
6 |
Wang et al. (2009)8b |
[Mn40P32W224O888]144− |
40 |
Fang, Kögerler et al. (2011)8c |
Cobalt-substituted derivatives |
[{W2Co2O8(H2O)2}(P2W12O46)2]20− |
2 |
Wang et al. (2008)9a |
[{Co(H2O)4}6{WO(H2O)}3(P2W12O48)3]18− |
6 |
Wang et al. (2010)9b |
[(Co2P2W16O60)3]24− |
6 |
Cronin et al. (2009)9c |
Nickel-substituted derivatives |
[(Ni2P2W16O60)3]30− |
6 |
Cronin et al. (2010)7c |
[{Ni3.5(H2O)13}{WO2(H2O)2}2{WO(H2O)}3(P2W12O48)3]18.75− |
3.5 |
Wang et al. (2008)8a |
[{Ni(H2O)4}6{WO(H2O)}3(P2W12O48)3]18− |
6 |
Wang et al. (2010)9b |
Copper-substituted derivative |
[{Cu3(H2O)9}{WO2(H2O)2}2{WO(H2O)}3(P2W12O48)3]20− |
3 |
Wang et al. (2008)8a |
Uranium-substituted derivative |
[Li(H2O)K4(H2O)3{(UO2)4(O2)4(H2O)2}2(PO3OH)2P6W36O136]25− |
4 |
Kortz et al. (2008)10 |
Niobium-substituted derivatives |
[P2W12O56(NbO2)6]12− |
6 |
Hill et al. (1997)11a |
[H6P2W12Nb4O59(NbO2)2]28− |
12 |
Yue et al. (2014)11b |
[{Nb4O6(OH)4}{Nb6P2W12O61}4]36− |
28 |
Niu et al. (2014)11c |
In contrast to the rapid development of reported 3d transition metal containing species, the synthesis and characterisation of niobium-substituted polyoxotungstates remains relatively unexplored,11–19 with the first Nb-substituted POM obtained in 1984.12 The substitution of WVI in polyoxotungstates with NbV is of particular interest, because of the expected enhanced basicity and nucleophilicity of the Nb-bound oxygen atoms, as well as the higher reactivity of the resulting polyanion.20 We have already demonstrated that niobium atoms incorporated in P2W12 are reactive sites with a strong tendency towards oligomer formation through Nb–O–Nb bonds,11c as seen in the Keggin type analogues.13–15
Herein, we present an important expansion of this family with two Nb-containing tungstophosphates, the dimeric Nb12-containing 24-tungsto-4-phosphate [H13{Nb6(O2)4P2W12O57}2]7− (1) and the tetrameric Nb32-containing 48-tungsto-8-phosphate [H14{P2W12Nb7O63(H2O)2}4{Nb4O4(OH)6}]16− (2), which were isolated as the salts K7[H13{Nb6(O2)4P2W12O57}2]·31H2O (1a) and (NH4)16[H14{P2W12Nb7O63(H2O)2}4{Nb4O4(OH)6}]·16H2O (2a), respectively. Furthermore, preliminary photocatalytic properties indicated that both compounds 1a and 2a exhibit significantly enhanced photocatalytic activity compared to similar structures.
Results and discussion
Synthesis
Typically, lacunary POMs are inorganic ligands derived from parent plenary structures, and can effectively bind transition metals with large range of nuclearities and diverse structural topologies currently realised.21 Incorporation of transition metal ions into lacunary cluster sites is a well-performed strategy for the construction of macromolecular POM assemblies.
In this context, multi-vacant Wells–Dawson polyoxoanions are of particular interest, as they possess vacant sites that could capture more transition metal ions and link them together to form large molecular architectures.7–11,22 It is worth noting that the monomeric, peroxo-niobium containing 12-tungsto-2-phosphate [(NbO2)6P2W12O56]12− (abbreviated as {(NbO2)6P2W12}), reported first by Hill and coworkers as early as 1997, has received little attention.11a To date, there is only one dimeric derivative reported, in which a {Nb12} assembly is wrapped by two P2W12 units.11b Recently, a direct method using a new one-pot synthetic strategy, enabled the study of the [Nb6O19]8− interaction with P2W12, which resulted in a tetrameric Nb28-containing polyanion.11c This indicated that it is possible to extend and grow this family following a systematic control of a combination of components, such as pH and temperature, to obtain large architectures.
The synthetic procedures for 1 and 2 are simple, and both compounds could be formed by carefully controlling the reaction parameters. It is well known that P2W12 is a metastable precursor that easily transforms in aqueous solution to other Wells–Dawson derivatives,7b,c,8b,9a,c,23 however, after insertion of six Nb ions, a more stable subunit is formed, which is evident in both 1 and 2.
An interesting aspect in the molecular growth of 1 and 2 is the possible formation of {(NbO2)xNb6−xP2W12} in situ, in which the oxoniobium(V) surface is more basic and reactive than its oxotungsten(VI) counterpart. If so, the NbV-substituted Wells–Dawson species serves as a likely starting point of aggregation, which is seldom for plenary POM structures.8c To rationalize the molecular growth, we postulate three concurrent reactions central to the formation of 1 and 2 under the selected reaction conditions (Fig. 1):
(1) The decomposition of [Nb6O19]8− in H2O2 aqueous solution, resulting in the release of intermediate {NbO2} species.
(2) The incorporation of {NbO2} into P2W12 at room temperature, and the formation of the peroxo precursor {(NbO2)6P2W12}.11a
(3) The stepwise cleavage of the O−O bonds followed by condensation to Nb–O–Nb bridges in acid solution on heating, which stabilizes these highly charged species.
