DOI:
10.1039/C4NJ01316F
(Paper)
New J. Chem., 2015,
39, 246-253
Homoleptic tris-cyclometalated iridium(III) complexes with phenylimidazole ligands for highly efficient sky-blue OLEDs
Received
(in Montpellier, France)
6th August 2014
, Accepted 8th October 2014
First published on 28th October 2014
Abstract
As an extension of our previous study, three sky-blue homoleptic iridium(III) complexes 1–3 with fluorine-free phenylimidazole ligands were synthesized and their photophysical, electrochemical and thermal properties were studied. All the complexes showed high photoluminescence quantum yields ranging from 0.50 to 0.53. The introduction of a bulky isopropyl group at the 2,6-position of N-phenyl of the phenylimidazole ligands increases the quantum yield and the decomposition temperature of the iridium(III) complexes. As the conjugated system of the ligand becomes larger, a very small bathochromic-shift of 1 nm was observed in complex 3 (475 nm) compared with complexes 1 (474 nm) and 2 (474 nm). All the OLED devices showed high current efficiencies of over 20 cd A−1 at the luminance of 1000 cd m−2. Devices incorporated with complexes 1–3 all showed longer lifetime in comparison with that of a FIrpic-based device.
Introduction
Organic light-emitting diodes (OLEDs) have been successfully used in display and solid state lighting since the pioneering work of Tang and VanSlyke in 1987.1 Compared with fluorescent OLEDs, phosphorescent OLEDs using transition metal complexes as emitters can harvest both singlet and triplet excitons, which can achieve an internal quantum efficiency as high as 100%.2–4 Thus, great efforts have been made on the development of transition metal complexes, such as Ir(III),5–13 Os(II),14–17 Ru(II),18–21 Pt(II),22–25 Re(I)26–29 or Cu(I).30–33 Among these phosphorescent emitters, Ir(III) complexes have attracted most attention due to their efficient emission, excellent thermal stability and broadly tunable emission colors at both the molecular and device levels. The emission properties could be attributed to the efficient intersystem crossing between the singlet and triplet excited states caused by the strong spin–orbit coupling of the Ir(III) metal ion.34 These features of Ir(III) complexes also make them very attractive in the fields of sensing,35,36 biolabeling37,38 and catalysis.39 In comparison with the large number of red and green emitters so far discovered and developed, the choices of blue emitting systems used in the OLED research have been based almost solely on bis[(4,6-difluorophenyl)-pyridinate-N,C2]picolinate (FIrpic).40–44 Although a few categories of other materials have been investigated, either the efficiency or the lifetime is not satisfied.7,24,45–52 Usually, the research of the blue iridium(III) complexes is focused on the use of the electron-withdrawing fluorine atom or groups, like F and –CF3, which increase the triplet energy and give rise to the blue shift. In addition, the strong electronegativity of the fluorine atom is believed to destabilize the molecule and shorten the device lifetime.50,53,54 Therefore, the search for the fluorine-free blue emitters is very important and imperative. As a benchmark material in research work, its poor stability has been recently associated with its ancillary ligand, picolinate, which presumably decomposes in devices following an acid-induced degradation55 similar to that of acetylacetonate complexes.56 Generally, homoleptic triscyclometalated iridium(III) complexes have higher chemical stability and longer lifetime than their heteroleptic biscyclometalated counterparts with a labile or vulnerable ancillary ligand, such as picolinate in FIrpic.
Following the initial study of the phenylimidazole-based homoleptic iridium complexes in the patent literature,57–59 we reported highly efficient sky-blue emitting devices with sky-blue tris[1-(2,4-diisopropyldibenzo[b,d]furan-3-yl)-2-phenyl-1H-imidazole]iridium(III) complex (Ir(dbi)3) (Scheme 1) as the phosphorescent emitter.10,60 A maximum current efficiency and an external quantum efficiency (EQE) of 61.5 cd A−1 and 23.1%, respectively, were achieved, which were the highest among those ever reported for blue homoleptic iridium(III) complexes. High efficiencies of 53.5 cd A−1 and 20.1% EQE were achieved even at the luminance of 1000 cd m−2. Very recently, Udagawa and coworkers reported an OLED device with another phenylimidazole based iridium(III) complex as the blue dopant (fac-Ir(mpim)3 in Scheme 1). The blue device has a current efficiency of 75.6 lm W−1 and an EQE of 29.6% at 100 cd m−2.13
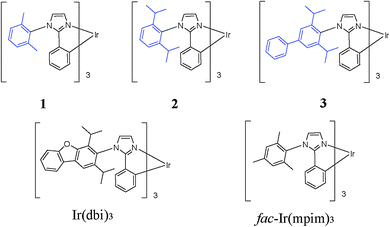 |
| Scheme 1 Chemical structure of the homoleptic iridium(III) complexes 1–3 in comparison with the reported materials Ir(dbi)3 and fac-Ir(mpim)3. | |
In view of the demonstrated high potential of the phenylimidazole based iridium(III) complex for practical blue OLED devices, we report here the synthesis and the physical properties of three structurally similar homoleptic iridium(III) complexes of phenylimidazole-based ligands with different substituents and conjugation degrees (Scheme 1). The OLED devices, with a simple architecture different from those reported before, were fabricated and compared.
