DOI:
10.1039/C4NJ01719F
(Letter)
New J. Chem., 2015,
39, 94-97
Synthesis of nickel oxalate/zeolitic imidazolate framework-67 (NiC2O4/ZIF-67) as a supercapacitor electrode
Received
(in Montpellier, France)
3rd October 2014
, Accepted 23rd October 2014
First published on 24th October 2014
Abstract
ZIF-67, NiC2O4·2H2O and NiC2O4/ZIF-67 materials are synthesized by a hydrothermal method. The NiC2O4/ZIF-67 electrode delivers a maximum capacitance of 1019.7 F g−1 at a scan rate of 5 mV s−1, and can maintain the specific capacitance of 73% after 2000 cycles in the 6 M KOH electrolyte.
Supercapacitors are important for electrochemical energy storage with a high charge–discharge rate, high capacitance and long cycle life.1,2 According to the charge storage mechanism and active materials utilized, they can be classified as electrochemical double layer capacitors (EDLCs) and pseudocapacitors. The mechanism of EDLCs is attributed to non-faradaic double layer capacitance on the interface between the electrode and electrolyte and the mechanism of pseudocapacitors is ascribed to faradaic charge transfer through redox reactions on the electrode.3 It is well known that electrode materials play a crucial role in the development of high performance supercapacitors in terms of morphology, porosity, and size.4
In recent years, nickel-based compounds as electrode materials have been extensively studied, for example, NiO,5 Ni(OH)2,6 NiMoO4,7 Ni3(NO3)2(OH)4,8 NiC2O4·2H2O (ref. 9) and so on. In particular, NiC2O4·2H2O as an electrode for supercapacitors was studied only by Insoo Jung et al.9 In that paper, cyclic voltammogram results show that nickel oxalate nanostructures are superior to that of nickel oxide nanostructures. But the electrochemical performance was not investigated. Recently, metal–organic frameworks (MOFs) have gained particular attention and have been applied in many fields10–12 as a novel class of nanoporous materials because of their high surface areas, tunable pore sizes and open metal sites. This class of designable framework structures is modularly mainly built from transition-metal clusters as nodes and organic ligands as struts. Up to now, there have been a limited number of reports on MOFs as supercapacitor electrode materials.13–18
In our previous work,19 zeolitic imidazolate framework-8 (ZIF-8) was studied as an electrode for supercapacitors. While ZIF-67 is isostructural to ZIF-8, the only difference is that the transition-metal atom is cobalt.20 So, in this work, NiC2O4/ZIF-67 is synthesized via a typical solvothermal method and investigated as potential electrode materials for supercapacitors with the KOH electrolyte. We hope that the dispersibility and stability of the nickel oxalate can be increased by being supported on the ZIF-67 and to improve the electrochemical performance. A supercapacitor assembled with this NiC2O4/ZIF-67 electrode exhibits a specific gravimetric capacitance of 1019.7 F g−1 at a scan rate of 5 mV s−1 and good cycling performance.
The powder XRD analysis of the crystal structure and purity of the samples are shown in Fig. 1. Some sharp peaks at θ = 5–20° are observed on the XRD pattern of ZIF-67, indicating that a highly crystalline material is achieved. The XRD pattern of the experimental ZIF-67 in this work matches with the patterns from the single crystal data of simulated ZIF-67. The difference of peak intensity is due to the crystal preferred orientation. The XRD pattern shown in Fig. 1d suggests that the obtained sample is nickel oxalate hydroxide [NiC2O4·2H2O] (JCPDS 25-0582). Fig. 1c is the XRD pattern of NiC2O4/ZIF-67, which does not show any other impurity peak. Though the pattern of NiC2O4/ZIF-67 is a little different from the experimental ZIF-67, it is worth noting that the crystalline order of the ZIF-67 host matrix mostly remains unchanged after loading NiC2O4·2H2O, as shown by the comparison of the powder XRD patterns. The morphology of NiC2O4·2H2O and NiC2O4/ZIF-67 are examined by SEM and TEM. As shown in Fig. 2(C) and (D), NiC2O4·2H2O is irregularly dispersed in ZIF-67 and the surfaces of NiC2O4·2H2O are bestrewed with many nanotwigs, forming a nest-like structure and porous morphology. Nitrogen adsorption–desorption isotherms are shown in Fig. 3. The BET surface area of ZIF-67 and NiC2O4/ZIF-67 are 621.4 m2 g−1 and 261.1 m2 g−1, respectively.