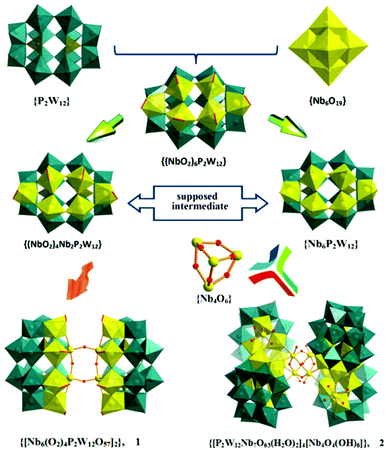 |
| Fig. 1 Postulated steps in the formation of 1 and 2. | |
The balanced chemical reaction for the formation of 1 and 2 is presented in eqn (1) and (2), respectively.
| 2[H2P2W12O48]12− + 2[Nb6O19]8− + 12H2O2 + 28H+ → [{Nb6(O2)4P2W12O57}2]20− + 28H2O | (1) |
| 12[H2P2W12O48]12− + 16[Nb6O19]8− + 96H2O2 + 350H+ → 3[{P2W12Nb7O63(H2O)2}4{Nb4O4(OH)6}]30− + 262H2O | (2) |
It is worth noting that the color of the starting solution turns from bright-yellow to light-yellow and finally colorless upon heating, suggesting the thermal decomposition of the peroxo groups. As expected, the reaction at 70 °C for 6 h gave a pale yellow solution with 1 as the major product, and 2 could be obtained with prolonged heating at 80 °C until the solution turned colorless. Moreover, the lower the pH, the shorter the required heating time. If the reaction is performed at pH 2.0, it takes about 36 h to turn colorless, whereas this can be done in about just 10 h when the pH value is 1.7. This demonstrates that small changes in the synthetic conditions can have considerable effect on the formation reaction thermodynamics of the product.
Structure of the polyanions
The structure of 1 (Fig. 2) comprises two hexalacunary P2W12 fragments with the vacant sites occupied by four peroxo-terminal {NbO2} groups (yellow polyhedra) and two oxo-bridging {NbO} groups (yellow balls), resulting in an assembly with idealized C2v symmetry. Each of the four peroxo-Nb atoms is coordinated by four μ2-oxo, one μ4-oxo (cap sites) or μ3-oxo (belt sites), and one terminal η2-coordinated peroxo ligand, leading to a distorted pentagonal-bipyramidal coordination sphere, whereas the two oxo-Nb atoms exhibit octahedral coordination. The two half-units [Nb6(O2)4P2W12O57]10− (Fig. S1†) are hence fused via the two parallel, equatorial Nb–O–Nb bridges (Fig. S2†), and are related by a mirror plane.
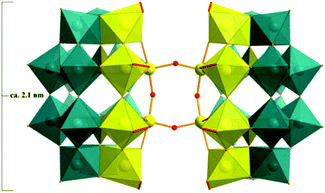 |
| Fig. 2 Combined polyhedral/ball-and-stick representation of polyanion 1. All cations and crystal waters are omitted for clarity. Teal polyhedra {WO6}; yellow polyhedra {Nb(O2)O5}; yellow balls Nb; dark-yellow polyhedra {PO4}; red balls O. | |
The structure of 1 suggests that the peroxo groups in the belt sites of the all-peroxo precursor {(NbO2)6P2W12} are preferentially reduced to hydroxo groups with respect to the cap sites, which then initiates dimerization.
Such dimerization through Nb–O–Nb bridges is known for the Nb-substituted Keggin anions (Fig. 3a).12,13a,c,14a As mentioned above, only one di-Nb–O–Nb-linked Wells–Dawson-dimer, [{H6P2W12Nb4O59(NbO2)2}2]8− (1′) has so far been reported very recently, with the peroxo groups ‘trans’ to each other (Fig. 3b).11b We now present a different di-Nb–O–Nb-bridged Wells–Dawson-dimer with ‘cis’ peroxo groups (1), which represents a previously unreported structure type. This cis-dimeric configuration seems especially relevant from a mechanistic point of view, providing a second perspective on the possible Nb–O–Nb linkage growth, and leading to further aggregation of more subunits with the Nb atoms from the equatorial sites, as in the case of polyanion 2.
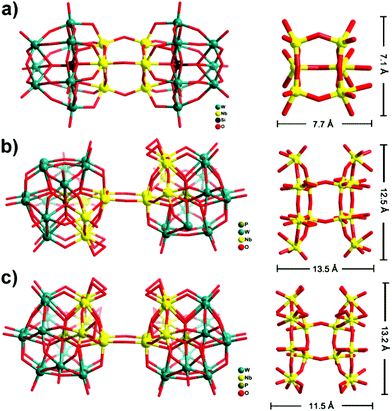 |
| Fig. 3 Comparison of the Nb–O–Nb bridges to polyanions 1 and 1′ and the Keggin-based niobium-substituted polyanions. (a) The ‘double Keggin’ structure of [Si2Nb6W18O77]8−, in which two {Nb3SiW9O40} subunits are linked through three Nb–O–Nb bridges.13c In the case of the ‘double Wells–Dawson’ structure, it can clearly be seen how the {Nb6(O2)2P2W12O59}11b or {Nb6(O2)4P2W12O57} subunits are fused together by two Nb–O–Nb bridges, forming (b) 1′trans- or (c) 1cis-dimer, respectively. | |
The crystal structure of 2 shows a large Nb32-containing 48-tungsto-8-phosphate (Fig. 4), comprising the dimeric [P4W24Nb14O126(H2O)4]18− subunits (Fig. 5a), connected by four Nb–O–Nb bridges in a perpendicular arrangement, and resulting in an adamantane-like {Nb4O6} core (Fig. S3a†). All four Nb ions within {Nb4O6} exhibit octahedral geometry coordinated by five μ2-oxygen atoms and one terminal oxygen atom with an average Nb–O bond length of 1.990 (8) Å. The O–Nb–O bond angles are between 85.5(5) and 95.0(5). Fig. 5a/b illustrates the connectivity within each dimeric subunit, in which two peroxo-free derivatives, [Nb6P2W12O61]10− ({Nb6P2W12}), are linked by two Nb–O–Nb bridges and ‘capped’ by two extra Nb centers. Each of the latter (Nb8 in Fig. 5b) is linked to two oxo ligands of each Wells–Dawson unit, and the coordination sphere is completed by two terminal oxo and two aqua ligands.
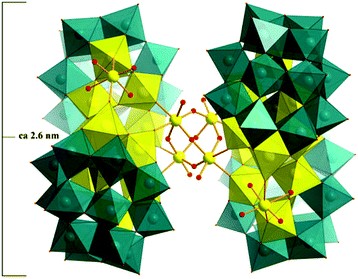 |
| Fig. 4 Combined polyhedral/ball-and-stick representation of polyanion 2. All cations and crystal waters are omitted for clarity. Teal polyhedra {WO6}; yellow polyhedra {NbO6}; yellow balls Nb; dark-yellow polyhedra {PO4}; red balls O. | |
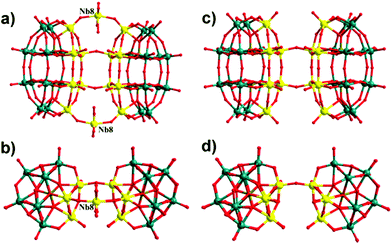 |
| Fig. 5 Comparisons of the subunit (a/b) [P4W24Nb14O126]18− in 2 and (c/d) [P4W24Nb12O122]20− in {Nb28(P2W12)4}11b from different directions. Teal balls W; yellow balls Nb; dark-yellow balls P; red balls O. | |
Alternatively, 2 can be viewed as four {P2W12} fragments supporting an unprecedented {Nb32} core (Fig. 6a), the maximum nuclearity of Nb observed in heteropolyoxometalate chemistry. Such Wells–Dawson-based tetrameric structures have so far been characterized for few compounds,11c,24–28 among which only for {Fe27P8W49}25 and {Nb28(P2W12)4}11c the hexalacunary {P2W12} fragment is maintained.