Experimental
General information
The phenylimidazole-based ligands 1-(2,6-dimethylphenyl)-2-phenyl-1H-imidazole, 1-(2,6-diisopropylphenyl)-2-phenyl-1H-imidazole, 1-(3,5-diisopropylbiphenyl-4-yl)-2-phenyl-1H-imidazole and tris(acetylacetonate)iridium(III) (Ir(acac)3) were received from SunaTech Inc. All reactants and solvents, unless otherwise stated, were purchased from commercial sources and used as received. 1H NMR and 13C NMR spectra were recorded on a 400 MHz Varian NMR spectrometer in CDCl3 with tetramethylsilane as an internal standard. UV-Vis absorption and photoluminescence (PL) spectra were recorded on a Perkin-Elmer Lambda 750 and a HITACHI F460 fluorescence spectrophotometer, respectively. The quantum efficiency measurements were carried out at room temperature in degassed acetonitrile solutions. Phosphorescence spectra were recorded using an Edinburgh Instruments FLS920 spectrometer. Thermogravimetric analysis (TGA) was undertaken using a SII-EXSTAR 6000 TG/DTA 6200 thermobalance (SII Nano Technology Inc., Tokyo, Japan) under a nitrogen atmosphere by heating the samples from 25 to 750 °C at a heating rate of 10 °C min−1. Elemental analysis was carried out using an EA1110 instrument (Carlo Erba Instruments, Italy). Cyclic voltammetry (CV) was carried out in a three-electrode electrochemical configuration using a platinum working electrode, a platinum auxiliary electrode and an Ag wire pseudo-reference electrode. The potential was referenced to the internal standard ferrocene/ferrocenium (Fc/Fc+) redox couple. The electrochemical cell was loaded with nitrogen-purged DMF and the supporting electrolyte tetrabutylammonium hexafluorophosphate (TBAPF6) in a nitrogen-filled glove box (oxygen and water contents <0.1 ppm) at room temperature. A PARSTAT 2263-2 Advanced Electrochemical System with PowerSUITE software was used for electrochemical measurement and control. The purities of the iridium(III) complexes were determined by high performance liquid chromatography (HPLC) carried out using a HPLC system from Agilent Instruments.
Synthesis
The synthesis of complexes 1–3 followed the same synthetic approach as reported previously for Ir(dbi)3 (Scheme 2). The ligand, Ir(acac)3, and tridecane were mixed in a flask and reacted at 240 °C under nitrogen for 48 hours. The crude product was purified by silica gel column chromatography using hexane–DCM as an eluent.
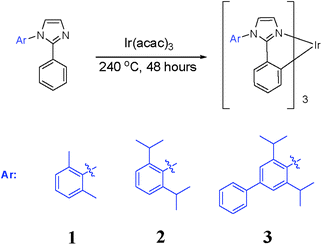 |
| Scheme 2 Synthetic route to complexes 1–3. | |
1. Tris[1-(2,6-dimethylphenyl)-2-phenyl-1H-imidazole]iridium(III) (1).
1-(2,6-Dimethylphenyl)-2-phenyl-1H-imidazole (18.30 g, 73.70 mmol), tris(acetylacetonate)iridium(III) (7.22 g, 14.74 mmol) and tridecane (4.5 mL) were mixed in a 250 mL three-neck oven-dried flask. The flask was purged 3 times with nitrogen, and the reaction mixture was then heated up to 240 °C under nitrogen for 48 hours. The crude product was purified by silica gel column chromatography using hexane–DCM as an eluent to afford the target product. After roto-evaporation, the target product was dried in vacuo (10.05 g, 73%). 1H NMR (400 MHz, CD2Cl2, δ): 7.29–7.22 (t, 3H), 7.18–7.12 (t, 6H), 6.72–6.61 (m, 9H), 6.52–6.46 (t, 3H), 6.34–6.28 (t, 3H), 6.12–6.06 (d, 3H), 2.05–1.98 (s, 9H), 1.83–1.78 (s, 9H); 13C NMR (100 MHz, CD2Cl2, δ): 160.60, 156.94, 137.83, 137.10, 137.02, 136.83, 136.52, 129.85, 129.21, 129.12, 128.40, 126.46, 121.34, 120.22, 119.02, 18.16, 18.05. Anal. calcd for C81H75IrN6O3: C 70.87, H 5.51, N 6.12, found C 70.84, H 5.48, N 6.09. The purity of 1 was confirmed to be 99.3% by HPLC analysis and it was used in PHOLED fabrication without further purification by sublimation.
2. Tris[1-(2,6-diisopropylphenyl)-2-phenyl-1H-imidazole]iridium(III) (2).
1-(2,6-Diisopropylphenyl)-2-phenyl-1H-imidazole (23.00 g, 75.56 mmol), tris(acetylacetonate)iridium (III) (7.40 g, 15.11 mmol) and tridecane (5 mL) were mixed in a 250 mL three-neck oven-dried flask. The flask was purged 3 times with nitrogen, and then the reaction mixture was heated up to 240 °C under a nitrogen atmosphere for 48 hours. The crude product was purified by silica gel column chromatography using hexane–DCM as an eluent to afford the target product. After roto-evaporation, the target product was dried in vacuo (11.99 g, 72%). 1H NMR (400 MHz, CD2Cl2, δ): 7.59–7.50 (t, 3H), 7.40–7.31 (t, 6H), 6.89–6.78 (m, 6H), 6.74–6.66 (m, 3H), 6.62–6.54 (t. 3H), 6.44–6.36 (t, 3H), 6.21–6.13 (m, 3H), 2.80–2.64 (m, 3H), 2.41–2.25 (m, 3H), 1.26–1.18 (m, 9H), 1.07–0.89 (m, 27H); 13C NMR (100 MHz, CD2Cl2, δ): 161.91, 159.87, 148.65, 148.00, 139.17, 137.94, 135.77, 131.87, 129.55, 127.45, 126.35, 126.07, 123.90, 123.32, 119.86, 104.99, 30.34, 30.02, 26.30, 26.05, 24.87, 24.74. The purity of 2 was confirmed to be 99.5% by HPLC analysis and it was used in PHOLED fabrication without further purification by sublimation.
