DOI:
10.1039/C4NJ01132E
(Paper)
New J. Chem., 2015,
39, 287-294
One-step synthesis of structure controlled vinyl functionalized hollow mesoporous silica nanospheres
Received
(in Montpellier, France)
8th July 2014
, Accepted 11th October 2014
First published on 28th October 2014
Abstract
In the present study, vinyl functionalized hollow mesoporous silica nanospheres (V-HMSNSs) with tailored mesostructure have been successfully synthesized via a one-step synthetic strategy using the cation surfactant cetyltrimethylammonium bromide (CTAB) as a single micelle template, vinyltriethoxysilane (VTES) as a precursor. It was demonstrated, for the first time, that V-HMSNSs with controllable mesostructure can be easily obtained by adjusting the concentration of CTAB in aqueous solution at low amounts of ammonia. Only solid spheres including vinyl functional groups were generated below the critical micelle concentration value (CMC, 0.0009 M) of CTAB. However, the nanospheres or nanorods with hollow mesostructure were obtained at this value or above it (0.005 M). Whereas no particles were obtained at a higher concentration of CTAB (0.008 M or more than it). It was suggested that the formation of CTAB micelles was closely related to the formation of V-HMSNSs. In addition, the V-HMSNSs with well-defined mesostructure could be obtained when the volume ratio of alcohol to water was about 25
:
25. More importantly, from these investigations, it appeared that the synthetic method should be highly flexible and enable uniform distribution of functional groups within mesoporous silica frameworks.
Introduction
There has been growing interest in the past decade in fabricating organic–inorganic hybrid silica materials, among these hybrid materials, a typical and important class of silica materials is hollow mesostructure silica material. So far, hollow mesoporous hybrid silica materials with various nanostructures have been synthesized, such as nanospheres, nanotubes or nanorods and other nanoporous structures, etc., due to their low density, low toxicity, high biocompatibility, large specific surface areas and excellent thermal and mechanical stability, and thus have been widely applied in the fields of catalysis, separation/adsorption, biomedicine and controlled release of drugs.1–7
The discovery of the M41S silica families by Mobil scientists in 1992 opened up an era of new inorganic materials with ordered mesopore structure by using organic templates as structure-directing agents.8,9 On this basis, for more than several years, many researchers have applied themselves to investigating the organic functionalized hollow mesoporous silica nanospheres (OHMSNs). Compared with traditionally inorganic silica hollow mesoporous nanospheres, OHMSNs combine the advantages of organic chemistry and inorganic building blocks. Except that they are more active and more easily modified by chemical transformation of the organic groups in the hybrid silica networks.10–15 Especially, the preparation of OHMSNs has attracted much attention because of their excellent properties and potential applications. By now, there are two common synthesis approaches for OHMSNs, called post-grafting and co-condensation synthesis.16–26 In the former method, organic groups were directly grafted onto the pore surface of pure hollow mesoporous silica materials. It may often lead to a nonhomogeneous distribution of the organic groups within the pores and a lower degree of occupation. In extreme cases (e.g., with very bulky grafting species), complete closure of the pores can be caused (pore blocking). In another method, co-condensation synthesis, also known as direct synthesis or one pot synthesis, is an alternative method, involving the simultaneous condensation of corresponding silica and organosilica precursors.16,20,27–29 However, in this case, the content of organic functionalities in the mixture does not exceed 40 mol%, furthermore, the proportion of terminal organic groups that are incorporated into the pore-wall network is lower than would correspond to the starting concentration of the reaction mixture.
In this study, a facile and low cost synthetic strategy has been adopted for preparation of OHMSNs. To our knowledge, the key point of this method lies in organic functional groups existing in the entire silica networks including the inner core and the outer shell. And more importantly, compared with the former two methods it offers a higher and more uniform surface coverage of functional groups and a better control of the surface properties of the resultant materials. In this paper, structure controlled vinyl functionalized hollow mesoporous silica nanospheres (V-HMSNSs) were successfully synthesized via a one-step method using CTAB as a template and VTES as a precursor. It was found that the concentration of CTAB plays an important role in the mesostructure and morphology formation of the samples at pure water solution.
