DOI:
10.1039/C4NR04005H
(Paper)
Nanoscale, 2015,
7, 294-300
Magnetic amphiphilic hybrid carbon nanotubes containing N-doped and undoped sections: powerful tensioactive nanostructures†
Received
16th July 2014
, Accepted 28th October 2014
First published on 3rd November 2014
Abstract
In this work, unique amphiphilic magnetic hybrid carbon nanotubes (CNTs) are synthesized and used as tensioactive nanostructures in different applications. These CNTs interact very well with aqueous media due to the hydrophilic N-doped section, whereas the undoped hydrophobic one has strong affinity for organic molecules. The amphiphilic character combined with the magnetic properties of these CNTs opens the door to completely new and exciting applications in adsorption science and catalysis. These amphiphilic N-doped CNTs can also be used as powerful tensioactive emulsification structures. They can emulsify water/organic mixtures and by a simple magnetic separation the emulsion can be easily broken. We demonstrate the application of these CNTs in the efficient adsorption of various molecules, in addition to promoting biphasic processes in three different reactions, i.e. transesterification of soybean oil, quinoline extractive oxidation with H2O2 and a metal-catalyzed aqueous oxidation of heptanol with molecular oxygen.
Introduction
Water-based biphasic systems are extremely important alternatives to develop greener industrial processes.1–3 In general, the use of a surfactant is necessary to improve the phase transfer, but this introduces several operational, technical and economical drawbacks.4 Solid particles can also promote phase transfer processes and can be recovered more easily. Recently, composites based on hydrophobic carbon nanomaterials supported on hydrophilic oxide particles have been used to stabilize water–oil emulsions and catalyze reactions at the liquid–liquid interface.5–9 Given that the development of robust amphiphilic nanostructures highly efficient in promoting emulsions has been very recently identified as an important challenge for colloidal materials,10 we believe that further research in this area is of considerable interest.
The presence of nitrogen atoms in the carbon nanotube (CNT) structure introduces several important physico-chemical properties, such as an increase in polarity, improved dispersion in different liquids and matrices, electrical conductivity and higher reactivity towards different molecules, offering the possibility of new surface CNT functionalization.11–14 At least three different types of nitrogen species are found in N-doped CNTs: pyridinic, pyrrolic and quaternary nitrogen. Various strategies have been successfully used to produce N-doped CNTs, varying from solvothermal, CNT functionalization, N-containing polymer decomposition, to CVD processes using different N-sources such as ethylenediamine, acetonitrile, pyridine, ammonia or aniline.12 In all these studies, the N atoms are distributed throughout the CNT structure.15,16
In this work, we have produced hybrid amphiphilic CNTs, containing in the same nanotube two different sections: a hydrophobic undoped part connected to a hydrophilic N-doped segment (Fig. 1). These hybrid CNTs were produced by a two stage reaction over an iron supported catalyst, i.e. the first step using pure ethylene for the growth of the undoped CNT section, which is completely hydrophobic, and the second step using acetonitrile as the C/N source to produce in the same tube a more hydrophilic/polar section. The possibility to create such N–C CNT heterojunctions has been recently proposed.17
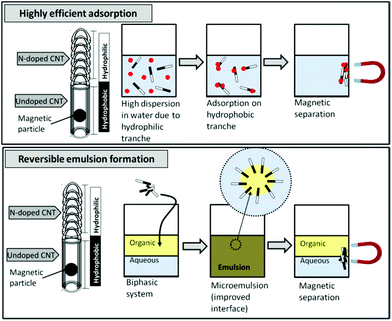 |
| Fig. 1 Application of the hybrid N-doped CNTs for adsorption of organics in water and the reversible emulsion formation process. | |
These hybrid CNTs show two very interesting features: (i) due to the combination of hydrophobic/hydrophilic sections, they can interact with polar and apolar media and should behave like tensioactive species, and (ii) due to the presence of remaining encapsulated Fe catalyst particles,18 these CNTs are magnetic. These properties can pave the way for many new and exciting applications in adsorption science and catalysis. In adsorption processes, especially of organics in an aqueous medium, the hydrophilic section should allow very good dispersion of the CNTs in water, while the highly exposed CNT hydrophobic surface should efficiently adsorb organic molecules (Fig. 1). These amphiphilic CNTs should also allow the reversible emulsion formation and breaking by the application of a magnetic field (Fig. 1).