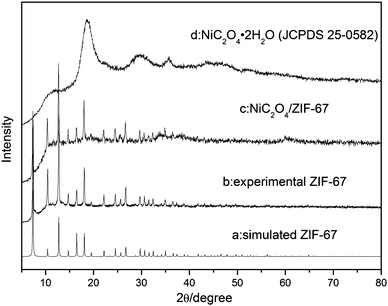 |
| Fig. 1 XRD patterns of all the experimental samples. | |
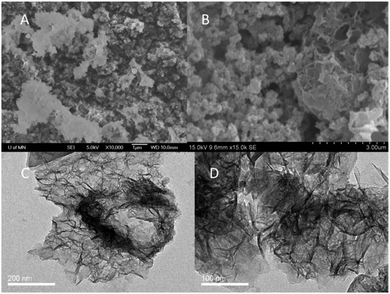 |
| Fig. 2 SEM images of (A) NiC2O4·2H2O and (B) NiC2O4/ZIF-67; TEM images of (C) NiC2O4·2H2O and (D) NiC2O4/ZIF-67. | |
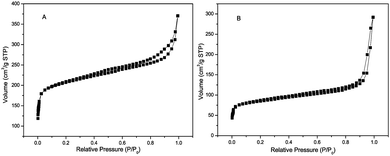 |
| Fig. 3 Nitrogen adsorption–desorption isotherm of (A) ZIF-67 and (B) NiC2O4/ZIF-67. | |
Fig. 4(b)–(d) shows cyclic voltammogram curves (CV) of ZIF-67, NiC2O4·2H2O and NiC2O4/ZIF-67 samples vs. Ag/AgCl as a function of scan rate (5, 10, 20, and 30 mV s−1) in the 6 M KOH electrolyte. Fig. 4(a) shows CV curves of the three samples at a scan rate of 5 mV s−1. In view of the appearance of the symmetrical peak shape in CV curves, the redox supercapacitor of the three samples are very different from the electric double layer capacitor of carbon materials, whose CV curve is almost close to an ideal rectangular shape with a potential-independent current response.21 This indicates that the capacity mainly results from the pseudo-capacitance, which is based on a redox mechanism. ZIF-67 exhibits the ability of ion exchange and redox activity, which may base on the metal ions of Co2+/Co3+.22 The NiC2O4·2H2O electrode presents well-defined redox current peaks, and in line with the reversible reactions:23
2NiC2O4·2H2O(s) ↔ [Ni(C2O4)2]2− Ni2+(aq) |
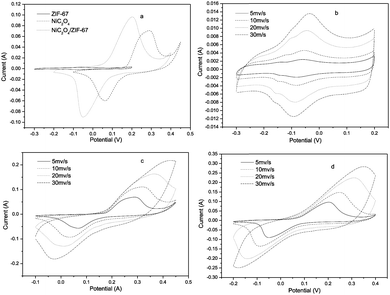 |
| Fig. 4 (a) CV curves of different samples at a scan rate of 5 mV s−1; CV curves at various scan rates of (b) experimental ZIF-67; (c) NiC2O4·2H2O; and (d) NiC2O4/ZIF-67. | |
It should be noted that with the sweep rate increased, the shape of the CV changed, the anodic peaks of the electrodes shift positively, while their cathodic peaks shift negatively, and the capacitance, inevitably, decreased, which is shown in Fig. 5(b). Compared with those for the pure NiC2O4·2H2O electrode, the oxidation and reduction potentials for NiC2O4/ZIF-67 electrodes shift remarkably to the negative direction, which suggests that NiC2O4/ZIF-67 electrodes can be charged more easily. It is probably due to the combination of ZIF-67 with NiC2O4·2H2O, which makes the electron transfer and the intercalation–deintercalation of electrolyte ions easier. The specific capacitances of electrodes at different scan rates are calculated according to the CV curves and the clear relationships are shown in Fig. 5(b). From the CV, the specific capacitance can be estimated as follows:24
|  | (1) |
where
C is the specific capacitance (F g
−1),
v is the scan rate (V s
−1),
m is the mass of the active material (g),
I and
V are the current density (A) and the corresponding voltage (V), respectively. The specific capacitance values for the ZIF-67, NiC
2O
4·2H
2O and NiC
2O
4/ZIF-67 electrodes are 65.5, 870.3 and 1019.7 F g
−1 at a scan rate of 5 mV s
−1, and decrease to 57.8, 526.7 and 573.8 F g
−1 with increasing scan rate to 30 mV s
−1, respectively. Due to the spatial organization of metal ions and organic ligands, ZIF-67 has rationally designed frameworks with nanosized channels and cavities. This type of porous architecture is favorable for an easy to-and-fro diffusion of ions during the charging–discharging process in a capacitor. The NiC
2O
4/ZIF-67 electrode shows much larger specific capacitance than the pure ZIF-67 and NiC