Experimental section
Chemicals and reagents
Vinyltriethoxysilane (VTES, 99%) was purchased from Aladdin. Aqueous ammonia solution (28 wt%), cetyltrimethylammonium bromide (CTAB), and other reagents were obtained from Sinopharm Chemical Reagent Company Ltd (China). All materials were of analytical grade and were used without further purification. Deionized water was prepared in our laboratory.
Synthesis procedure
Synthesis of V-HMSNSs in aqueous solution using ammonia as the catalyst.
In a typical synthesis procedure, 0.016 g, 0.05 g, 0.1 g, and 0.2 g of CTAB and 1 mL, 2 mL, and 3 mL of aqueous ammonia solution were dissolved in 50 mL of water. After the surfactant was fully dissolved, 1.8 g of VTES was added dropwise into the solution with vigorous stirring at room temperature (25 °C). The molar ratio of the reactants was VTES/CTAB/NH3/H2O = 1
:
(0.0045–0.057)
:
(5.6–17)
:
287. The resultant mixture was stirred at the same temperature for additional 3 h, the solid product was recovered by filtration and air-dried at room temperature overnight. Finally, in order to completely remove the surfactant, 1.0 g of as-synthesized material was extracted by refluxing in a certain amount of ethanol containing concentrated aqueous HCl solution for 24 h. The extracted samples were vinyl-functionalized hollow mesoporous silica nanoparticles.
Synthesis of V-HMSNSs in alcohol–water solution using ammonia as the catalyst.
The synthesis procedure is similar to V-HMSNSs in aqueous solution, except that the alcohol water mixture was used as a solvent. The molar ratio of the reactants was VTES/CTAB/NH3/EtOH/H2O = 1
:
0.057
:
5.6
:
(0–90)
:
(294–0).
Characterization methods
Transmission electron microscopy (TEM) (JEM-2100F STEM/EDS) was used for observing the morphology and measuring the geometric parameters of the particles. In addition, the average diameters of the samples were defined by statistics
, where xi is the diameter gained by measuring n spheres (n ≥ 5) for each sample using TEM, and
is the average particle size. The samples were prepared by dipping a carbon coated copper grid, and evaporating the ethanol solvent. Fourier transform infrared (FTIR) spectra were collected using a Nicolet Nexus 470 IR spectrometer with KBr pellets. Thermogravimetric analysis (TGA) was carried out on a TG 209 instrument to determine the thermal stability of the samples, and the heating rate was 25 °C min−1 from 25 to 1000 °C under room atmospheric pressure. X-ray photoelectron spectroscopy (XPS) (VG Multilab 2000) was used to provide information on organic group coverage on the surface. The nitrogen adsorption experiment was performed at 77 K on a Micromeritics ASAP 2020 system. Prior to the measurements, the samples were outgassed at 150 °C for 7 h. The Brunauer–Emmett–Teller (BET) specific surface areas were calculated using adsorption data. The total pore volumes were estimated from the amounts adsorbed at highest relative pressure (P/P0) for each sample (P/P0 = 0.99). Pore size distributions were calculated from the adsorption branch using the Barrett–Joyner–Halenda (BJH) method.
Results and discussion
The formation mechanism of V-HMSNSs
A fundamental condition for the synthesis of V-HMSNSs was that an attractive interaction between the template and the silica precursor was produced to ensure inclusion of the structure director without phase separation taking place. According to the suggestion of Huo et al.,30,31 if the reaction takes place under basic conditions (whereby the silica species were present as anions) and cationic quaternary ammonium surfactants were used as the structure-directing agents (SDAs), the synthetic pathway was termed S+I− (S: surfactant; I: inorganic species). In this study, V-HMSNSs were obtained using this synthetic strategy, the typical synthesis of V-HMSNSs was schematically illustrated in Scheme 1. A vinyl functionalized silica shell was directly formed on the surface of each CTAB micelle by the hydrolysis and condensation of VTES with the aid of an ammonium catalyst, forming a core–shell structure, denoted CTAB@V-SiO2. Finally, cationic surfactants, CTAB cores, were extracted completely to synthesize V-HMSNSs by using a certain amount of ethanol containing concentrated aqueous HCl solution.