Herein, we describe the synthesis and characterization of magnetic amphiphilic N-doped CNTs, and their application in the efficient adsorption of the hormone ethinylestradiol (EE) and 16 different polyaromatic hydrocarbons (PAHs), which are hazardous water contaminants. We also report their use for three different biphasic reactions, i.e. transesterification of soybean oil, quinoline extractive oxidation with H2O2 and a metal-catalyzed aqueous oxidation of heptanol with molecular oxygen.
Experimental
CNT synthesis
A simple impregnation procedure was used to deposit the Fe catalyst (20 wt% using Fe(NO3)3) present in an aqueous solution onto the surface of γ-Al2O3 (SCCa 5/150 Alumina powder from Sasol, SBET = 172 m2 g–1). After drying in an oven overnight at 130 °C, the resulting powder was calcined in air at 450 °C. Prior to the carbon growth, the Fe catalyst was pre-reduced in situ with hydrogen for 30 min at 650 °C. The hybrid carbon nanotubes were synthesized by a catalytic CVD process in a fluidized bed reactor using ethylene as the carbon source and acetonitrile as the carbon/nitrogen source, at the same temperature. A typical experiment was carried out initially with ethylene (600 mL min−1) to produce hydrophobic CNTs. After this first reaction stage the gas inlet was switched to acetonitrile/N2 (600 mL min–1 N2, ca. 0.19 bar vapor pressure of acetonitrile corresponding to a temperature of 35 °C) in order to continue the nanotube growth doped with nitrogen. Four samples were produced: (i) ethylene alone for 30 min, (ii) acetonitrile/N2 for 30 min, (iii) ethylene for 10 min, followed by acetonitrile/N2 for 20 min, and (iv) acetonitrile/N2 for 20 min followed by ethylene for 10 min. The CNTs were purified with an aqueous solution of H2SO4 (50 vol%) under reflux for 3 h to facilitate the total dissolution of alumina and partial elimination of exposed iron particles contained in the nanotubes.
Ru/CNT and Pd/CNT catalyst preparation
In order to prepare the supported metal catalysts, the desired amounts of palladium(II) nitrate (Pd(NO3)2·xH2O) and ruthenium(III) nitrosyl nitrate solution (Ru(NO)(NO3)x(OH)y) were added to an acetone solution (20 mL) containing 0.5 g of nanotubes, so as to introduce 2 wt% of the metal phase. After stirring overnight at ambient temperature, the catalysts were filtered, washed with acetone and dried in an oven at 393 K. The catalysts were then reduced at 300 °C for 2 hours in a horizontal oven (20 vol% H2 in Ar).
Characterization
The hybrid nanostructures were characterized using a transmission electronic microscope (TEM-FEI Tecnai-G2-20-FEI 2006, at 200 kV), CHN Perkin-Elmer elemental analyzer, Raman spectroscope (SENTERRA at = 633 nm), and TG/DTA Shimadzu, under air (10 °C min−1 to 900 °C). The textural characterization (BET surface areas, SBET) of the materials was based on the N2 adsorption isotherms determined at −196 °C with Quantachrome Autosorb apparatus. Potentiometric titration (Metrhomn 670 automatic titrator) used 50 mg of CNT dispersed in 25 mL of aqueous HCl solution (0.0070 mol L−1) and KNO3 (0.04 mol L−1) solution directly into the electrochemical cell and titrated with a CO2-free NaOH solution (0.0524 mol L−1) and the data were treated according to the literature.19,20
Adsorption of ethinylestradiol (EE)
The adsorption experiments were carried out with 50 mL of ethinylestradiol aqueous solution (20 ppm) and 5 mg of nanotubes or commercial activated carbon. After 24 h, the mixture was filtered and the supernatant was analyzed using a UV-VIS spectrophotometer (Shimadzu UV 2550–280 nm).
Biphasic oxidation of quinolone
The biphasic oxidation of quinoline dissolved in cyclohexane (5 mL, 500 ppm) was carried out with an aqueous solution of iron chloride (1 mL FeCl2·4H2O 5.6 mmol L−1), hydrogen peroxide 30 wt% (300 μL), and formic acid (300 μL, 98 wt%) in the presence of 1 wt% of CNT. The reaction was emulsified after 20 s sonication. The reaction was monitored by magnetically breaking the emulsion and analyses of quinoline in the organic phase was performed by gas chromatography (Shimadzu GC 17A column Equity-5).
Transesterification of soybean oil with methanol
Biodiesel was synthesized by reaction of soybean oil (20 mL) and methanol (6 mL) with KOH as a catalyst 2 wt% (over the oil mass) and 5 mg of the E10A20 nanotube, at room temperature. A test in the absence of carbon nanotubes was also performed. The mixtures were homogenized in a vortex mixer (Certomat MV) for 3 min. The reaction products were characterized using an Infraspec VFA-IR Spectrometer.