2O
4·2H
2O electrode. This good pseudo-capacitive performance is mainly due to the synergistic electrochemical behavior of ZIF-67 and NiC
2O
4·2H
2O. In the previous introduction, ZIF-67 holds an intersecting three-dimensional structural feature, a large surface area and a large pore size. For these reasons NiC
2O
4·2H
2O may be well dispersed. The results of SEM and TEM confirm this conjecture. Typically, the porous structure is favorable for fast ion/electron transfer. In addition, the branched-like shape of the aggregate structure plays a basic role in the morphology requirement for electrochemical accessibility of OH
- electrolytes to NiC
2O
4·2H
2O active materials and a fast diffusion rate within the redox phase. It is believed that the unique structure provides an important morphological foundation for the high specific capacitance.
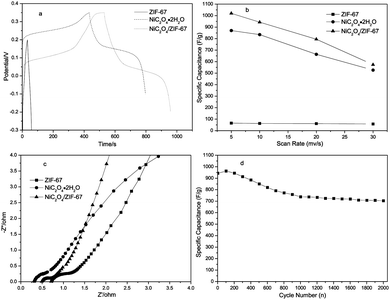 |
| Fig. 5 (a) CP curves of all the experimental samples; (b) the specific capacitance as a function of scan rate of different samples; (c) EIS spectra of all the experimental samples; and (d) cycling performance of NiC2O4/ZIF-67 at a scan rate of 10 mV s−1. | |
The chronopotentiometry (CP) tests of the ZIF-67, NiC2O4·2H2O and NiC2O4/ZIF-67 electrodes are shown in Fig. 5(a) at the current density of 1 A g−1, respectively. The discharge curves of samples are not linear, suggesting the pseudocapacitive behavior of this electrode material, which is in agreement with CV analyses. Since the charge and discharge time of NiC2O4·2H2O and NiC2O4/ZIF-67 electrodes are very long, the curve of the ZIF-67 electrode looks linear. The specific capacitance can be calculated from the CP curve according to eqn (2).25
|  | (2) |
where
C is the specific capacitance,
I is the current, Δ
t is the discharge time, Δ
V is the potential window, and
m is the mass of the electroactive material. Apparently, for the NiC
2O
4/ZIF-67 electrode, the capacitive characteristic is better than the pure ZIF-67 or NiC
2O
4·2H
2O electrode. To further understand the fundamental behavior of the three sample electrodes, EIS analysis is performed and the corresponding Nyquist plots are shown in
Fig. 5(c). Each data point in the Nyquist plot is recorded by changing the frequency. The representation of the impedance data can be carried out using (i) the Nyquist plot,
viz. the imaginary
vs. the real part of the impedance (–
Z′′
vs. Z′); (ii) Bode’ phase angle plot (
θ versus log
ω) and (iii) Bode’ magnitude plot (|
Z|
versus log
ω). The impedance spectra of the samples are almost similar in form with a quasi-semicircle at a higher frequency region and a spike at lower frequency. At high frequency, the solution resistance
Rs and the charge transfer resistance
Rct can be obtained from the Nyquist plot, where the high-frequency semicircle intercepts the real axis at
Rs and (
Rs +
Rct), respectively. From
Fig. 5(c), the
Rs values of the three electrodes decrease in the order of the pure ZIF-67 electrode (0.7 Ω) > the NiC
2O
4/ZIF-67 electrode (0.5 Ω)> the NiC
2O
4·2H
2O electrode (0.3 Ω). And the
Rct of the NiC
2O
4/ZIF-67 electrode (0.5 Ω) are lower than the pure ZIF-67 (1.0 Ω) or NiC
2O
4·2H
2O (1.1 Ω) electrodes. The long-term cycle stability of electrode materials is another critical requirement for practical applications.