 |
| Scheme 1 Schematic illustration of one step method based on the soft template route for preparing V-HMSNSs. | |
Effect of the concentrations of CTAB
It has been well known that CTAB not only acted as a template, but also acted as a surfactant. The vinyltriethoxysilane precursor can form an emulsion with the aid of a surfactant, which favored the hydrolysis and condensation of the precursor in aqueous solution. Therefore, the influence of different concentrations of CTAB on the formation of V-HMSNSs has been investigated in aqueous solution using ammonia as the catalyst. As listed in Table 1, it was found that no particles were produced until the concentration of CTAB was reduced to 0.005 in aqueous solution. Fig. 1 shows the TEM images of samples synthesized at different concentrations of CTAB: (A, B) = 0.0005 M, (C, D) = 0.0009 M and (E, F) = 0.005 M in aqueous solution, in which Fig. 1(A), (C) and (E) referred to the low magnification TEM images, and Fig. 1(B), (D) and (F) referred to the corresponding high magnification TEM images. It was clearly observed that the solid nanospheres were changed into hollow mesostructures as the concentration of CTAB was increased from 0.0005 to 0.0009 M. And more interestingly, the coexistence of nanospheres and nanorods with hollow mesostructures occurred when the concentration of CTAB was up to 0.0009 M (Fig. 1(C) and (D)). While the CTAB concentration was further increased to 0.005 M, the completely vinyl functionalized hollow mesoporous silica nanorods can be obtained (Fig. 1(E) and (F)). Based on the previous reports, it was well known that the critical micelle concentration (CMC) of CTAB in aqueous solution was about 0.0009 M.32,33 So it was not difficult to understand that single micelles were formed when the concentration of CTAB was lower than 0.0009 M, resulting in hydrolysis and condensation of VTES alone forming solid spheres, as shown in Fig. 1(A) and (B). While the concentration of CTAB was increased to its CMC value, the single micelles may be aggregated into large rod-like micelles, which favored the inclusion of CTAB by VTES molecules, finally leading to the formation of hollow mesostructures, just as indicated in Fig. 1(C) and (D). And with the CTAB concentration further increased, larger aggregates of micelles were formed, resulting in the formation of rod-like micelles, and then VTES molecules were hydrolyzed and condensed around the surface of rod-like micelles. Consequently, rod-like hollow mesostructures were completely generated, as shown in Fig. 1(E) and (F). Furthermore, in order to characterize the structure and the component of V-HMSNSs, the FTIR spectra of (a) CTAB, (b) CATB@V-SiO2, (c) V-HMSNSs and (d) pure silica nanospheres were compared, as shown in Fig. 2. For CATB@V-SiO2 (Fig. 2(b)), bands observed at 2921 cm−1 and 2839 cm−1 were attributed to CTAB, which were assigned to asymmetric (2921 cm−1) and symmetric (2839 cm−1) stretching vibrations of C–CH2 in the methylene chains (Fig. 2(a)). However, for V-HMSNSs (Fig. 2(c)), no absorption peaks were observed at this range. In addition, the characteristic peaks of V-HMSNSs can be clearly found as compared with the typical spectrum of standard pure silica nanospheres, such that the peaks at 3064, 3019 and 2965 cm−1 were assigned to stretching vibrations of
CHx in the V-HMSNS frameworks. Also the strong amplitudes of Si–O–Si at 1096 cm−1 in pure silica networks (Fig. 2(d)) were split into two peaks (1046 and 1134 cm−1) in V-HMSNSs.34 The results of FTIR spectra fully illustrated that CTAB was completely removed from V-HMSNSs through extraction, besides, vinyl groups existed in the structure of V-HMSNSs. In addition, in order to indicate the distribution and the percentage of the organic groups in samples, Fig. 3(b) presented the TGA curve of V-HMSNSs synthesized in this study. In comparison, the TGA curve of pure silica nanoparticles was also given, as shown in Fig. 3(a). There was no obvious weight loss in the entire TGA process, except that weight loss in the range of 30–160 °C due to evaporation of surface physical water. However, for the V-HMSNSs, the TGA process involves four main stages (Fig. 3(b)). In the first stage (30−180 °C), the weight remained almost unchanged, which indicated that the V-HMSNSs have no obvious physical/chemical change. In the second stage (180–250 °C), the weight increased by 7.3%, which revealed the oxidation of vinyl groups.35 In the third stage (250–450 °C), the weight decreased sharply with the increment of temperature, indicating that the oxides may be further changed into CO2 resulting in weight loss. In the fourth stage (450–800 °C), the weight declined slowly. While the temperature was above 800 °C, the weight remained nearly unchanged. Meanwhile, the surface chemical composition of the V-HMSNSs was determined by XPS. Fig. 4(b) shows a typical XPS survey spectrum of the pure silica surface. The characteristic signals for pure silica (Si2p at 103 eV and Si2s at 155 eV) and oxygen (O1s at 531.8 eV) are clearly detected. An additional carbon signal (C1s at 285 eV) is also detected, due to the interference from unavoidable contamination of pure silica during analysis.36,37 Comparatively, for V-HMSNSs, characteristic signals attributed to silicon, oxygen and additional signals assigned to C1s at 285 eV, and the carbon signal is noticeably stronger compared with the pure silica surface, as shown in Fig. 4(a), due to the additional carbon in the V-HMSNS frameworks. Besides, the ratio of C1s/O1s of V-HMSNSs, compared with the pure silica nanoparticles, was increased from 3/61 to 42/22, which suggests that a large number of hydroxyl groups in pure silica are replaced by vinyl groups in V-HMSNSs.