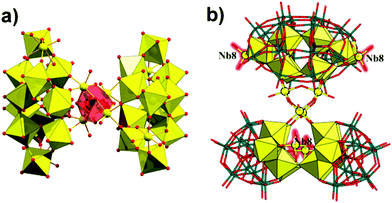 |
| Fig. 6 (a) Representation of the unprecedented {Nb32} cluster in polyanion 2. (b) The Nb8 atoms in polyanion 2 are highlighted in red rendering. | |
Structural analysis reveals that the structure of [H14{P2W12Nb7O63(H2O)2}4{Nb4O4(OH)6}]16− is very similar to that of the recently reported cluster {Nb28(P2W12)4}11c but with subtle differences. For instance, both polyanions form tetrahedrally shaped structures containing four {Nb6P2W12} Wells–Dawson units linked together via an {Nb4O6} adamantine core. Despite the fact that P2W12 is metastable, both polyanions have retained this entity throughout the synthetic process. In the previous {Nb28(P2W12)4}, the subunit is [P4W24Nb12O122]20− in which two {Nb6P2W12} moieties are linked by two Nb–O–Nb bridges (Fig. 5c). However, polyanion 2 comprises a [P4W24Nb14O126]18− subunit in which the two {Nb6P2W12} moieties are fused together by two Nb–O–Nb bridges and the two additional Nb8 atoms. This result indicates that the subunit may be able to react further with adjacent subunits via these exposed axial Nb8 centers, subsequently forming a large structure.
Moreover, the similarity in the fragmentation patterns of 1, {Nb28(P2W12)4} and 2 allow the assumption that these clusters share a similar assembly pathway. The three complexes show how it is possible to increase the number of the encapsulated Nb nuclearity (from 12 and 28 to 32) by slightly changing the synthetic conditions. This result suggests that the new architectures based on the plenary {Nb28(P2W12)4} species may be successfully accessed.
Bond valence sum (BVS) calculations for 1 and 2 are consistent with W and Nb being in the +6 and +5 oxidation states, respectively.29 BVS calculations for all oxygen atoms in 2 suggest that all the four terminal ligands in the {Nb4O6} core are monoprotonated, as well as the two bridging oxygen atoms resulting in an {Nb4(OH)6} core (Fig. S3b†). Charge-balance considerations with counter cations suggested that compounds 1a and 2a contain some protons, namely K7[H13{Nb6(O2)4P2W12O57}2]·31H2O and (NH4)16[H14{P2W12Nb7O63(H2O)2}4{Nb4O4(OH)6}]·16H2O. It should be noted that the very large number of crystallographically independent atoms and parameters makes the direct location of protons from the Fourier maps difficult, as stated already by Nyman and us.30 Thus, we believe that the thirteen protons in 1a are delocalized, whereas six protons are localized and fourteen protons are delocalized in 2a.
IR spectroscopy
The IR spectra of 1a and 2a are similar to that of the plenary Wells–Dawson K3.5Na4[H4.5(NbO2)6P2W12O56]·12.5H2O (3a) (Fig. S4†).11c Interestingly, the characteristic vibration peak of ν(P–O) at about 1074 cm−1 for 1a and 2a shows splitting as compared to the IR spectrum of 3a, which might be attributed to the structural distortion and the consequent lowering of the symmetry. The medium band around 712 cm−1 for 1a and 2a originate from Nb–O–Nb stretching vibrations,12,13a,d,14,31 whilst absent in the IR spectrum of 3a. Additionally, the main difference between 1a and 2a is the weak intensity band at 871 cm−1 for 1a, characteristic of the antisymmetric stretching vibrations of peroxo groups.13a,32 These results are in complete agreement with the solid-state structure.
NMR spectroscopy
The structural novelty of polyanion 2, containing an unprecedented {Nb32} cluster, is very intriguing. We hence explored the solution properties of 2a redissolved in H2O–D2O by multinuclear NMR spectroscopy. The 31P NMR spectrum showed a singlet at δ = −8.1 ppm, consistent with the eight equivalent phosphate groups. In addition, several weak peaks are also observed, and probably attributed to small transformation or decomposition products, such as previously reported in [P2W15Nb3O62]9− reaction system.19c,33 On the other hand, the 183W NMR spectrum exhibits six peaks of equal intensity at δ = −124.4, −135.9, −141.5, −165.4, −192.7, and −195.6 ppm, corresponding to the six inequivalent tungstens of the solid-state structure (Fig. S5†).
Photocatalytic properties
Photocatalytic water-splitting offers a promising way for environmentally friendly solar-hydrogen production. Great efforts have been made on POM-based photocatalysts over the past few years.34 Two tantalotungstates have shown photocatalytic properties in the production of H2, as demonstrated by Liu et al.,35 showing the potential activities of mixed-metal POMs.
To demonstrate the photocatalytic H2 evolution activities of the title compounds, 100 mg of 1a or 2a and 0.05 mg of H2PtCl6 were dissolved in 100 mL of 10% methanol, which was irradiated under UV using a 500 W mercury lamp. In this system, 1a or 2a was used as light photosensitizers and catalysts in the presence of a Pt co-catalyst,36 and methanol was employed as a sacrificial electron donor, which is the source of the electrons required in the reduction half-reaction of water.34c,e,35,37 As shown in Fig. 7b, the H2 evolution rates in the three runs were 1336, 1590 and 1468 μmol h−1 g−1 for compound 2a, respectively. The total evolved H2 over a 12 h span was 1757.7 μmol, with a corresponding turnover number of 298 (moles of H2 formed/moles of 2a). However, for compound 1a, the H2 evolution rates in the three runs were 771, 756 and 723 μmol h−1 g−1, respectively, and the total evolved H2 over the same 12 h span was 900.2 μmol (Fig. 7a).