Oxidation of 2-heptanol in water with molecular oxygen
The oxidation reaction of 2-heptanol was performed in a 30 mL stainless steel autoclave with magnetic stirring in a silicone oil bath. In a typical run, the reaction mixture contained 0.1 g of catalyst, 2 mmol of 2-heptanol, and a solution of 10 mL toluene and 5 mL water. The reactor was then pressurized with air (5 bar) before the temperature was increased to 358 K and the reaction was allowed to proceed for 3 hours. The analysis was performed on a Clarus 500 gas chromatograph, equipped with a split/splitless injector, a capillary column (Stabilwax®-DA Fused Silica 30 m, 0.25 mm i.d.,) and a flame ionization detector (FID). After the reaction, the catalyst was separated from the reaction products by filtration. The catalyst was then washed first with acetone, then with an aqueous solution of NaOH (0.5 M) and finally with distilled water, before being dried at 383 K for 12 h in an oven.
Results and discussion
Magnetic amphiphilic hybrid carbon nanotube synthesis and characterization
The hybrid CNTs were synthesized by chemical vapor deposition using ethylene as the C source and acetonitrile as the C/N source (see Experimental section). Four different samples were produced: (i) using ethylene only for 30 min (E30); (ii) using acetonitrile only for 30 min (A30); (iii) using ethylene for 10 min, followed by acetonitrile for 20 min (E10A20); and (iv) acetonitrile for 20 min, followed by ethylene for 10 min (A20E10). TEM images of the E30 sample showed the presence of very regular multi-walled CNTs with an average diameter of ca. 12 nm (Fig. 2). On the other hand, the sample A30 showed the classical “bamboo-like” structure of N-doped CNTs, with larger diameters (ca. 18 nm, Fig. 2). The introduction of nitrogen induces a significant decrease of the yield of the reaction (Table 1), presumably due to different growth mechanisms.21 The production of such defective CNTs has already been reported, when acetonitrile was used as a carbon and nitrogen source.22,23
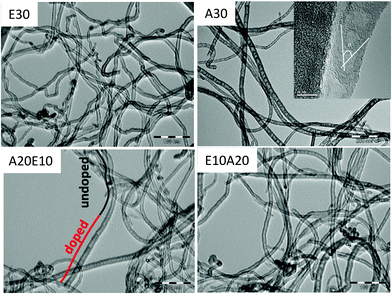 |
| Fig. 2 TEM images of CNTs containing 0 (E30), 0.4 (E10A20), 5.0 (A20E10) and 6.1 wt% (A30) nitrogen. | |
Table 1 Yield, BET surface area, and the C/N content for the produced CNTs
Sample |
Yield (gC gcat–1) |
Diam. (nm) |
S
BET (m2 g–1) |
%C (wt%) |
%N (wt%) |
E30 |
4.8 |
12 |
258 |
94.6 |
0 |
E10A20 |
4.5 |
16 |
257 |
94.8 |
0.4 |
A20E10 |
3.7 |
18 |
196 |
86.6 |
5.0 |
A30 |
1.1 |
18 |
194 |
76.9 |
6.1 |
For the samples E10A20 and A20E10 we have been able to observe a hybrid structure with two different sections in the same CNT: a regular section suggesting the presence of an undoped CNT (indicated by a black line in Fig. 2), which continues to be a bamboo-like CNT, suggesting the presence of an N-doped CNT (indicated by a red line in Fig. 2). It is also possible to observe in these heterostructures the presence of encapsulated metallic particles (Fig. S1†).
The N content in each sample was determined by elemental analysis and the results are shown in Table 1. The sample E30 showed 94.6% carbon with no nitrogen or hydrogen being detected. The remaining 5.4% is likely related to some oxygen and encapsulated iron particles confined inside the CNTs.
For sample E10A20, the N content increased slightly to 0.4%. On the other hand, for the sample A20E10 the N content reached 5.0%, which further increased to 6.1% for A30. These results were confirmed by EELS analyses (Fig. S2†). A nitrogen content of 5.0–6.1% corresponds to a C/N bulky atomic ratio of ca. 15–20 in the CNT composition. XPS analyses for the sample A30 (Fig. S3†) showed 96/4 C/N ratio suggesting a lower N concentration on the surface. It is known that N-doped nanotube structures show several defects and tends to form arches in the graphene layers as intern caps due to the shorter length of the N–C bonds.15 Nitrogen is preferentially incorporated inside the transversal bended arches in the CNT structure, whereas the curled arches have a relatively low nitrogen incorporation.16 Nitrogen peak deconvolution indicated the presence of 29% pyridinic nitrogen, 31% of pyrrolic nitrogen, 12% of quaternary nitrogen, and 28% of nitrogen oxides.