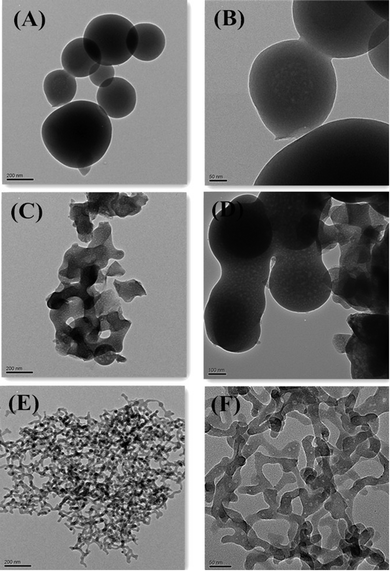 |
| Fig. 1 TEM images of V-HMSNSs synthesized with different concentrations of CTAB: (A, B) = 0.0005 M, (C, D) = 0.0009 M and (E, F) = 0.005 M in aqueous solution. | |
Table 1 Physicochemical parameters of the samples synthesized using different concentrations of CTAB at room temperature (25 °C)
No. |
Water (mL) |
CTAB concentrations (M) |
Average particle size (nm) |
BET surface area (m2 g−1) |
Total pore volume (cm3 g−1) |
1 |
50 |
0.01 |
No particles |
— |
— |
2 |
50 |
0.008 |
No particles |
— |
— |
3 |
50 |
0.005 |
2070 |
113 |
0.24 |
4 |
50 |
0.0009 |
512 |
14 |
0.019 |
5 |
50 |
0.0005 |
510 |
Solid spheres |
Solid spheres |
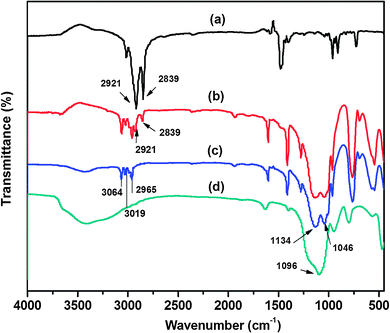 |
| Fig. 2 FTIR spectra of (a) CTAB, (b) CATB@V-SiO2, (c) V-HMSNSs and (d) pure silica nanospheres. | |
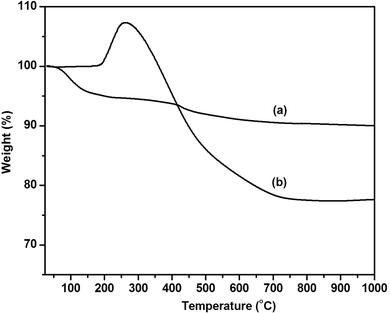 |
| Fig. 3 TGA analysis of (a) pure silica nanoparticles and (b) V-HMSNSs. | |
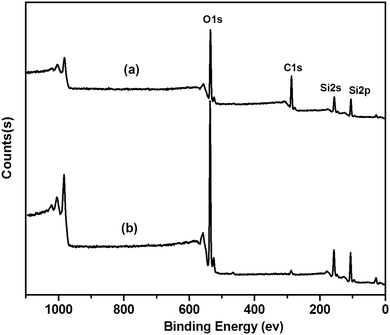 |
| Fig. 4 XPS analysis of (a) V-HMSNSs and (b) pure silica nanoparticles. | |
To better understand the effect of CTAB concentration on the formation of a mesostructure, N2 adsorption–desorption measurements were carried out on the products synthesized in aqueous solution using different concentrations of CTAB. Fig. 5 shows the representative N2 adsorption–desorption isotherms and the corresponding pore size distributions of the samples whose TEM images were displayed in Fig. 1(C)–(F). From N2 adsorption–desorption isotherms, it was found that all of these two samples exhibited a typical isotherm, these behaviors illustrated that the shells on the surface of the obtained V-HMSNSs were porous as well as showed a very broad distribution of these pores. The pore size distributions of the obtained V-HMSNSs were calculated by the BJH method from the adsorption branch. On the right side of Fig. 5 gave the size distributions of obtained V-HMSNSs with different concentrations of CTAB (0.0009 M and 0.005). It can be clearly observed that the pore size distributions of these two samples were all very broad in the range of 1 nm to 65 nm.