Fig. 5(d) depicts the specific capacitance and coulombic efficiency as a function of cycle number plots at a scan rate of 10 mV s
−1 for up to 2000 cycles. The hybrids exhibit a good long-term electrochemical stability with 27% loss after 2000 cycles. These results demonstrate that the as-prepared hybrid is stable as an active electrode material.
In summary, a composite of NiC2O4/ZIF-67 was prepared by a facile and effective hydrothermal approach for electrochemical supercapacitors. The composition, shape and structure were determined by XRD, SEM and TEM. The results of electrochemical tests illustrated that the composite possesses high capacitance (1019.7 F g−1), and over 73% of the original capacitance was retained after repeating the galvanostatic charge–discharge test for 2000 cycles. These results also demonstrate the exciting application potential for high performance as a supercapacitor.
Experimental
Preparation of the electrodes
Synthesis method of ZIF-67 was according to the literature of Junfeng Qian et al.26 The process of NiC2O4/ZIF-67 complexes was synthesized by a typical water solution route. 1 mmol of oxalic acid (H2C2O4) was dissolved in 15 mL of N,N-dimethylacetamide (DMA) to form a homogeneous solution under continuous magnetic stirring. Then, 1 mmol of nickel chloride hexahydrate (NiCl2·6H2O) and ZIF-67 (0.6 g) were introduced into the solution when H2C2O4 was dissolved completely, followed by dropwise adding 10 mL of deionized water. After stirring for 5 min, the as-prepared green-colored precipitate was separated by centrifugation and washed with ethanol several times and dried in a vacuum at 60 °C for 12 h. At last the dried green precipitate was grinded to get the finished product. As a control, NiC2O4·2H2O was also synthesized by the same method as described above, except that there was no ZIF-67 involved.
The working electrode was prepared by mixing 75 wt% of the synthesized active material powder, 20 wt% of acetylene black and 5 wt% of poly(tetrafluoroethylene). After that, the resulting paste was immersed into a nickel foam (1 cm2) served as a current collector under a pressure of 10 MPa. The prepared electrode was dried at 90 °C in a oven for 2 h. The electrode of ZIF-67, NiC2O4·2H2O and NiC2O4/ZIF-67 contained about 4.125, 3.75 and 4.65 mg electroactive materials, respectively, and had a geometric surface area of 1 cm2.
Characterization techniques
Powder XRD data were collected using a Deutschland BRUKER D2 PHASER X-Ray Diffractometer with Cu Kα radiation. The 2θ was scanned in the range of 5–80° with a resolution of 0.02 s−1. Scanning electron microscopy (SEM) analysis (HITACHI S-3400N) was used to capture and determine the morphologies of the samples. The transmission electron microscopy (TEM) images were obtained using a Hitachi H-600 transmission electron microscope at an acceleration voltage of 200 kV. Nitrogen adsorption–desorption measurements for the products were performed using a Micromeritics ASAP 2460 instrument with a degassing temperature of 473 K, and using Brunauer–Emmett–Teller (BET) calculations for the surface area. The pseudocapactive properties of the electrode were studied using cyclic voltammogram (CV), the galvanostatic charge–discharge test and electrochemical impedance spectroscopy (EIS) in 6 M KOH aqueous solution. All the tests were conducted using the CHI660D electrochemical analyzer using a three-electrode configuration with the active material powder electrode as a working electrode, the platinum sheet as a counter electrode and Ag/AgCl as a reference electrode. The EIS measurements were conducted for the working electrode in a frequency range of 100 kHz to 0.01 Hz with ac perturbation of 5 mV.
Acknowledgements
This work was supported by the Fund of Graduate Innovation Project, College of Chemistry and Chemical Engineering, Shanghai University of Engineering Science (E1-0903-14-01106).
Notes and references
- P. Simon and Y. Gogotsi, Nat. Mater., 2008, 7, 845–854 CrossRef CAS PubMed.
- J. R. Miller and P. Simon, Science, 2008, 321, 651–652 CrossRef CAS PubMed.