3. Tris[1-(3,5-diisopropylbiphenyl-4-yl)-2-phenyl-1H-imidazole]iridium(III) (3).
1-(3,5-Diisopropylbiphenyl-4-yl)-2-phenyl-1H-imidazole (27.30 g, 71.74 mmol), tris(acetylacetonate)iridium (III) (7.02 g, 14.35 mmol) and tridecane (5 mL) were mixed in a 250 mL three-neck oven-dried flask. The flask was purged 3 times with nitrogen, and then the reaction mixture was heated up to 240 °C under a nitrogen atmosphere for 48 hours. The crude product was purified by silica gel column chromatography using hexane–DCM as an eluent to afford the target product. After roto-evaporation, the target product was dried in vacuo (13.75 g, 72%). 1H NMR (400 MHz, CD2Cl2, δ): 7.79–7.69 (d, 6H), 7.63–7.48 (m, 12H), 7.46–7.39 (t, 3H), 6.92–6.84 (d, 6H), 6.77–6.69 (d, 3H), 6.66–6.59 (t, 3H), 6.50–6.41 (t, 3H), 6.38–6.32 (d, 3H), 2.89–2.70 (m, 3H), 2.50–2.33 (m, 3H), 1.36–1.23 (d, 9H), 1.17–0.90 (m, 27); 13C NMR (100 MHz, CD2Cl2, δ): 159.97, 149.02, 148.35, 144.60, 142.73, 139.28, 138.04, 135.15, 130.70, 129.59, 129.12, 127.59, 125.23, 124.95, 123.99, 123.50, 120.11, 30.54, 30.23, 26.36, 26.10, 24.93, 24.82. The purity of 3 was confirmed to be 99.2% by HPLC analysis and it was used in PHOLED fabrication without further purification by sublimation.
Device fabrication and measurement
OLED devices were fabricated by sequentially depositing organic layers and the metal cathode layer onto an indium tin oxide (ITO) glass substrate using thermal evaporation in one run under high vacuum (∼4.5 × 10−4 Pa). Prior to use, the substrate was degreased with solvents and cleaned in a UV-ozone chamber for 15 min before it was loaded into the evaporation system. The active device area for all the devices was 3 × 3 mm2. The thickness of the deposited layer and the evaporation speed of the individual materials were monitored using quartz crystal microbalance monitors. Electrical testing and optical measurements were performed in air without encapsulation except for the lifetime measurement. The devices for lifetime evaluation were encapsulated immediately after preparation by using epoxy and glass lids. The EL spectra were recorded on a Spectra Scan PR655 spectrometer. The current–voltage (I–V) and luminance–voltage (L–V) relationship were characterized using a computer controlled Keithley 2400 Sourcemeter.
Results and discussion
In order to systematically investigate the three iridium(III) complexes, i.e., 1–3, we conducted studies on their photophysical, electrochemical and thermal properties. Based on these properties, we discuss the relationship between the structure and the properties of the complexes. Device performance including the lifetime is evaluated and compared.
Photophysical properties
The UV-Vis absorption and photoluminescence (PL) spectra of the three complexes shown in Fig. 1 were recorded at room temperature in acetonitrile (CH3CN), the detailed data are summarized in Table 1. The absorption spectrum of all the three complexes shows intense bands in the ultraviolet part of the spectrum ranging from 200 to 320 nm. These bands could be attributed to the spin-allowed 1(π–π*) of the phenylimidazole ligands.34 At the long wavelength of the spectra, the bands from 320 to 450 nm are assigned to both the spin-allowed singlet-to-singlet metal-to-ligand charge-transfer (1MLCT) and spin-forbidden singlet-to-triplet 3MLCT transitions.46 The high intensity of these MLCT bands could be attributed to the effective mixing of these charge transitions caused by the strong spin–orbit coupling of the iridium ion. As demonstrated in Fig. 1, the spectrum of complex 2 is almost the same as complex 1. Since both complexes 1 and 2 share the same backbone of the phenylimidazole based ligand, the similarity of the spectrum indicates that the substitution of methyl and isopropyl groups on the ligand does not change the absorption largely. The absorption intensity of complex 3 is higher than complexes 1 and 2 in the ultraviolet part of the spectrum between 200 and 320 nm. In comparison with complexes 1 and 2, ligand 3 has one more phenyl group, so the conjugated system is larger. Therefore the high molar extinction coefficient of 3 is believed to be caused by the strong spin-allowed 1(π–π*) of the phenylimidazole ligands. The band gaps of the complexes calculated from the absorption edge are 2.77, 2.76 and 2.75 eV, respectively. Though the band gaps are very close, the changing trend is obvious. The detailed data are summarized in Table 1.
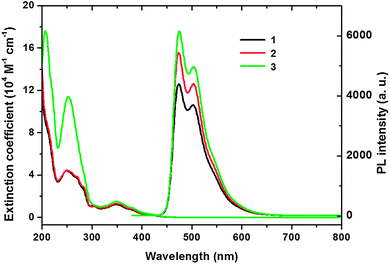 |
| Fig. 1 UV-Vis absorption and PL spectra of 1–3 (each at 1 × 10−5 M in CH3CN, excited at 357 nm) at room temperature. | |
Table 1 Comparison of iridium complexes 1–3 with Ir(dbi)3 and fac-Ir(mpim)3
Complex |
ε (M−1 cm−1)@λ(nm) |
λ
em@λex (nm) |
HOMO (eV) |
LUMO (eV) |
E
ox (V) |
E
g
(eV) |
T
d
(°C) |
Φ
|
Calculated from the absorption edge of UV-vis absorption.
T
d corresponding to 5% weight loss.
The data for Ir(dbi)3 are quoted from ref. 10 for comparison.