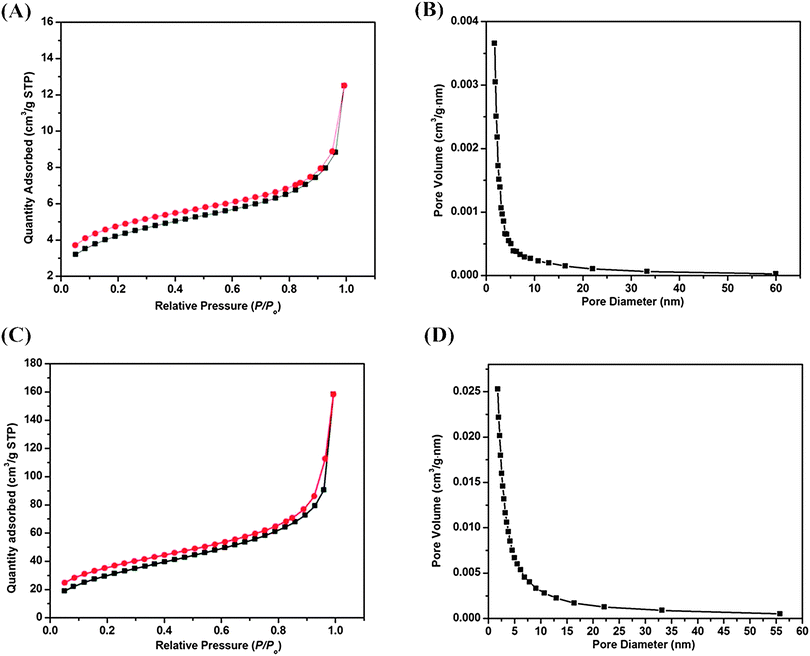 |
| Fig. 5 Nitrogen adsorption (■) and desorption ( ) isotherm curves and corresponding pore size distributions of V-HMSNSs synthesized with different concentrations of CTAB: (A, B) = 0.0009 M and (C, D) = 0.005 M in aqueous solution. | |
In addition, the BET surface area and total volume of obtained V-HMSNSs are all listed in Table 1. It was found that the BET surface area and total volume of obtained V-HMSNSs were increased as the CTAB concentrations were increased, which should be a result of the different porous structures of V-HMSNSs prepared with different CTAB concentrations. For one thing, the rate of hydrolysis and condensation of VTES can be accelerated with the increase of the concentration of CTAB. For another, the increase of the concentration of CTAB was helpful to form more micelles, and further wrapped by silica spheres, which was in favor of the formation of more pores.
Effect of the amounts of ammonia
Generally hydrolysis of organosilanes was a very slow reaction, though acids or bases are used as catalysts. Therefore, it was believed that the hydrolysis and condensation rates of the organosilanes were closely related to the amounts of acids or bases or catalysts. In this study, the influence of different amounts of ammonia catalyst in aqueous solution on the formation of V-HMSNSs has been discussed. Fig. 6 shows the representative TEM images of the samples prepared at different volumes of ammonia: (A) 1 mL, (B) 2 mL and (C) 3 mL in aqueous solution at CMC values of CTAB. Obviously, hollow-to-solid sphere transition occurred as the amount of ammonia was increased from 1 mL to 3 mL (Fig. 6(A)). It was not difficult to explain that the hydrolysis and condensation rates of the vinyltriethoxysilane precursor in aqueous solution may be greatly accelerated at higher amounts of ammonia, resulting in the formation of a relatively large solid sphere. For instance, CTAB@V-SiO2 nanocomposite particles with thick shell were formed as the amount of ammonia was increased to 2 mL, as shown in Fig. 6(B). Furthermore, larger solid spheres were obtained as the amount of ammonia was increased up to 3 mL (Fig. 6(C)).