XRD analyses of the samples showed a slight decrease of the d002 distance with the nitrogen content, i.e. from 3.43 Å for E30 to 3.36 Å for A30 (Fig. S4†). Such a phenomenon has already been reported for N-doped carbon nanostructures.24 DTA analyses under air (Fig. 3, TGA in Fig. S5†) for E30 showed a well-defined peak near 600 °C related to the oxidation of a well-organized carbon structure. In comparison, sample A30 showed an oxidation temperature of ca. 465 °C, which suggests the presence of more defective and reactive carbon. The sample A20E10 presented only a peak at 477 °C, suggesting the presence of a majority of N-doped sections, whereas the sample E10A20 showed two peaks, i.e. one intense peak at ca. 611 °C related to the carbon sections of CNTs and one peak of low intensity at ca. 470 °C related to the N-doped part. From the measured weight loss it is anticipated that the remaining iron content followed the order A30 (∼10.5%) > E10A20 ≈ A20E10 (∼3.5%) > E30 (∼2%). ICP-MS analyses performed on sample E10A20 showed a Fe content of ca. 4.2 wt%.
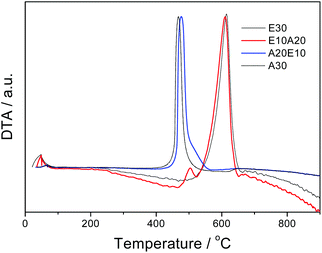 |
| Fig. 3 DTA analyses under air of samples E30, A30, A20E10 and E10A20. | |
BET measurements showed a surface area of 258 m2 g−1 for the samples E30 and E10A20, slightly higher compared to A30 and A20E10 with 195 m2 g−1 surface area (Table 1), in accordance with the larger measured external mean diameter for the nitrogen rich samples.
Raman spectra (Fig. S6†) showed the presence of one strong first order peak at ∼1560 cm−1 (G-band) and three second order features at ∼1330 cm−1 (D-band), ∼2700 cm−1 (G-band) and ∼2940 cm−1, typical of multi-walled CNTs. The second-order peak around 2940 cm−1 is a combination of the D and G bands.25 The ratio of D and G band intensities can be used to evaluate defects in the CNT structures. The influence of nitrogen in the CNTs can also be evaluated by the ratio of G′ and G intensities (Fig. S6†). Two effects can be observed as the N content in the CNTs increases: the ID/IG ratio increases and the relative intensity of the G′ band decreases. These results are consistent with the presence of N in the CNT structure, i.e. there is an increase of defects in the CNT structure in the presence of nitrogen.25–27
Magnetic amphiphilic hybrid carbon nanotube applications
Dispersion tests.
Dispersion tests in a hexane–water biphasic system showed that E30 remains in the hydrophobic phase with poor dispersion. On the other hand, all the N-doped CNTs tend to locate at the hexane–water interface (Fig. 4 for sample A10E20). Under slow stirring, the hybrid CNTs diffuse to the aqueous phase. This good dispersion in water is likely related to the interaction of the N-doped sections with the aqueous phase. Another remarkable feature of the magnetic hybrid CNTs is the possibility to form and break emulsions in a reversible way by simple stirring and magnetic separation (Fig. 4). The tensioactive hybrid CNTs can produce an emulsion from water–hexane biphasic mixtures containing small organic droplets (10–50 μm) surrounded by CNTs in an aqueous matrix (Fig. 4). No emulsification was observed in the presence of E30 for hexane/water even after prolonged sonication.
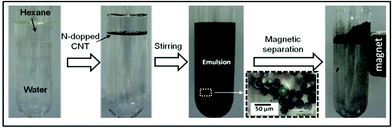 |
| Fig. 4 Photograph of the water–hexane biphasic system, emulsified and after magnetic separation in the presence of the sample A10E20. | |
The hybrid CNTs can also reversibly emulsify different organic phases such as decaline, 1-octene, toluene, and soy bean oil (Fig. S7†). A remarkable feature of this system is that after emulsification, the emulsion can be broken by a simple magnetic separation step. The movement of the magnetic amphiphilic CNTs towards the magnet leads to organic droplet collision, coalescence, collapsing and finally phase separation. The A30 material also emulsifies the hexane–water mixture to some extent, suggesting an amphiphilic behavior. However, the efficiency of the A30 material for emulsification is much lower compared to A20E10 and E10A20. This result suggests that the presence of two separate hydrophilic–hydrophobic sections is important for the emulsification process.