- B. E. Conway, J. Electrochem. Soc., 1991, 138, 1539–1548 CrossRef CAS PubMed.
- H. Jiang, J. Ma and C. Li, Chem. Commun., 2012, 48, 4465–4467 RSC.
- G. Zhang, L. Yu, H. E. Hoster and X. W. Lou, Nanoscale, 2013, 5, 877–881 RSC.
- D.-L. Fang, Z.-D. Chen, X. Liu, Z.-F. Wu and C.-H. Zheng, Electrochim. Acta, 2012, 81, 321–329 CrossRef CAS PubMed.
- B. Senthilkumar, K. Vijaya Sankar, R. Kalai Selvan, M. Danielle and M. Manickam, RSC Adv., 2013, 3, 352–357 RSC.
- L.-B. Kong, L. Deng, X.-M. Li, M.-C. Liu, Y.-C. Luo and L. Kang, Mater. Res. Bull., 2012, 47, 1641–1647 CrossRef CAS PubMed.
- I. Jung, J. Choi and Y. Tak, J. Mater. Chem., 2010, 20, 6164–6169 RSC.
- Y. Fu, D. Sun, Y. Chen, R. Huang, Z. Ding, X. Fu and Z. Li, Angew. Chem., Int. Ed., 2012, 51, 3364–3367 CrossRef CAS PubMed.
- M. Jahan, Q. Bao and K. P. Loh, J. Am. Chem. Soc., 2012, 134, 6707–6713 CrossRef CAS PubMed.
- H. Bux, F. Liang, Y. Li, J. Cravillon, M. Wiebcke and J. r. Caro, J. Am. Chem. Soc., 2009, 131, 16000–16001 CrossRef CAS PubMed.
- C. Liao, Y. Zuo, W. Zhang, J. Zhao, B. Tang, A. Tang, Y. Sun and J. Xu, Russ. J. Electrochem., 2013, 49, 983–986 CrossRef CAS.
- D. Y. Lee, S. J. Yoon, N. K. Shrestha, S.-H. Lee, H. Ahn and S.-H. Han, Microporous Mesoporous Mater., 2012, 153, 163–165 CrossRef CAS PubMed.
- D. Y. Lee, D. V. Shinde, E.-K. Kim, W. Lee, I.-W. Oh, N. K. Shrestha, J. K. Lee and S.-H. Han, Microporous Mesoporous Mater., 2013, 171, 53–57 CrossRef CAS PubMed.
- R. Díaz, M. G. Orcajo, J. A. Botas, G. Calleja and J. Palma, Mater. Lett., 2012, 68, 126–128 CrossRef PubMed.
- A. Morozan and F. Jaouen, Energy Environ. Sci., 2012, 5, 9269–9290 CAS.
- S.-L. Li and Q. Xu, Energy Environ. Sci., 2013, 6, 1656–1683 CAS.
- Y. Gao, J. Wu, W. Zhang, Y. Tan, J. Zhao and B. Tang, Mater. Lett., 2014, 128, 208–211 CrossRef CAS PubMed.
- R. Banerjee, A. Phan, B. Wang, C. Knobler, H. Furukawa, M. O'Keeffe and O. M. Yaghi, Science, 2008, 319, 939–943 CrossRef CAS PubMed.
- E. Frackowiak and F. Béguin, Carbon, 2001, 39, 937–950 CrossRef CAS.
- S. Horike, D. Umeyama and S. Kitagawa, Acc. Chem. Res., 2013, 46, 2376–2384 CrossRef CAS PubMed.
- J. A. Allen, J. Phys. Chem., 1953, 57, 715–716 CrossRef CAS.
- N. A. M. Barakat, A. G. El-Deen, G. Shin, M. Park and H. Y. Kim, Mater. Lett., 2013, 99, 168–171 CrossRef CAS PubMed.
- B. Hu, X. Qin, A. M. Asiri, K. A. Alamry, A. O. Al-Youbi and X. Sun, Electrochim. Acta, 2013, 107, 339–342 CrossRef CAS PubMed.
- J. Qian, F. Sun and L. Qin, Mater. Lett., 2012, 82, 220–223 CrossRef CAS PubMed.
|
This journal is © The Royal Society of Chemistry and the Centre National de la Recherche Scientifique 2015 |