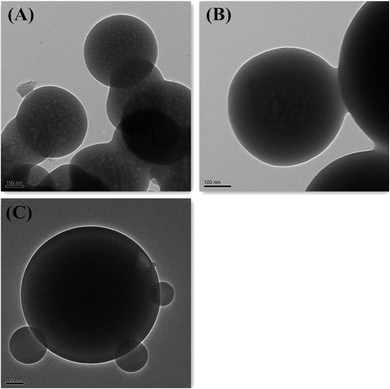 |
| Fig. 6 TEM images of V-HMSNSs synthesized with different volumes of NH3: (A) = 1 mL, (B) = 2 mL and (C) = 3 mL in aqueous solution. | |
Effect of the volume ratios of alcohol to water
It was well known that ethanol played a very important role in determining the hydrolysis and condensation rates of silanes. According to the previous reports, ethanol can greatly promote the hydrolysis and condensation rates of a silane precursor, and this was closely related to the amount of alcohol used.38 Therefore, in order to further study the influence of ethanol on the formation of V-HMSNSs, the effect of the volume ratio of alcohol to water on the formation of V-HMSNSs has been investigated with other conditions fixed, as listed in Table 2. It was found that no particles were formed as the volume ratio of alcohol to water was increased from 0
:
50 to 15
:
35 (Table 2). While the volume ratio of alcohol to water was increased to 20
:
30, the TEM image confirmed that the sample was composed of irregular spherical solid particles with a large average size of around 1660 nm (Fig. 7(A)), and agglomerates of silica nanoparticles (Fig. 7(B)). As the volume ratio of alcohol to water was further increased to 25
:
25, the part of ellipsoidal silica particles with mesostructure was observed in the TEM image in Fig. 7(C). However, there were still some spherical solid particles in the sample (Fig. 7(D)). Fig. 8 shows the representative N2 adsorption–desorption isotherms and the corresponding pore size distribution of the samples whose TEM images were displayed in Fig. 7(C) and (D). The BET surface area and total pore volume were relatively small of about 1.8 m2 g−1 and 0.0028 cm3 g−1, respectively (Table 2), due to the presence of some solid silica spheres. Meanwhile, the corresponding pore size distribution centered at 16.3 nm, and in addition to the mesoporosity, BJH pore size distribution clearly showed that V-HMSNSs also had micropores with a diameter centered at around 1.8 nm (Fig. 8(B)). The mechanism of hydrolysis and condensation of the vinyltriethoxysilane precursor is as follows:
Table 2 Physicochemical parameters of the samples synthesized using different alcohol–water ratios at room temperature (25 °C)
No. |
CTAB concentration (M) |
Alcohol/water volume ratio (v : v) |
Average particle size (nm) |
BET specific surface area (m2 g−1) |
Total pore volume (cm3 g−1) |
Pore size (nm) |
1 |
0.01 |
0 : 50 |
No particles |
— |
— |
— |
2 |
0.01 |
15 : 35 |
No particles |
— |
— |
— |
3 |
0.01 |
20 : 30 |
1660 |
Solid spheres |
Solid spheres |
Solid spheres |
4 |
0.01 |
25 : 25 |
3476 |
1.8 |
0.0028 |
6.1 |
5 |
0.01 |
40 : 10 |
No particles |
— |
— |
— |
6 |
0.01 |
50 : 0 |
No particles |
— |
— |
— |
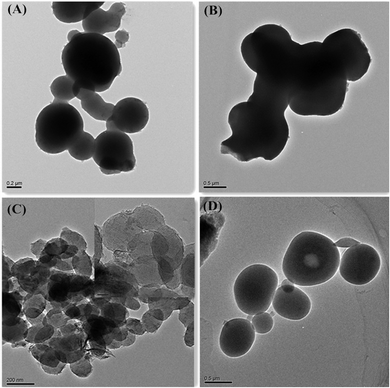 |
| Fig. 7 TEM images of V-HMSNSs synthesized with different volume ratios of alcohol to water: (A, B) 20 : 30 and (C, D) = 25 : 25. | |
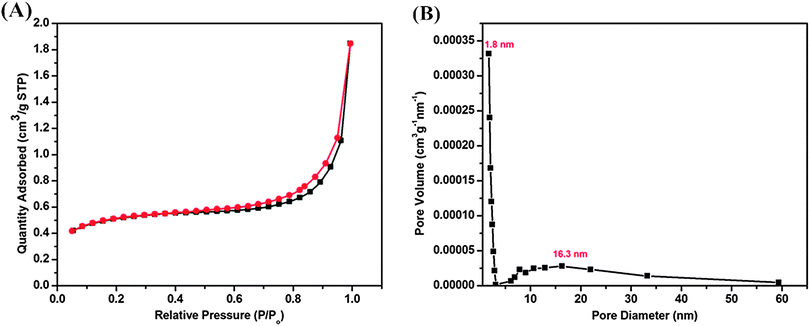 |
| Fig. 8 (A) Nitrogen adsorption (■) and desorption ( ) isotherm curve and (B) corresponding pore size distribution of V-HMSNSs synthesized with a volume ratio of alcohol to water of 25 : 25. | |