Ethinylestradiol and polyaromatic hydrocarbon adsorption.
This feature of the hybrid N-doped CNTs offers several perspectives for a large variety of applications, especially as adsorbents to remove organic pollutants from an aqueous medium. We present herein preliminary results on the adsorption of two classes of water hazardous contaminants: the hormone ethinylestradiol and 16 different polyaromatic contaminants. Ethinylestradiol is currently considered one of the most hazardous endocrinous disruptor contaminants present in water.28 EE is a relatively large and fairly hydrophobic molecule (solubility in water ca. 5 mg L−1 at room temperature). Common adsorbents based on activated carbon offer limitations due to diffusion of the large EE molecule into the micropores.29 Previous work showed that well dispersed CNTs are efficient adsorbents due to a surface completely exposed and available for EE adsorption.30,31 However, hydrophobic CNTs usually agglomerate in water, strongly decreasing the hormone adsorption.29 In fact, the hydrophobic sample E30 showed a relatively low EE adsorption capacity of 26 mgEE g−1 compared to the sample E10A20, which adsorbed ca. 133 mgEE g−1 (Fig. 5). When the N-content increased to 5–6% (samples A20E10 and A30) leading to a more hydrophilic surface, the EE adsorption decreased. These results suggest that the exposed hydrophobic surface present in part of the N-doped CNTs is crucial for the EE adsorption process. It is very interesting to observe that E10A20 showed EE adsorption capacities even higher than a high surface area (788 m2 g−1) microporous activated carbon. When the normalized adsorption capacities (Fig. 5) are compared, it can be observed that the sample E10A20 shows a much higher adsorption of 502 μgEE m−2 compared to activated carbon (63 μgEE m−2). This result is likely related to the relatively large EE molecule, which cannot adsorb in most of the micropores present in the activated carbon.
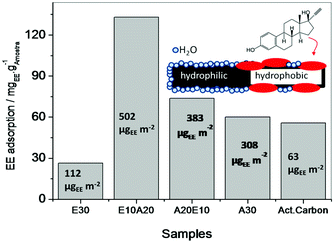 |
| Fig. 5 Ethinylestradiol (EE) adsorption on CNT samples compared with microporous activated carbon. | |
The very efficient adsorption of different PAHs on the amphiphilic N-doped CNTs led to a completely new analytical application as a material for water sampling (Fig. S8†). The CNTs can be easily dispersed in water and efficiently adsorb 16 different PAH contaminants at very low concentrations (Fig. 6, Fig. S9† for a description of each component). The adsorption efficiencies for each sorbent (CNTs and polydimethylsiloxane, PDMS) were calculated by the ratios (area/mass) between the peak areas obtained for each PAH and the mass of the sorbents (2.0 mg of CNT and 1.5 mg of PDMS fiber). After adsorption and pre-concentration, the CNTs can be easily removed from the medium by simple separation with a magnet and the PAHs were extracted with a solvent (Fig. S8†). GC/MS analyses showed that the use of CNTs is more efficient than the classical method that involves PDMS fibers, with much lower solvent consumption, technical simplicity and time with good linearity, determination coefficient and limit of detection (see S10†).
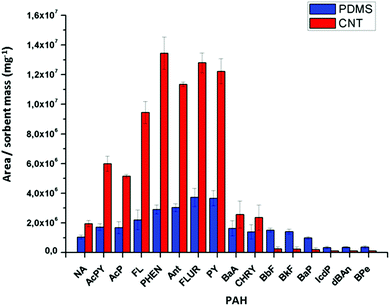 |
| Fig. 6 Ratios between the areas of the chromatographic peaks and masses of sorbents for extraction of 16 PAHs on polydimethylsiloxane and E10A20. | |
Reversible emulsion formation in biphasic systems.
The amphiphilic and magnetic properties of the hybrid CNTs can also be used for reversible emulsion formation in biphasic systems. These properties can be the key for a great variety of biphasic processes relevant to different chemical areas, especially in catalysis. We illustrate herein the use of the hybrid N-doped CNTs to promote three different biphasic reactions: (i) the biphasic oxidative extraction of the nitrogen model petroleum contaminant quinoline, (ii) the biphasic transesterification of soybean oil with methanol and (iii) the metal-catalyzed oxidation of 2-heptanol with molecular oxygen.