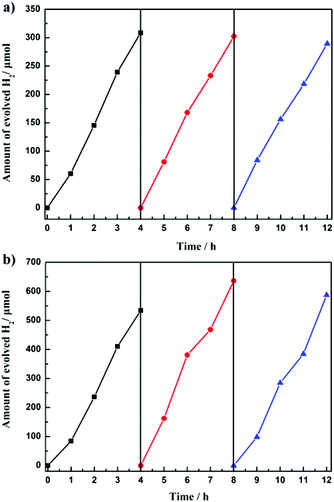 |
| Fig. 7 Time courses of H2 evolution from 100 mg photocatalyst 1a (a) and 2a (b) under UV irradiation in 100 mL of 10% methanol aqueous solution. | |
For comparison, the use of K6[α-P2W18O62]·14H2O (P2W18) (control) and K3.5Na4[H4.5(NbO2)6P2W12O56]·12.5H2O (3a) was tested under otherwise identical reaction conditions (lines c and d in Fig. 8). Evolution of H2 was continuous in 4 h at a rate of 553 and 325 μmol h−1 g−1, respectively, which is significantly smaller than that of 2a. To investigate the important role of 2a in the photocatalytic process, three blank experiments were performed with no H2 evolution observed (line e–g in Fig. 8). On the one hand, relatively no H2 is detected when the Pt-co-catalyst or 2a is absent, indicating that both play a crucial role in light harvesting for photocatalysis. On the other hand, when the sacrificial solvent (CH3OH) is absent, no H2 production was also observed.
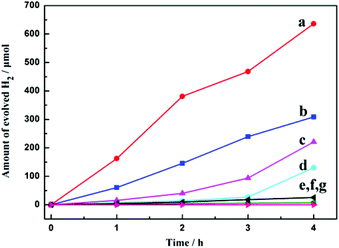 |
| Fig. 8 Time courses of photocatalytic H2 evolution over different photocatalytic systems: (lines a–d) compounds 2a (red), 1a (blue), P2W18 (pink) and 3a (cyan) as photocatalysts; (lines e–g) the absence of Pt-co-catalyst (black), 2a (green), CH3OH (purple). | |
Furthermore, the photocatalysis of 1a, 2a, 3a, and P2W18 was accompanied by a color change of the reaction mixture from colorless to deep blue, indicating reduction of WVI to WV. The color however remained unchanged when the mixture was left under an oxygen-free atmosphere. However, reoxidation of WV to WVI occurred within hours of air exposure, accompanied with the disappearance of the deep blue color (Fig. S6†). In addition, the significantly higher rates when using 1a or 2aversus the P2W18 control clearly indicate a positive photocatalytic effect upon Nb incorporation into heteropolytungstates, which is in agreement with the reduced band gaps of 2.58 and 2.43 eV for 1a and 2a, respectively, (Fig. S7 and S8†).38 Finally, the prominently lower H2 production rates in the case of 1a and 3a compared to 2a may be attributed to the peroxo moieties in their structures, which appear to decrease the catalytic activity. We plan to further study this hypothesis at a later stage.
Conclusion
In conclusion, two multi-Nb-substituted POMs were synthesized using similar reaction conditions. Our work demonstrates that the {Nb6P2W12} fragment can be stabilized as a dimer or a tetramer by careful synthetic control. Polyanion 1 consists of two oxo bridged [Nb6(O2)4P2W12O57]10− units, leading to a dimeric structure. On the other hand, polyanion 2 encapsulates 32 Nb centers, representing the largest transition metal-containing P2W12-based POM characterized so far. Furthermore, 2a exhibits good photocatalytic H2 evolution activity compared to other similar structures. We are currently exploring if other derivatives of 1 and 2 can be prepared.
Experimental section
Materials and methods
All reagents were used as purchased without further purification. K7[HNb6O19]·13H2O and K12[H2P2W12O48]·24H2O were synthesized according to published procedures.39 The purity of both compounds was confirmed by infrared spectroscopy. Infrared (IR) spectra were recorded on a AVATAR360 FT-IR spectrophotometer using KBr pellets in the range of 4000–400 cm−1. The following abbreviations were used to assign the peak intensities: s = strong, m = medium, and w = weak. C, H and N elemental analyses were measured on a PerkinElmer 2400 II CHNS/O analyzer. Nb and W elemental analyses were obtained by PerkinElmer Optima 2100 DV inductively coupled plasma optical emission spectrometry. NMR spectroscopy for compound 2a was carried out at room temperature on a 400 MHz JEOL ECS instrument, Jacobs University, Bremen, Germany. The 31P NMR measurements were performed in 5 mm tubes at a working frequency of 162.1 MHz and H3PO4 (85%) as a reference, whereas the 183W NMR measurements were performed in 10 mm tubes at a working frequency of 16.7 MHz and 1 M Na2WO4 as a reference. Photocatalytic reactions were carried out in a Pyrex inner-irradiation-type reaction vessel with a magnetic stirrer at room temperature. The reactant solution was evacuated using N2 several times to ensure complete air removal and then irradiated using a 500 W mercury lamp. The produced H2 was analyzed by a GC9800 instrument with a thermal conductivity detector and a 5 Å molecular sieve column (2 mm × 2 m) using N2 as a carrier gas.
Synthesis of K7[H13{Nb6(O2)4P2W12O57}2]·31H2O (1a).
K7[HNb6O19]·13H2O (1.60 g, 1.17 mmol) was dissolved in a solution consisting of 13.5 mL of 30% aqueous H2O2 and 165 mL of water with moderate stirring. Next, 1 M HClaq (12.5 mL, 12.5 mmol) was added dropwise to give a bright yellow, effervescent solution. (Caution: If at this stage the reaction solution became cloudy, due to a suspension of Nb2O5, the solution was discarded.) Immediately after the addition of HClaq, and while the mixture was rapidly stirred, finely powdered K12[H2P2W12O48]·24H2O (4.80 g, 1.22 mmol) was added in a single step, resulting in a clear, yellow solution (at this point pH was about 2.2). Fifteen minutes later, the pH was adjusted to ca. 2.0 by adding 0.5 M HClaq. Stirring of the suspension was continued for approximately 1 h and filtered through a filter paper (when necessary) to obtain a clear solution. The filtrate was then heated to 70 °C for 6 hours. A pale yellow precipitate formed upon cooling was removed by filtration, followed by the addition of KCl (2.65 g, 35.6 mmol). The solution was then stirred for 30 min and filtered. The resulting pale yellow filtrate was kept at room temperature to allow slow evaporation. Yellow needle-shaped crystals formed within four weeks. Yield: 0.51 g, 0.06 mmol (10% based on Nb). IR (cm−1): 1397(s), 1082(s), 1059(m), 1002(w), 954(s), 898(m), 871(w), 784(s), 712(m), 650(m). Elemental analysis for 1a (Mw 8576.1 g mol−1), % calculated: K 3.2, P 1.4, Nb 13.0, W 51.4; % found: K 3.4, P 1.4, Nb 12.8, W 51.9.