Hydrolysis:
|  | (1) |
Condensation:
| 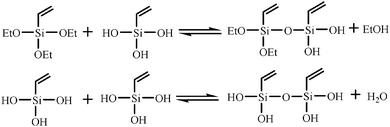 | (2) |
|  | (3) |
This suggested that the formation of vinyl functionalized silica nanospheres under the current conditions was mainly dependent on the hydrolysis and condensation rates of vinyltriethoxysilane. Furthermore, the hydrolysis and condensation rates of vinyltriethoxysilane will be affected by the amount of ethanol used. It was believed that the hydrolysis and condensation rates of the vinyltriethoxysilane precursor may be greatly suppressed under pure water conditions or at a low ratio of alcohol to water. In this study, the results proved that the particles were not obtained until the volume ratio of alcohol to water was up to 20
:
30. At this point, a few hydrolyzed silanol groups were further condensed into a small silica oligomer before compacting with the CTAB template, solid spheres were thus obtained. While the volume ratio of alcohol to water was further increased to 25
:
25, the adequate amount of ethanol favored the formation of a large number of hydrolyzed silanol groups, and these hydrolyzed silanol groups may be collided with the CTAB molecules, and further hydrolysis and condensation take place on the surface of the CTAB template before self condensation occurred. Consequently, V-HMSNSs were synthesized after removal of the CTAB template. However, the hydrolysis rate of the vinyltriethoxysilane precursor was much faster than the condensation rate when the volume ratio of alcohol to water was beyond 25
:
25, such as 40
:
10 or 50
:
0, only hydrolyzed silanol groups or silica oligomers were formed. So no particles were observed (Table 2).
Conclusions
A facile and low cost method has been developed for the synthesis of vinyl functionalized hollow mesoporous silica nanospheres under mild conditions. The vinyl functionalized hollow mesostructure silica nanospheres and nanorods can be obtained by adjusting the cetyltrimethylammonium bromide concentration in aqueous solution at low ammonia concentrations. Vinyl functionalized hollow mesostructure silica nanospheres and nanorods were formed at the critical micelle concentration value of the cetyltrimethylammonium bromide or little higher this value. Meanwhile, the hollow mesostructure nanospheres can also be prepared under similar conditions except for the addition of alcohol, and the results showed that the vinyl functionalized hollow mesostructure silica nanospheres can be obtained only when the volume ratio of alcohol to water was 25
:
25. In addition, vinyl functionalized hollow mesoporous silica nanospheres are expected to be used in adsorption as well as microencapsulation.
Acknowledgements
This work was partially supported by the National Natural Science Foundation of China (Grants 51273155). This work was also financially supported by the GuangDong Well-SilicaSol Co., Ltd, China.
References
- H. Djojoputro, X. F. Zhou, S. Z. Qiao, L. Z. Wang, C. Z. Yu and G. Q. Lu, J. Am. Chem. Soc., 2006, 128, 6320 CrossRef CAS PubMed.