Biphasic oxidative extraction of quinolone.
The biphasic oxidative extraction of N-containing petrol contaminants was investigated using a 500 ppm quinoline–hexane (10 mL) solution mixed with 1.6 mL of aqueous H2O2 (1.65 mmol L−1) containing FeCl2·4H2O (5.6 mmol L−1) and formic acid (5 mmol L−1). Quinoline is oxidized using the H2O2/Fe2+/HCOOH system at the oil–water interface to more polar intermediates, which can be removed from the organic phase by extraction to the aqueous phase.6 The results are shown in Fig. 7. Blank experiments performed with H2O2/Fe2+/HCOOH without CNTs showed very limited quinoline oxidation/removal of ca. 10% due to the poor interface. Experiments with only E10A20 (no H2O2) showed no significant removal of quinoline by adsorption on the nanotube alone. Reaction in the presence of the undoped CNTs (E30) showed some emulsification with a limited oxidation/removal of ca. 40%. On the other hand, in the presence of the amphiphilic N-doped CNT the quinoline oxidation/removal significantly increased in the order A30 < A20E10 and reached ca. 100% after 15 min reaction in the presence of the sample E10A20. These results are directly related to the ability of the E10A20 CNTs to efficiently form emulsions. The purified organic phase can then be magnetically separated and the aqueous phase containing the quinoline oxidized derivatives can be treated and properly disposed.
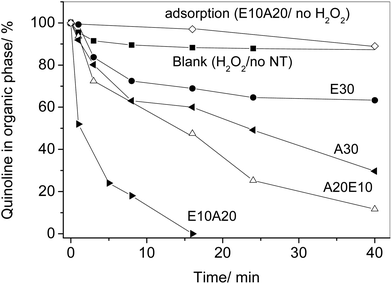 |
| Fig. 7 Biphasic extractive oxidation of the N-containing petrol contaminant quinoline with H2O2. | |
Biphasic transesterification of soybean oil.
The amphiphilic CNTs E10A20 were also tested in the biphasic transesterification of soybean oil with methanol catalyzed by KOH to produce biodiesel. The methanol/KOH and oil mixture was stirred for 3 min at 60 °C and left for 60 additional minutes (Fig. 8). Under the classical homogeneous conditions, the 3 min initial stirring is enough to produce an oil conversion of ca. 80%. However, the reaction stops due to rapid phase separation. If the reaction is stirred for ca. 18 min, the mixture is re-emulsified and the oil conversion increases to 96% after 60 min. Preliminary results in the presence of the E10A20 showed that the reaction mixture remains emulsified even after the stirring is stopped and the oil conversion reaches ca. 100% after only 10 min. Moreover, the presence of the amphiphilic CNTs strongly accelerates the biodiesel purification step carried out by washing with water to remove glycerol, methanol and the catalyst. During this step the emulsion formed with water can be rapidly broken by a simple magnetic separation process (Fig. S11†).
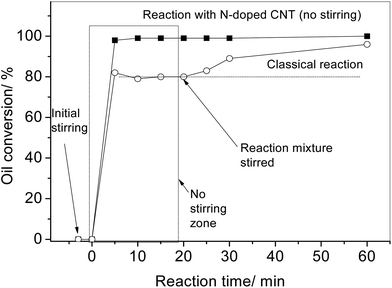 |
| Fig. 8 Transesterification of soybean oil with methanol by the classical homogeneous KOH catalyzed reaction in the presence of the E10A20 CNTs. | |
Oxidation of 2-heptanol with molecular oxygen.
Finally, we investigated the potential of these CNTs as supports for ruthenium or palladium catalysts. Indeed, high metal dispersions have already been achieved on N-doped CNTs.32–35 2 wt% Ru and Pd catalysts were prepared by solvent excess impregnation from Ru(NO)(NO3)x(OH)y and Pd(NO3)2·xH2O, respectively. TEM micrographs and particle size distribution are shown in Fig. 9 for the ruthenium catalysts. It is clear that the Ru dispersion increases with the nitrogen content in the CNTs. The same trend has been observed for the Pd catalysts (Fig. S12†), for which the mean Pd particle size was in the order 4.4 nm (Pd/E30) > 1.4 nm (Pd/E10A20) > 1.3 nm (Pd/A20E10) > 1.2 nm (Pd/A30). For the hybrid structures E10A20 and A20E10, for which the nucleation should arise preferentially on the N-doped sections, a much higher density of metallic nanoparticles is noticed on the hydrophilic sections. The catalytic activity of the Ru/A20E10 and Pd/A20E10 catalysts was evaluated for the oxidation of 2-heptanol in water with molecular oxygen.36,37 Indeed, N-doped carbon supports have been recently proven to be particularly attractive for this reaction.38 The reaction was carried out at 85 °C under 5 bar air. We have checked independently that the hybrid CNT support alone did not show any activity for this reaction. Both Ru and Pd-based catalysts show 100% conversion and 100% selectivity towards 2-heptanone.