Synthesis of (NH4)16[H14{P2W12Nb7O63(H2O)2}4{Nb4O4(OH)6}]·16H2O (2a).
K7[HNb6O19]·13H2O (2.00 g, 1.47 mmol) was dissolved in a solution consisting of 13.5 mL of 30% aqueous H2O2 and 165 mL of water with moderate stirring. Next, 1 M HClaq (12.5 mL, 12.5 mmol) was added dropwise to give a bright yellow, effervescent solution. Finely powdered K12[H2P2W12O48]·24H2O (4.80 g, 1.22 mmol) was added quickly in a single step, resulting in a clear, yellow solution. Fifteen minutes later, the pH was adjusted to ca. 2.0 by adding 0.5 M HClaq. Stirring of the suspension was continued for approximately 1 h and filtered through a filter paper (when necessary) to obtain a clear solution. The filtrate was adjusted to 1.7 by the addition of HClaq and then heated to 80 °C until the yellow color disappeared. Then NH4Cl (1.90 g, 35.6 mmol) was added to the filtrate. Pale yellow block-shaped crystals formed within four weeks. Yield: 1.32 g, 0.10 mmol (35% based on Nb). IR (cm−1): 1402(s), 1086(s), 1059(m), 1002(w), 955(s), 900(m), 871(w), 781(s), 712(s), 590(w), 555(s), 493(m), 427(w). Elemental analysis for 2a (Mw 16978.1 g mol−1), % calculated: N 1.3, P 1.5, Nb 17.5, W 52.0; % found: N 1.3, P 1.5, Nb 17.8, W 52.5.
X-ray crystallography
Suitable single crystals were selected and placed in a thin glass tube due to efflorescence. X-ray diffraction intensity data were recorded at 296(2) K on a Bruker Apex-II CCD diffractometer using Mo Kα monochromated radiation (λ = 0.71073 Å). Structure solution and refinement were carried out with SHELXS-97 and SHELXL-97 program packages.40 No hydrogen atoms associated with the water molecules were located from the difference Fourier map. All hydrogen atoms were refined isotropically as a riding mode using the default SHELXTL parameters. Selected details of the data collection and structural refinement of compounds 1a and 2a can be found in Table 2 and full details are available in the corresponding CIF files. CSD-426569 (1a) and CSD-426570 (2a) can be obtained free of charge from the Fachinformationszentrum Karlsruhe, 76344 Eggenstein-Leopoldshafen, Germany (fax: (+49)7247-808-666; e-mail: crysdata@fiz-karlsruhe.de, http://www.fiz-karlsruhe.de/request_for_deposited_data.html).
Table 2 Crystal data and structure refinement
|
1a
|
2a
|
R
1 = ∑||Fo| − |Fc||/∑|Fo|.
wR2 = {∑[w(Fo2 − Fc2)2]/∑[w(Fo2)2]}1/2.
|
Empirical formula |
K7H75Nb12P4W24O161 |
N16H132P8W48Nb32O286 |
Formula weight |
8576.1 |
16978.1 |
Crystal system |
Monoclinic |
Orthorhombic |
Space group |
C2/c |
Fddd
|
a/Å |
42.852(3) |
24.0163(8) |
b/Å |
12.6794(8) |
44.0686(14) |
c/Å |
29.4933(18) |
72.836(2) |
β/° |
101.4760(10) |
90 |
V/Å3 |
15 704.4(17) |
77 087(4) |
Z
|
4 |
8 |
D
c/g cm−3 |
3.620 |
2.923 |
μ/mm−1 |
18.681 |
15.288 |
F
000
|
15 228 |
60 000 |
Crystal size/mm3 |
0.47 × 0.28 × 0.23 |
0.22 × 0.15 × 0.12 |
Reflns collected |
37 625 |
97 316 |
Indep reflns |
13 691 |
16 835 |
R
int
|
0.1227 |
0.1026 |
Goodness-of-fit on F2 |
1.024 |
1.010 |
R
1 [I > 2σ(I)]a |
0.0982 |
0.0548 |
wR2 (all data)b |
0.2599 |
0.1557 |
Acknowledgements
We gratefully acknowledge support from the NSFC (grant numbers 91222102 and 21371048) and the NSF from Henan Province (grant numbers 122300410126, 13A150058 and 142300410009).
Notes and references
-
M. T. Pope, Heteropoly and Isopoly Oxometalates, Springer, New York, 1983 Search PubMed.
-
(a) Special edition:
C. L. Hill (guest ed.), Chem. Rev., 1998, 98, 1–390 Search PubMed;
(b) D.-L. Long, R. Tsunashima and L. Cronin, Angew. Chem., Int. Ed., 2010, 49, 1736–1758 CrossRef CAS PubMed.
-
(a) A. Dolbecq, E. Dumas, C. R. Mayer and P. Mialane, Chem. Rev., 2010, 110, 6009–6048 CrossRef CAS PubMed;
(b) J. M. Cameron, J. Gao, D.-L. Long and L. Cronin, Inorg. Chem. Front., 2014, 1, 178–185 RSC;
(c) H. N. Miras, L. Vilà-Nadal and L. L. Cronin, Chem. Soc. Rev., 2014, 43, 5679–5699 RSC;
(d) P. Yang, Y. Xiang, Z. Lin, B. S. Bassil, J. Cao, L. Fan, Y. Fan, M.-X. Li, P. Jimnez-Lozano, J. J. Carb, J. M. Poblet and U. Kortz, Angew. Chem., Int. Ed., 2014, 53, 11974–11978 CrossRef CAS PubMed.