The data for fac-Ir(mpim) 3 are quoted from ref. 13 for comparison.
|
1
|
44 178@249, 12 109@348 |
474, 503@357 |
4.92 |
2.15 |
0.12 |
2.77 |
276 |
0.50 |
2
|
44 460@250, 12 879@349 |
474, 503@357 |
4.91 |
2.15 |
0.11 |
2.76 |
363 |
0.53 |
3
|
113 922@252, 14 901@350 |
475, 504@357 |
4.90 |
2.15 |
0.10 |
2.75 |
416 |
0.52 |
Ir(dbi)3c |
103 457@254, 95 115@285, 16 073@349 |
475, 504@357 |
4.95 |
2.21 |
0.15 |
2.74 |
429 |
0.52 |
fac-Ir(mpim) 3d |
|
474@353 |
|
|
|
|
412 |
|
The photoluminescence emission quantum yields (Φ) of the three complexes were measured in deaerated acetonitrile (CH3CN) solution using fac-tris[2-phenylpyridinato]iridium(III) (Ir(ppy)3, Φ = 0.4) as a reference. The emission quantum yields in degassed solutions are very high, ranging from 0.50 to 0.53. As shown in Fig. 1, the PL intensity of the complexes increases from complex 1 to 3. Since the absorption of 1 and 2 are very similar, the high emission quantum yield is expected for complex 2. The emission quantum yield of complex 1 is 0.50, while complex 2 was measured to be as high as 0.53. For complex 3, an emission quantum yield of 0.52 was obtained. As for the molecular structure, complexes 1 and 2 differ only in the substituent, i.e., methyl for complex 1 and isopropyl for complex 2. Both methyl and isopropyl groups belong to the electron donating group, but do not have much difference in the electron donating capability. The slightly higher quantum yield of complex 2 could be attributed to the larger steric hindrance of the substituted isopropyl group, which will limit the molecular, vibrational and rotational motions. Complex 3 has the emission quantum yield of 0.52, which is close to the value of complex 2. The increased PL intensity could be attributed to the stronger absorption of complex 3.
Unlike the absorption, the emission spectra of the three complexes have almost the same features, i.e., a main peak at around 474 nm and a shoulder at around 503 nm. The vibronic structure of the emission bands comes from the mixing of the ligand centered and the MLCT state.51 The main peak and the shoulder peak of complexes 1 and 2 are exactly the same, which are at 474 and 503 nm, respectively. The peak emission of the reported material fac-Ir(mpim)3, which has a similar structure as complex 1 (Scheme 1), is also at 474 nm. A very small bathochromic-shift was observed as the ligand conjugation becomes larger. Both the main peak spectra of complex 3 and Ir(dbi)3 are at 475 nm, which are 1 nm red-shifted relative to complexes 1 and 2. Also, a 1 nm red-shift was found in the shoulder peak for complex 3 and Ir(dbi)3. Therefore, in order to obtain deep blue tris-cyclometalated iridium(III) emitters, the conjugated system of the ligand should be carefully designed.
Electrochemical properties
The electrochemical properties of the tris-cyclometalated iridium(III) complexes were investigated by cyclic voltammetry in DMF solution containing 0.1 M tetra-butylammonium hexafluorophosphate (TBAPF6) as the supporting electrolyte. The voltammograms are demonstrated in Fig. 2, and the detailed numerical data are summarized in Table 1. As shown in Fig. 2, all the complexes exhibited a reversible oxidation wave, with the potentials ranging from 0.10 V to 0.12 V.
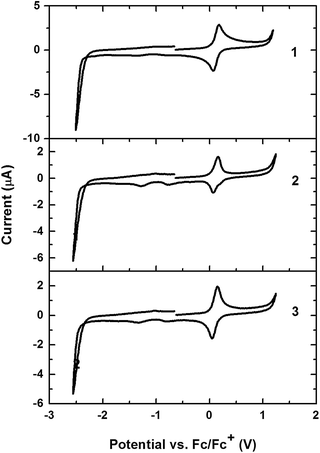 |
| Fig. 2 Cyclic voltammograms of 1–3 (2 × 10−3 M in DMF, 0.1 M of TBAPF6, scan rate 100 mV s−1). | |
Thermal properties
The thermal properties of the iridium(III) complexes were investigated by thermogravimetric analysis (TGA), the TGA curves are displayed in Fig. 3. The decomposition temperature (Td) of the complexes is in the range of 276 to 429 °C, defined as the temperature at which 5% weight loss occurs. In comparison with complex 1, Td of complex 2 is much higher. As the alkyl substituents change from methyl to isopropyl, the decomposition temperature of complex 2 increases by nearly 100 °C. The increased Td value of complex 2 indicates that the size and steric hindrance of isopropyl is beneficial for thermal stability of the transition-metal complex. The ligand of complex 3 has one more benzene ring, so a higher decomposition temperature was expected compared with complex 2. The detailed thermal data are summarized in Table 1.
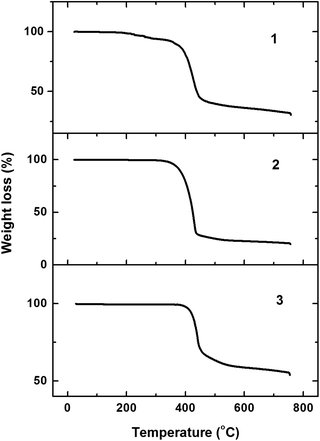 |
| Fig. 3 TGA curves of 1–3 (10 °C min−1 from 25 to 750 °C). | |
OLED performance
The electroluminescence properties of these homoleptic triscyclometalated iridium(III) complexes were evaluated using fabricated OLED devices that have the following configuration: ITO/TAPC (30 nm)/iridium(III) complex, CTPO 10–15 wt% (30 nm)/BCP (30 nm)/LiF (1 nm)/Al, where TAPC is 1,1-bis[(di-4-tolylamino)phenyl]cyclohexane, CTPO is 9-(3-(5-(4-(diphenylphosphoryl)phenyl)-4-phenyl-4H-1,2,4-triazol-3-yl)phenyl)-9H-carbazole, and BCP is 2,9-dimethyl-4,7-diphenyl-1,10-phenanthroline. TAPC was used as the hole transport material, CTPO as the host material and BCP as the electron transport material. Devices 1, 2 and 3 correspond to the devices based on complexes 1, 2 and 3, respectively. Fig. 4 shows the device architectures and the chemical structures of the used materials.