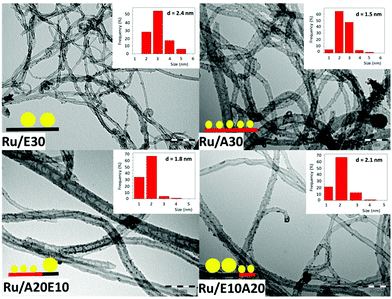 |
| Fig. 9 TEM micrographs and Ru particle size distribution of the 2 wt% Ru catalysts. | |
Conclusions
The results described herein with magnetic amphiphilic N-doped CNTs are very promising for the development of many different applications both in catalysis and adsorption processes. Some of the reactions currently under investigation in our laboratories include biphasic hydroformylation, vegetable oil hydrolysis, fatty acid esterification, isopropanol decomposition, and organic selective oxidations with H2O2.
Acknowledgements
Funding from the CAPES-COFECUB program (Ph715/11) is gratefully acknowledged.
Notes and references
- B. Cornils, Org. Process Res. Dev., 1998, 2, 121–127 CrossRef CAS.
- A. H. Mohammad Fauzi and N. A. S. Amin, Renewable Sustainable Energy Rev., 2012, 16, 5770–5786 CrossRef CAS PubMed.
- R. Quirino and R. Larock, J. Am. Oil Chem. Soc., 2012, 89, 1113–1124 CrossRef CAS PubMed.
- R. Aveyard, B. P. Binks and J. H. Clint, Adv. Colloid Interface Sci., 2003, 100–102, 503–546 CrossRef CAS.
- S. Crossley, J. Faria, M. Shen and D. E. Resasco, Science, 2010, 327, 68–72 CrossRef CAS PubMed.
- I. F. Teixeira, A. A. d. S. Oliveira, T. Christofani and F. C. C. Moura, J. Mater. Chem. A, 2013, 1, 10203–10208 CAS.
- A. P. C. Teixeira, A. D. Purceno, A. S. Barros, B. R. S. Lemos, J. D. Ardisson, W. A. A. Macedo, E. C. O. Nassor, C. C. Amorim, F. C. C. Moura, M. G. Hernández-Terrones, F. M. Portela and R. M. Lago, Catal. Today, 2012, 190, 133–143 CrossRef CAS PubMed.
- A. A. S. Oliveira, I. F. Teixeira, L. P. Ribeiro, J. C. Tristão, A. Dias and R. M. Lago, J. Braz. Chem. Soc., 2010, 21, 2184–2188 CrossRef CAS PubMed.
- A. D. Purceno, A. P. C. Teixeira, N. J. d. Souza, L. E. Fernandez-Outon, J. D. Ardisson and R. M. Lago, J. Colloid Interface Sci., 2012, 379, 84–88 CrossRef CAS PubMed.
- J.-S. Chen, Front. Mater., 2014, 1 CrossRef PubMed.
- O. Stephan, P. M. Ajayan, C. Colliex, P. Redlich, J. M. Lambert, P. Bernier and P. Lefin, Science, 1994, 266, 1683–1685 CAS.
- P. Ayala, R. Arenal, M. Rümmeli, A. Rubio and T. Pichler, Carbon, 2010, 48, 575–586 CrossRef CAS PubMed.
- C. Shan, W. Zhao, X. L. Lu, D. J. O'Brien, Y. Li, Z. Cao, A. L. Elias, R. Cruz-Silva, M. Terrones, B. Wei and J. Suhr, Nano Lett., 2013, 13, 5514–5520 CrossRef CAS PubMed.
- W. H. Shin, H. M. Jeong, B. G. Kim, J. K. Kang and J. W. Choi, Nano Lett., 2012, 12, 2283–2288 CrossRef CAS PubMed.
- A. A. Koós, M. Dowling, K. Jurkschat, A. Crossley and N. Grobert, Carbon, 2009, 47, 30–37 CrossRef PubMed.