-
(a) Q. S. Yin, J. M. Tan, C. Besson, Y. V. Geletii, D. G. Musaev, A. E. Kuznetsov, Z. Luo, K. I. Hardcastle and C. L. Hill, Science, 2010, 328, 342–345 CrossRef CAS PubMed;
(b) Special edition:
D.-L. Long and L. Cronin (guest ed.), Dalton Trans., 2012, 41, 9799–10106 Search PubMed;
(c)
U. Kortz and T. Liu (Guest Eds.), Eur. J. Inorg. Chem., 2013, 2013, 1556–1967 Search PubMed;
(d) X.-B. Han, Z.-M. Zhang, T. Zhang, Y.-G. Li, W. Lin, W. You, Z.-M. Su and E.-B. Wang, J. Am. Chem. Soc., 2014, 136, 5359–5366 CrossRef CAS PubMed.
-
(a) D.-L. Long, E. Burkholder and L. Cronin, Chem. Soc. Rev., 2007, 36, 105–121 RSC;
(b) Special edition:
U. Kortz (guest ed.), Eur. J. Inorg. Chem., 2009, 2009, 5055–5276 Search PubMed;
(c) Special edition:
L. Cronin and A. Müller (guest ed.), Chem. Soc. Rev., 2012, 41, 7325–7648 Search PubMed;
(d) Y. Yang, B. Zhang, Y. Wang, L. Yue, W. Li and L. Wu, J. Am. Chem. Soc., 2013, 135, 14500–14503 CrossRef CAS PubMed;
(e) D.-Y. Du, L.-K. Yan, Z.-M. Su, S.-L. Li, Y.-Q. Lan and E.-B. Wang, Coord. Chem. Rev., 2013, 257, 702–717 CrossRef CAS PubMed.
-
(a) S.-T. Zheng and G.-Y. Yang, Chem. Soc. Rev., 2012, 41, 7623–7646 RSC;
(b) Z. Zhou, D. Zhang, L. Yang, P. Ma, Y. Si, U. Kortz, J. Niu and J. Wang, Chem. Commun., 2013, 49, 5189–5191 RSC;
(c) J. M. Cameron, J. Gao, L. Vilà-Nadal, D.-L. Long and L. Cronin, Chem. Commun., 2014, 50, 2155–2157 RSC;
(d) Z.-G. Jiang, K. Shi, Y.-M. Lin and Q.-M. Wang, Chem. Commun., 2014, 50, 2353–2355 RSC;
(e) J. H. Son, C. A. Ohlin, R. L. Johnson, P. Yu and W. H. Casey, Chem. – Eur. J., 2013, 19, 5191–5197 CrossRef CAS PubMed;
(f) J.-Q. Shen, Q. Wu, Y. Zhang, Z.-M. Zhang, Y.-G. Li, Y. Lu and E.-B. Wang, Chem. – Eur. J., 2014, 20, 2840–2848 CrossRef CAS PubMed;
(g) M. Ibrahim, Y. Xiang, B. S. Bassil, Y. Lan, A. K. Powell, P. de Oliveira, B. Keita and U. Kortz, Inorg. Chem., 2013, 52, 8399–8408 CrossRef CAS PubMed;
(h) T. Ueda, M. Ohnishi, M. Shiro, J. I. Nambu, T. Yonemura, J. F. Boas and A. M. Bond, Inorg. Chem., 2014, 53, 4891–4898 CrossRef CAS PubMed;
(i) M. Ibrahim, A. Haider, Y. Lan, B. S. Bassil, A. M. Carey, R. Liu, G. Zhang, B. Keita, W. Li, G. E. Kostakis, A. K. Powell and U. Kortz, Inorg. Chem., 2014, 53, 5179–5188 CrossRef CAS PubMed;
(j) R. Al-Oweini, B. S. Bassil, J. Friedl, V. Kottisch, M. Ibrahim, M. Asano, B. Keita, G. Novitchi, Y. Lan, A. Powell, U. Stimming and U. Kortz, Inorg. Chem., 2014, 53, 5663–5673 CrossRef CAS PubMed;
(k) P. I. Molina, H. N. Miras, D.-L. Long and L. Cronin, Dalton Trans., 2014, 43, 5190–5199 RSC.
-
(a) B. Godin, Y.-G. Chen, J. Vaissermann, L. Ruhlmann, M. Verdaguer and P. Gouzerh, Angew. Chem., Int. Ed., 2005, 44, 3072–3075 CrossRef CAS PubMed;
(b) B. Godin, J. Vaissermann, P. Herson, L. Ruhlmann, M. Verdaguer and P. Gouzerh, Chem. Commun., 2005, 5624–5626 RSC;
(c) T. Boyd, S. G. Mitchell, H. N. Miras, D.-L. Long and L. Cronin, Dalton Trans., 2010, 39, 6460–6465 RSC.
-
(a) Z.-M. Zhang, S. Yao, Y.-G. Li, Y.-H. Wang, Y.-F. Qi and E.-B. Wang, Chem. Commun., 2008, 1650–1652 RSC;
(b) Y.-W. Li, Y.-G. Li, Y.-H. Wang, X.-J. Feng, Y. Lu and E.-B. Wang, Inorg. Chem., 2009, 48, 6452–6458 CrossRef CAS PubMed;
(c) X. Fang, P. Kögerler, Y. Furukawa, M. Speldrich and M. Luban, Angew. Chem., Int. Ed., 2011, 50, 5212–5216 CrossRef CAS PubMed.
-
(a) Z. Zhang, S. Yao, Y. Qi, Y. Li, Y. Wang and E. Wang, Dalton Trans., 2008, 3051–3053 RSC;
(b) S. Yao, Z. Zhang, Y. Li and E. Wang, Dalton Trans., 2010, 3884–3889 RSC;
(c) S. G. Mitchell, S. Khanra, H. N. Miras, T. Boyd, D.-L. Long and L. Cronin, Chem. Commun., 2009, 2712–2714 RSC.
- S. S. Mal, M. H. Dickman and U. Kortz, Chem. – Eur. J., 2008, 14, 9851–9855 CrossRef CAS PubMed.
-
(a) D. A. Judd, Q. Chen, C. F. Campana and C. L. Hill, J. Am. Chem. Soc., 1997, 119, 5461–5462 CrossRef CAS;
(b) Y. Ren, Y. Hu, Y. Shan, Z. Kong, M. Gu, B. Yue and H. He, Inorg. Chem. Commun., 2014, 40, 108–111 CrossRef CAS PubMed;
(c) D. Zhang, Z. Liang, S. Xie, P. Ma, C. Zhang, J. Wang and J. Niu, Inorg. Chem., 2014, 53, 9917–9922 CrossRef CAS PubMed.