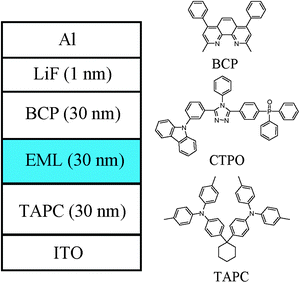 |
| Fig. 4 Structure of OLED devices and the materials used. EML: CTPO doped with 1–3. | |
The current density–voltage–luminance (J–V–L) and the current efficiency–voltage–power efficiency (CE–V–PE) characteristics are illustrated in Fig. 5. The EL spectra of all the devices are similar to the photoluminescence of the complexes in solution. Interestingly, the EL spectra peaks are all at 472 nm, which is the same as the devices using FIrpic as the emitter. All the device performances are summarized in Table 2. For each complex, the concentration was optimized and the doping concentrations are 12 wt%, 14 wt% and 10 wt%, respectively. Devices based on complexes 1–3 showed peak current and power efficiency of 31.1 cd A−1 & 17.4 lm W−1, 35.7 cd A−1 & 28.2 lm W−1, and 31.0 cd A−1 & 26.5 lm W−1, respectively. The current efficiencies all remained higher than 20 cd A−1 even at the high luminance of 1000 cd m−2, which are 21.6, 25.5, and 29.2 cd A−1, respectively. The higher current and power efficiencies achieved for complex 3 (Φ = 0.53) could be attributed to its higher quantum yield compared with complexes 1 (Φ = 0.50) and 2 (Φ = 0.52).
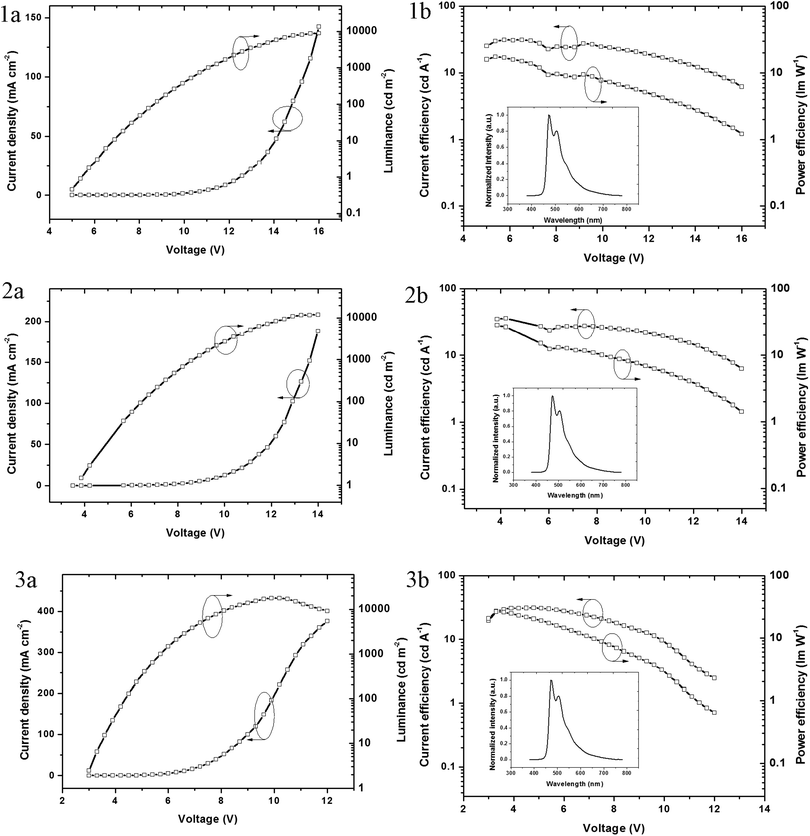 |
| Fig. 5
J–V–L and CE–V–PE characteristics of the devices based on iridium(III) complexes 1–3. Inset in 1b, 2b and 3b: EL spectra of the device. | |
Table 2 EL data for devices based on iridium(III) complexes 1–3a
Complex |
100 cd m−2 |
1000 cd m−2 |
Maximum CE |
Maximum PE |
CIE (x, y) |
Voltage |
CE |
PE |
Voltage |
CE |
PE |
Voltage |
CE |
Voltage |
PE |
Voltage: V; CE: cd A−1; PE: lm W−1.
|
1
|
8.6 |
24.3 |
8.8 |
11.0 |
21.6 |
6.1 |
6.5 |
31.1 |
5.4 |
17.4 |
0.19, 0.39 |
2
|
6.4 |
26.6 |
12.4 |
8.6 |
25.5 |
7.9 |
4.2 |
35.7 |
3.8 |
28.2 |
0.20, 0.38 |
3
|
4.3 |
30.7 |
27.4 |
5.6 |
29.2 |
21.3 |
4.8 |
31.0 |
3.3 |
26.5 |
0.19, 0.38 |
In order to investigate the charge transport ability of the complexes with different phenylimidazole-based ligands, devices with the structure of the ITO/TAPC (30 nm)/iridium(III) complex, CTPO 10 wt% (30 nm)/BCP (30 nm)/LiF (1 nm)/Al, were fabricated. As shown in Fig. 6, over the voltage range from 0 V to 14 V, the current densities are almost the same for the devices based on 1–3, indicating that the differences in the ligands barely affect the charge transport ability of the iridium(III) complexes.