- I. Florea, O. Ersen, R. Arenal, D. Ihiawakrim, C. Messaoudi, K. Chizari, I. Janowska and C. Pham-Huu, J. Am. Chem. Soc., 2012, 134, 9672–9680 CrossRef CAS PubMed.
- G.-L. Tian, M.-Q. Zhao, Q. Zhang, J.-Q. Huang and F. Wei, Carbon, 2012, 50, 5323–5330 CrossRef CAS PubMed.
- H. Kim and W. Sigmund, J. Cryst. Growth, 2005, 276, 594–605 CrossRef CAS PubMed.
- J. P. de Mesquita, P. B. Martelli and H. D. F. Gorgulho, J. Braz. Chem. Soc., 2006, 17, 1133–1143 CrossRef CAS PubMed.
- H. F. Gorgulho, J. P. Mesquita, F. Goncalves, M. F. R. Pereira and J. L. Figueiredo, Carbon, 2008, 46, 1544–1555 CrossRef CAS PubMed.
- J. P. O'Byrne, Z. Li, S. L. T. Jones, P. G. Fleming, J. A. Larsson, M. A. Morris and J. D. Holmes, ChemPhysChem, 2011, 12, 2995–3001 CrossRef PubMed.
- R. Yadav, P. Dobal, T. Shripathi, R. Katiyar and O. Srivastava, Nanoscale Res. Lett., 2008, 4, 197–203 CrossRef PubMed.
- M. He, S. Zhou, J. Zhang, Z. Liu and C. Robinson, J. Phys. Chem. B, 2005, 109, 9275–9279 CrossRef CAS PubMed.
- D. Geng, S. Yang, Y. Zhang, J. Yang, J. Liu, R. Li, T.-K. Sham, X. Sun, S. Ye and S. Knights, Appl. Surf. Sci., 2011, 257, 9193–9198 CrossRef CAS PubMed.
- L. G. Bulusheva, A. V. Okotrub, I. A. Kinloch, I. P. Asanov, A. G. Kurenya, A. G. Kudashov, X. Chen and H. Song, Phys. Status Solidi B, 2008, 245, 1971–1974 CrossRef CAS.
- M. S. Dresselhaus, G. Dresselhaus, R. Saito and A. Jorio, Phys. Rep., 2005, 409, 47–99 CrossRef PubMed.
- T. Sharifi, F. Nitze, H. R. Barzegar, C.-W. Tai, M. Mazurkiewicz, A. Malolepszy, L. Stobinski and T. Wågberg, Carbon, 2012, 50, 3535–3541 CrossRef CAS PubMed.
- A. Flores and E. M. Hill, Chemosphere, 2008, 73, 1115–1120 CrossRef CAS PubMed.
- S. A. Snyder, S. Adham, A. M. Redding, F. S. Cannon, J. DeCarolis, J. Oppenheimer, E. C. Wert and Y. Yoon, Desalination, 2007, 202, 156–181 CrossRef CAS PubMed.
- B. Pan, D. Lin, H. Mashayekhi and B. Xing, Environ. Sci. Technol., 2008, 42, 5480–5485 CrossRef CAS.
- L. Joseph, J. Heo, Y.-G. Park, J. R. V. Flora and Y. Yoon, Desalination, 2011, 281, 68–74 CrossRef CAS PubMed.
- L. Mabena, S. Sinha Ray, S. Mhlanga and N. Coville, Appl. Nanosci., 2011, 1, 67–77 CrossRef CAS PubMed.
- B. P. Vinayan and S. Ramaprabhu, Nanoscale, 2013, 5, 5109–5118 RSC.
- Y. Chen, J. Wang, H. Liu, M. N. Banis, R. Li, X. Sun, T.-K. Sham, S. Ye and S. Knights, J. Phys. Chem. C, 2011, 115, 3769–3776 CAS.
- S. Abate, M. Freni, R. Arrigo, M. E. Schuster, S. Perathoner and G. Centi, ChemCatChem, 2013, 5, 1899–1905 CrossRef CAS.
- K. Yamaguchi and N. Mizuno, Angew. Chem., Int. Ed., 2002, 41, 4538–4542 CrossRef CAS.
- T. Mallat and A. Baiker, Chem. Rev., 2004, 104, 3037–3058 CrossRef CAS PubMed.
- P. Zhang, Y. Gong, H. Li, Z. Chen and Y. Wang, Nat. Commun., 2013, 4, 1593 CrossRef PubMed.
Footnote |
† Electronic supplementary information (ESI) available. See DOI: 10.1039/c4nr04005h |
|
This journal is © The Royal Society of Chemistry 2015 |