- R. G. Finke and M. W. Droege, J. Am. Chem. Soc., 1984, 106, 7274–7277 CrossRef CAS.
-
(a) G.-S. Kim, H. Zeng, W. A. Neiwert, J. J. Cowan, D. VanDerveer, C. L. Hill and I. A. Weinstock, Inorg. Chem., 2003, 42, 5537–5544 CrossRef CAS PubMed;
(b) G.-S. Kim, H. Zeng and C. L. Hill, Bull. Korean Chem. Soc., 2003, 24, 1005–1008 CrossRef CAS;
(c) G.-S. Kim, H. D. Zeng, J. T. Rhule, I. A. Weinstock and C. L. Hill, Chem. Commun., 1999, 1651–1652 RSC;
(d) G.-S. Kim, H. D. Zeng, D. VanDerveer and C. L. Hill, Angew. Chem., Int. Ed., 1999, 38, 3205–3207 CrossRef CAS;
(e) M. K. Harrup, G.-S. Kim, H. Zeng, R. P. Johnson, D. VanDerveer and C. L. Hill, Inorg. Chem., 1998, 37, 5550–5556 CrossRef CAS PubMed.
-
(a) S. J. Li, S. X. Liu, C. C. Li, F. J. Ma, D. D. Liang, W. Zhang, R. K. Tan, Y. Y. Zhang and L. Xu, Chem. – Eur. J., 2010, 16, 13435–13442 CrossRef CAS PubMed;
(b) S. J. Li, S. X. Liu, C. C. Li, F. J. Ma, W. Zhang, D. D. Liang, R. K. Tan, Y. Y. Zhang and Q. Tang, Inorg. Chim. Acta, 2011, 376, 296–301 CrossRef CAS PubMed.
- D. Zhang, S. Li, J. Wang and J. Niu, Inorg. Chem. Commun., 2012, 17, 75–78 CrossRef CAS PubMed.
- G.-S. Kim, D. A. Judd, C. L. Hill and R. F. Schinazi, J. Med. Chem., 1994, 37, 816–820 CrossRef CAS.
- E. Radkov and R. H. Beer, Polyhedron, 1995, 14, 2139–2143 CrossRef CAS.
- D. A. Judd, J. H. Nettles, N. Nevins, J. P. Snyder, D. C. Liotta, J. Tang, J. Ermolieff, R. F. Schinazi and C. L. Hill, J. Am. Chem. Soc., 2001, 123, 886–897 CrossRef CAS PubMed.
-
(a) D. J. Edlund, R. J. Saxton, D. K. Lyon and R. G. Finke, Organometallics, 1988, 7, 1692–1704 CrossRef CAS;
(b) R. G. Finke, D. K. Lyon, K. Nomiya and T. J. R. Weakley, Acta Crystallogr., Sect. C: Cryst. Struct. Commun., 1990, 46, 1592–1596 CrossRef;
(c) H. Weiner, J. D. Aiken III and R. G. Finke, Inorg. Chem., 1996, 35, 7905–7913 CrossRef CAS;
(d) W. W. Laxson, S. Özkar and R. G. Finke, Inorg. Chem., 2014, 53, 2666–2676 CrossRef CAS PubMed.
-
(a) C. J. Besecker, V. W. Day, W. G. Klemperer and M. R. Thompson, Inorg. Chem., 1995, 24, 44–50 CrossRef;
(b) J. M. Maestre, J. P. Sarasa, C. Bo and J. M. Poblet, Inorg. Chem., 1998, 37, 3071–3077 CrossRef CAS;
(c) T. M. Che, V. W. Day, L. C. Francesconi, M. F. Fredrich, W. G. Klemperer and W. Shum, Inorg. Chem., 1985, 24, 4055–4062 CrossRef CAS;
(d) F. Bannani, R. Thouvenot and M. Debbabi, Eur. J. Inorg. Chem., 2007, 4357–4363 CrossRef CAS;
(e) H. Driss, K. Boubekeur, M. Debbabi and R. Thouvenot, Eur. J. Inorg. Chem., 2008, 3678–3686 CrossRef CAS;
(f) S.-J. Li, S.-X. Liu, N.-N. Ma, Y.-Q. Qiu, J. Miao, C.-C. Li, Q. Tang and L. Xu, CrystEngComm, 2012, 14, 1397–1404 RSC.
-
(a) I. M. Mbomekalle, B. Keita, M. Nierlich, U. Kortz, P. Berthet and L. Nadjo, Inorg. Chem., 2003, 42, 5143–5152 CrossRef CAS PubMed;
(b) F. Li and L. Xu, Dalton Trans., 2011, 40, 4024–4034 RSC;
(c) O. Oms, A. Dolbecq and P. Mialane, Chem. Soc. Rev., 2012, 41, 7497–7536 RSC;
(d) N. V. Izarova, M. T. Pope and U. Kortz, Angew. Chem., Int. Ed., 2012, 51, 9492–9510 CrossRef CAS PubMed;
(e) J. Gao, J. Yan, S. Beeg, D.-L. Long and L. Cronin, J. Am. Chem. Soc., 2013, 135, 1796–1805 CrossRef CAS PubMed;
(f) L. Huang, S.-S. Wang, J.-W. Zhao, L. Cheng and G.-Y. Yang, J. Am. Chem. Soc., 2014, 136, 7637–7642 CrossRef CAS PubMed.
-
(a) X. Fang and P. Kögerler, Angew. Chem., Int. Ed., 2008, 47, 8123–8126 CrossRef CAS PubMed;
(b) S. G. Mitchell, C. Streb, H. N. Miras, T. Boyd, D.-L. Long and L. Cronin, Nat. Chem., 2010, 2, 308–312 CrossRef CAS PubMed.
- Z. M. Zhang, Y. G. Li, Y. H. Wang, Y. F. Qi and E. B. Wang, Inorg. Chem., 2008, 47, 7615–7622 CrossRef CAS PubMed.
-
(a) U. Kortz, S. S. Hamzeh and N. A. Nasser, Chem. – Eur. J., 2003, 9, 2945–2952 CrossRef CAS;
(b) Y. Sakai, S. Ohta, Y. Shintoyo, S. Yoshida, Y. Taguchi, Y. Matsuki, S. Matsunaga and K. Nomiya, Inorg. Chem., 2011, 50, 6575–6583 CrossRef CAS PubMed;
(c) Y. Sakai, K. Yoza, C. N. Kato and K. Nomiya, Chem. – Eur. J., 2003, 9, 4077–4083 CrossRef CAS PubMed;
(d) Y. Sakai, S. Yoshida, T. Hasegawa, H. Murakami and K. Nomiya, Bull. Chem. Soc. Jpn., 2007, 80, 1965–1974 CrossRef CAS.