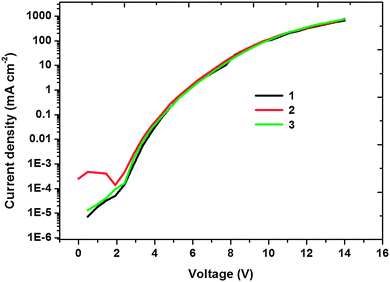 |
| Fig. 6 Current density–voltage characteristics of the devices based on 1–3. The device based on 4 is given as a reference. | |
At the luminance of 100 and 1000 cd m−2, the OLED devices based on different emitters have different working voltages. As shown in Fig. 7, the working voltage dropped quickly from 1 to 3. Since complexes 1–3 have nearly the same charge transport ability, the difference in the working voltages could be attributed to radiative transition of the excitons.
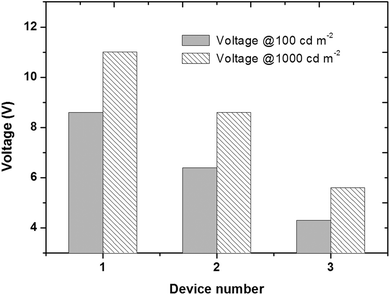 |
| Fig. 7 Operating voltage of the devices at the luminance of 100 and 1000 cd m−2. | |
Lifetime measurement
The stability of complexes 1–3 at the device level is evaluated by measuring the lifetime T50 (in hour), which is defined as the time when the luminance of an OLED device is reduced to half of its initial value during the test. As demonstrated in the luminance–time profile in Fig. 8, the luminance of devices based on 1–3 show almost the same decay bahavior. The T50 values of devices based on complexes 1–3 are 4.9, 5.2 and 4.6 hours, respectively. For comparison, the decay of the FIrpic-based device is also presented in Fig. 8. Although the lifetimes were assessed under simple encapsulation conditions, the big difference in lifetime (∼5 h for devices based on complexes 1–3vs. ∼0.1 h for the FIrpic-based device) does indicate a much higher stability of the devices based on complexes 1–3 relative to the FIrpic-based device. There are a number of materials used in the construction of the OLED devices, but the excellent molecular stability of the homoleptical cyclometalated iridium(III) complexes is certainly the reason for the big difference in the device lifetimes.
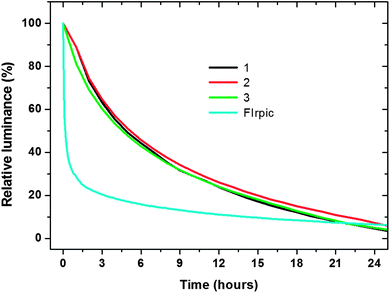 |
| Fig. 8 Luminance–time characteristics of the devices with an initial luminance of around 1000 cd m−2. | |
Conclusions
In summary, we have synthesized three tris(phenylimidazole) cyclometalated iridium(III) complexes 1–3. The photophysical, electrochemical and thermal properties as well as the OLED performance were characterized. The complexes with bulky substituents, like the isopropyl group, have higher quantum yield than complexes with methyl groups. A red-shift was observed in PL spectra as the conjugated system of the ligand becomes larger. Together with a number of previous reports, this work proves that the material properties and device performance are consistently linked to the conjugation degree of the phenylimidazole ligands and that the phenylimidazole based fluorine-free homoleptic iridium(III) complexes are very promising sky-blue emitters for phosphorescent OLEDs.
Acknowledgements
This work was supported by the project of the Major Research plan of the National Natural Science Foundation of China (Grant No. 91123034, 21005084, 20902066 and 21402233), Strategic Priority Research Program of the Chinese Academy of Sciences (Grant No. XDA09020201) and a Project supported by the National Science and Technology Ministry (Grant No. 2012BAF13B05-402). The authors thank the Natural Science Foundation of Jiangsu Province (BK2012631 and BK20140387) and the Open Fund of the State Key Laboratory of Luminescent Materials and Devices (South China University of Technology, 2012-skllmd-05) for financial support. The authors also thank Zezhu Huang, Xuezhen Ji, Liqiang Zeng and Fanzhen Kong of SunaTech Inc. for the syntheses of complexes 1–3.
References
- C. W. Tang and S. A. VanSlyke, Appl. Phys. Lett., 1987, 51, 913 CrossRef CAS.
- M. A. Baldo, D. F. O'Brien, Y. You, A. Shoustikov, S. Sibley, M. E. Thompson and S. R. Forrest, Nature, 1998, 395, 151 CrossRef CAS.
- C. Adachi, M. A. Baldo, M. E. Thompson and S. R. Forrest, J. Appl. Phys., 2001, 90, 5048 CrossRef CAS.
- M. A. Baldo, S. Lamansky, P. E. Burrows, M. E. Thompson and S. R. Forrest, Appl. Phys. Lett., 1999, 75, 4 CrossRef CAS.
- S. Lamansky, P. Djurovich, D. Murphy, F. Abdel-Razzaq, R. Kwong, I. Tsyba, M. Bortz, B. Mui, R. Bau and M. E. Thompson, Inorg. Chem., 2001, 40, 1704 CrossRef CAS PubMed.
- A. Tsuboyama, H. Iwawaki, M. Furugori, T. Mukaide, J. Kamatani, S. Igawa, T. Moriyama, S. Miura, T. Takiguchi, S. Okada, M. Hoshino and K. Ueno, J. Am. Chem. Soc., 2003, 125, 12971 CrossRef CAS PubMed.
- T. Sajoto, P. I. Djurovich, A. B. Tamayo, J. Oxgaard, W. A. Goddard and M. E. Thompson, J. Am. Chem. Soc., 2009, 131, 9813 CrossRef CAS PubMed.
- J. M. Fernández-Hernández, J. I. Beltrán, V. Lemaur, M.-D. Gálvez-López, C.-H. Chien, F. Polo, E. Orselli, R. Fröhlich, J. Cornil and L. De Cola, Inorg. Chem., 2013, 52, 1812 CrossRef PubMed.
- F. Kessler, Y. Watanabe, H. Sasabe, H. Katagiri, M. K. Nazeeruddin, M. Gratzel and J. Kido, J. Mater. Chem. C, 2013, 1, 1070 RSC.
- J. Zhuang, W. Li, W. Su, Y. Liu, Q. Shen, L. Liao and M. Zhou, Org. Electron., 2013, 14, 2596 CrossRef CAS.
- J. Frey, B. F. E. Curchod, R. Scopelliti, I. Tavernelli, U. Rothlisberger, M. K. Nazeeruddin and E. Baranoff, Dalton Trans., 2014, 43, 5667 RSC.
- T. Giridhar, T.-H. Han, W. Cho, C. Saravanan, T.-W. Lee and S.-H. Jin, Chem. – Eur. J., 2014, 20, 8260 CrossRef CAS PubMed.
- K. Udagawa, H. Sasabe, C. Cai and J. Kido, Adv. Mater., 2014, 26, 5062 CrossRef CAS PubMed.
- B. Carlson, G. D. Phelan, W. Kaminsky, L. Dalton, X. Jiang, S. Liu and A. K. Y. Jen, J. Am. Chem. Soc., 2002, 124, 14162 CrossRef CAS PubMed.
- Y.-L. Tung, P.-C. Wu, C.-S. Liu, Y. Chi, J.-K. Yu, Y.-H. Hu, P.-T. Chou, S.-M. Peng, G.-H. Lee, Y. Tao, A. J. Carty, C.-F. Shu and F.-I. Wu, Organometallics, 2004, 23, 3745 CrossRef CAS.
- C.-H. Lin, C.-W. Hsu, J.-L. Liao, Y.-M. Cheng, Y. Chi, T.-Y. Lin, M.-W. Chung, P.-T. Chou, G.-H. Lee, C.-H. Chang, C.-Y. Shih and C.-L. Ho, J. Mater. Chem., 2012, 22, 10684 RSC.
- S.-H. Chang, C.-F. Chang, J.-L. Liao, Y. Chi, D.-Y. Zhou, L.-S. Liao, T.-Y. Jiang, T.-P. Chou, E. Y. Li, G.-H. Lee, T.-Y. Kuo and P.-T. Chou, Inorg. Chem., 2013, 52, 5867 CrossRef CAS PubMed.
- K. M. Maness, R. H. Terrill, T. J. Meyer, R. W. Murray and R. M. Wightman, J. Am. Chem. Soc., 1996, 118, 10609 CrossRef CAS.
- E. S. Handy, A. J. Pal and M. F. Rubner, J. Am. Chem. Soc., 1999, 121, 3525 CrossRef CAS.
- Y. L. Tung, S. W. Lee, Y. Chi, L. S. Chen, C. F. Shu, F. I. Wu, A. J. Carty, P. T. Chou, S. M. Peng and G. H. Lee, Adv. Mater., 2005, 17, 1059 CrossRef CAS.
- H. Xia, M. Li, D. Lu, C. B. Zhang, W. J. Xie, X. D. Liu, B. Yang and Y. G. Ma, Adv. Funct. Mater., 2007, 17, 1757 CrossRef CAS.
- J. Brooks, Y. Babayan, S. Lamansky, P. I. Djurovich, I. Tsyba, R. Bau and M. E. Thompson, Inorg. Chem., 2002, 41, 3055 CrossRef CAS PubMed.
- W. Lu, B.-X. Mi, M. C. W. Chan, Z. Hui, C.-M. Che, N. Zhu and S.-T. Lee, J. Am. Chem. Soc., 2004, 126, 4958 CrossRef CAS PubMed.
- Z. M. Hudson, C. Sun, M. G. Helander, Y.-L. Chang, Z.-H. Lu and S. Wang, J. Am. Chem. Soc., 2012, 134, 13930 CrossRef CAS PubMed.
- E. Rossi, A. Colombo, C. Dragonetti, D. Roberto, R. Ugo, A. Valore, L. Falciola, P. Brulatti, M. Cocchi and J. A. G. Williams, J. Mater. Chem., 2012, 22, 10650 RSC.
- Y. Li, Y. Liu, J. Guo, F. Wu, W. Tian, B. Li and Y. Wang, Synth. Met., 2001, 118, 175 CrossRef CAS.
- F. Li, M. Zhang, J. Feng, G. Cheng, Z. Wu, Y. Ma, S. Liu, J. Sheng and S. T. Lee, Appl. Phys. Lett., 2003, 83, 365 CrossRef CAS.
- B. Li, M. Li, Z. Hong, W. Li, T. Yu and H. Wei, Appl. Phys. Lett., 2004, 85, 4786 CrossRef CAS.
- Z. Si, J. Li, B. Li, F. Zhao, S. Liu and W. Li, Inorg. Chem., 2007, 46, 6155–6163 CrossRef CAS PubMed.
- Q. Zhang, Q. Zhou, Y. Cheng, L. Wang, D. Ma, X. Jing and F. Wang, Adv. Mater., 2004, 16, 432 CrossRef CAS.