- B. Godin, Y. G. Chen, J. Vaissermann, L. Ruhlmann, M. Verdaguer and P. Gouzerh, Angew. Chem., Int. Ed., 2005, 44, 3072–3075 CrossRef CAS PubMed.
-
(a) C. P. Pradeep, D.-L. Long, P. Kögerler and L. Cronin, Chem. Commun., 2007, 4254–4256 RSC;
(b) P. I. Molina, H. N. Miras, D.-L. Long and L. Cronin, Dalton Trans., 2014, 43, 5190–5199 RSC.
- C. Lydon, M. M. Sabi, M. D. Symes, D. L. Long, M. Murrie, S. Yoshii, H. Nojiri and L. Cronin, Chem. Commun., 2012, 48, 9819–9821 RSC.
- M. Ibrahim, Y. X. Xiang, B. S. Bassil, Y. H. Lan, A. K. Powell, P. Oliveira, B. Keita and U. Kortz, Inorg. Chem., 2013, 52, 8399–8408 CrossRef CAS PubMed.
- D. Altermatt and I. D. Brown, Acta Crystallogr., Sect. B: Struct. Sci., 1985, 41, 244–247 CrossRef.
-
(a) R. P. Bontchev and M. Nyman, Angew. Chem., Int. Ed., 2006, 45, 6670 CrossRef CAS PubMed;
(b) J. Niu, P. Ma, H. Niu, J. Li, J. Zhao, Y. Song and J. Wang, Chem. – Eur. J., 2007, 13, 8739 CrossRef CAS PubMed.
-
(a) V. W. Day, W. G. Klemperer and C. Schwartz, J. Am. Chem. Soc., 1987, 109, 6030–6044 CrossRef CAS;
(b) D. J. Edlund, R. J. Saxton, D. K. Lyon and R. G. Finke, Organometallics, 1988, 7, 1692–1704 CrossRef CAS;
(c) Y. J. Lu, R. Lalancette and R. H. Beer, Inorg. Chem., 1996, 35, 2524–2529 CrossRef CAS PubMed.
- M. Roch, J. Weber and A. F. Williams, Inorg. Chem., 1984, 23, 4571–4580 CrossRef CAS.
-
(a) M. Pohl, Y. Lin, T. J. R. Weakley, K. Nomiya, M. Kaneko, H. Weiner and R. G. Finke, Inorg. Chem., 1995, 34, 767–777 CrossRef CAS;
(b) B. J. Hornstein and R. G. Finke, Inorg. Chem., 2002, 41, 2720–2730 CrossRef CAS PubMed.
-
(a) Q. Yin, J. M. Tan, C. Besson, Y. V. Geletii, D. G. Musaev, A. E. Kuznetsov, Z. Luo, K. I. Hardcastle and C. L. Hill, Science, 2010, 328, 342–345 CrossRef CAS PubMed;
(b) Z. Zhang, Q. Lin, D. Kurunthu, T. Wu, F. Zuo, S.-T. Zheng, C. J. Bardeen, X. Bu and P. Feng, J. Am. Chem. Soc., 2011, 133, 6934–6937 CrossRef CAS PubMed;
(c) P. Huang, C. Qin, Z. M. Su, Y. Xing, X. L. Wang, K. Z. Shao, Y. Q. Lan and E. B. Wang, J. Am. Chem. Soc., 2012, 134, 14004–14010 CrossRef CAS PubMed;
(d) X.-L. Hu, C.-Y. Sun, C. Qin, X.-L. Wang, H.-N. Wang, E.-L. Zhou, W.-E. Li and Z.-M. Su, Chem. Commun., 2013, 49, 3564–3566 RSC;
(e) J.-H. Son, J. Wang, F. E. Osterloh, P. Yu and W. H. Casey, Chem. Commun., 2014, 50, 836–838 RSC;
(f) Y.-Q. Jiao, C. Qin, X.-L. Wang, F.-H. Liu, P. Huang, C.-G. Wang, K.-Z. Shao and Z.-M. Su, Chem. Commun., 2014, 50, 5961–5963 RSC;
(g) B. Rausch, M. D. Symes, G. Chisholm and L. Cronin, Science, 2014, 325, 1326–1330 CrossRef PubMed.
- S. Li, S. Liu, S. Liu, Y. Liu, Q. Tang, Z. Shi, S. Ouyang and J. Ye, J. Am. Chem. Soc., 2012, 134, 19716–19721 CrossRef CAS PubMed.
- H2PtCl6 was reduced to Pt particles at the beginning of the photocatalytic reaction.
-
(a) E. Papaconstantinou, Chem. Soc. Rev., 1989, 18, 1–31 RSC;
(b) D. C. Duncan, T. L. Netzel and C. L. Hill, Inorg. Chem., 1995, 34, 4640–4646 CrossRef CAS;
(c) J. H. Yang, D. E. Wang, H. X. Han and C. Li, Acc. Chem. Res., 2013, 46, 1900–1909 CrossRef CAS PubMed.
-
J. I. Pankove, Optical Processes in Semiconductors, Prentice-Hall, Englewood Cliffs, 1971 Search PubMed.
-
(a) M. Filowitz, R. K. C. Ho, W. G. Klemperer and W. Shum, Inorg. Chem., 1979, 18, 93–103 CrossRef CAS;
(b) R. Contant, Inorg. Synth., 1990, 27, 104–111 CrossRef CAS.
-
(a) G. Sheldrick, Acta Crystallogr., Sect. A: Fundam. Crystallogr., 1990, 46, 467–473 CrossRef;
(b) G. Sheldrick, Acta Crystallogr., Sect. A: Fundam. Crystallogr., 2008, 64, 112–122 CrossRef CAS PubMed.
Footnote |
† Electronic supplementary information (ESI) available: X-ray crystallographic data in CIF format, Fig. S1–S8, and BVS results of all of the oxygen atoms on polyanions 1 and 2. CSD 426569 (1) and 426570 (2). For ESI and crystallographic data in CIF or other electronic format see DOI: 10.1039/c4qi00169a |
|
This journal is © the Partner Organisations 2015 |