- G. Che, Z. Su, W. Li, B. Chu, M. Li, Z. Hu and Z. Zhang, Appl. Phys. Lett., 2006, 89, 103511 CrossRef.
- M. Hashimoto, S. Igawa, M. Yashima, I. Kawata, M. Hoshino and M. Osawa, J. Am. Chem. Soc., 2011, 133, 10348 CrossRef CAS PubMed.
- Q. Zhang, T. Komino, S. Huang, S. Matsunami, K. Goushi and C. Adachi, Adv. Funct. Mater., 2012, 22, 2327 CrossRef CAS.
- A. B. Tamayo, B. D. Alleyne, P. I. Djurovich, S. Lamansky, I. Tsyba, N. N. Ho, R. Bau and M. E. Thompson, J. Am. Chem. Soc., 2003, 125, 7377 CrossRef CAS PubMed.
- G. Di Marco, M. Lanza, M. Pieruccini and S. Campagna, Adv. Mater., 1996, 8, 576 CrossRef CAS.
- Q. Zhao, T. Cao, F. Li, X. Li, H. Jing, T. Yi and C. Huang, Organometallics, 2007, 26, 2077 CrossRef CAS.
- X. Wang, J. Jia, Z. Huang, M. Zhou and H. Fei, Chem. – Eur. J., 2011, 17, 8028 CrossRef CAS PubMed.
- H. Wu, T. Yang, Q. Zhao, J. Zhou, C. Li and F. Li, Dalton Trans., 2011, 40, 1969 RSC.
- T. Suzuki, Chem. Rev., 2011, 111, 1825 CrossRef CAS PubMed.
- C. Adachi, R. C. Kwong, P. Djurovich, V. Adamovich, M. A. Baldo, M. E. Thompson and S. R. Forrest, Appl. Phys. Lett., 2001, 79, 2082 CrossRef CAS.
- R. J. Holmes, S. R. Forrest, Y.-J. Tung, R. C. Kwong, J. J. Brown, S. Garon and M. E. Thompson, Appl. Phys. Lett., 2003, 82, 2422 CrossRef CAS.
- S. Tokito, T. Iijima, Y. Suzuri, H. Kita, T. Tsuzuki and F. Sato, Appl. Phys. Lett., 2003, 83, 569 CrossRef CAS.
- S. J. Yeh, M. F. Wu, C. T. Chen, Y. H. Song, Y. Chi, M. H. Ho, S. F. Hsu and C. H. Chen, Adv. Mater., 2005, 17, 285 CrossRef CAS.
- H. Sasabe, K. Minamoto, Y.-J. Pu, M. Hirasawa and J. Kido, Org. Electron., 2012, 13, 2615 CrossRef CAS.
- K. Dedeian, J. Shi, E. Forsythe, D. C. Morton and P. Y. Zavalij, Inorg. Chem., 2006, 46, 1603 CrossRef PubMed.
- E. Orselli, G. S. Kottas, A. E. Konradsson, P. Coppo, R. Fröhlich, L. De Cola, A. van Dijken, M. Büchel and H. Börner, Inorg. Chem., 2007, 46, 11082 CrossRef CAS PubMed.
- L. S. Liao and K. P. Klubek, Appl. Phys. Lett., 2008, 92, 223311 CrossRef.
- K. S. Yook, S. O. Jeon, C. W. Joo and J. Y. Lee, Org. Electron., 2009, 10, 170 CrossRef CAS.
- S. O. Jeon, S. E. Jang, H. S. Son and J. Y. Lee, Adv. Mater., 2011, 23, 1436 CrossRef CAS PubMed.
- K. S. Yook and J. Y. Lee, Adv. Mater., 2012, 24, 3169 CrossRef CAS PubMed.
- C.-H. Yang, M. Mauro, F. Polo, S. Watanabe, I. Muenster, R. Fröhlich and L. De Cola, Chem. Mater., 2012, 24, 3684 CrossRef CAS.
- C. Fan, Y. Li, C. Yang, H. Wu, J. Qin and Y. Cao, Chem. Mater., 2012, 24, 4581 CrossRef CAS.
- R. J. Holmes, S. R. Forrest, T. Sajoto, A. Tamayo, P. I. Djurovich, M. E. Thompson, J. Brooks, Y.-J. Tung, B. W. D'Andrade, M. S. Weaver, R. C. Kwong and J. J. Brown, Appl. Phys. Lett., 2005, 87, 243507 CrossRef.
- R. Seifert, I. Rabelo de Moraes, S. Scholz, M. C. Gather, B. Lüssem and K. Leo, Org. Electron., 2013, 14, 115 CrossRef CAS.
- E. Baranoff, B. F. E. Curchod, J. Frey, R. Scopelliti, F. Kessler, I. Tavernelli, U. Rothlisberger, M. Grätzel and M. K. Nazeeruddin, Inorg. Chem., 2012, 51, 215 CrossRef CAS PubMed.
- Y. Li, Y. Liu and M. Zhou, Dalton Trans., 2012, 41, 3807 RSC.
-
P. B. M. Chun Lin, R. W. Walters, J.-y. Tsai, C. Brown and J. Deng, WO 2006121811 A1, 2006.
-
W. Y. Chuanjun Xia, B. Ma, S. Beers, J.-y. Tsai, J. Fiordeliso, E. Barron and C. Lin, US 20110057559 A1, 2011.
-
C. Xia and D. Rayabarapu, US 20110204333 A1, 2011.
- J. Zhuang, W. Li, W. Su, M. Zhou and Z. Cui, New J. Chem., 2014, 38, 650 RSC.
|
This journal is © The Royal Society of Chemistry and the Centre National de la Recherche Scientifique 2015 |
Click here to see how this site uses Cookies. View our privacy policy here.