- F. Hoffmann, M. Cornelius, J. Morell and M. FrÖba, Angew. Chem., Int. Ed., 2006, 45, 3216 CrossRef CAS PubMed.
- J. Y. Yuan, Y. Y. Xu, A. Walther, S. Bolisetty, M. Schumacher, H. Schmalz, M. Ballauff and A. H. Müller, Nat. Mater., 2008, 7, 718 CrossRef CAS PubMed.
- J. Liu, S. Y. Bai, H. Zhong, C. Li and Q. H. Yang, J. Phys. Chem. C, 2010, 114, 953 CAS.
- A. E. Kadib, N. Katir, M. Bousminaac and J. P. Majoral, New J. Chem., 2012, 36, 241 RSC.
- M. Mandal and M. Kruk, Chem. Mater., 2012, 24, 123 CrossRef CAS.
- X. B. Li, Y. Yang and Q. H. Yang, J. Mater. Chem. A, 2013, 1, 1525 CAS.
- J. S. Beck, J. C. Vartuli, W. J. Roth, M. E. Leonowicz, C. T. Kresge, K. D. Schmitt, C. T. W. Chu, D. H. Olson, E. W. Sheppard, S. B. McCullen, J. B. Higgins and J. L. Schlenker, J. Am. Chem. Soc., 1992, 114, 10834 CrossRef CAS.
- C. T. Kresge, M. E. Leonowicz, W. J. Roth, J. C. Vartuli and J. S. Beck, Nature, 1992, 359, 710 CrossRef CAS.
- S. Inagaki, S. Guan, Y. Fukushima, T. Ohsuna and O. Terasaki, J. Am. Chem. Soc., 1999, 121, 9611 CrossRef CAS.
- B. J. Melde, B. T. Holland, C. F. Blanford and A. Stein, Chem. Mater., 1999, 11, 3302 CrossRef CAS.
- T. Asefa, M. J. MacLachlan, N. Coombs and G. A. Ozin, Nature, 1999, 402, 867 CAS.
- A. M. Liu, K. Hidajat, S. Kawi and D. Y. Zhao, Chem. Commun., 2000, 1145 RSC.
- A. Matsumoto, K. Tsutsumi, K. Schumacher and K. K. Unger, Langmuir, 2002, 18, 4014 CrossRef CAS.
- D. R. Radu, C.-Y. Lai, J. W. Wiench, M. Pruski and V. S.-Y. Lin, J. Am. Chem. Soc., 2004, 126, 1640 CrossRef CAS PubMed.
- S. L. Burkett, S. D. Sims and S. Mann, Chem. Commun., 1996, 1367 RSC.
- M. H. Lim, C. F. Blanford and A. Stein, J. Am. Chem. Soc., 1997, 119, 4090 CrossRef CAS.
- R. Anwander, I. Nagl and M. Widenmeyer, J. Phys. Chem. B, 2000, 104, 3532 CrossRef CAS.
- K. Yao, Y. S. Imai, L. Y. Shi, A. M. Dong, Y. Adachi, K. Nishikubo, E. Abe and H. Tateyama, J. Colloid Interface Sci., 2005, 285, 259 CrossRef CAS PubMed.
- Q. Wei, Z. R. Nie, Y. L. Hao, Z. X. Chen, J. X. Zou and W. Wang, Mater. Lett., 2005, 59, 3611 CrossRef CAS PubMed.
- M. Choi, F. Kleitz, D. Liu, H. Y. Lee, W. S. Ahn and R. Ryoo, J. Am. Chem. Soc., 2005, 127, 1924 CrossRef CAS PubMed.
- Y. S. Lin, S. H. Wu, C. T. Tseng, Y. Hung, C. Chang and C. Y. Mou, Chem. Commun., 2009, 3542 RSC.
- P. Zarabadi-Poor, A. Badiei, B. D. Fahlman, P. Arab and G. M. Ziarani, Ind. Eng. Chem. Res., 2011, 50, 10036 CrossRef CAS.
- L. Han, Q. R. Chen, Y. Wang, C. B. Gao and S. A. Che, Microporous Mesoporous Mater., 2011, 139, 94 CrossRef CAS PubMed.
- R. Vathyam, E. Wondimu, S. Das, C. Zhang, S. Hayes, Z. M. Tao and T. Asefa, J. Phys. Chem. C, 2011, 115, 13135 CAS.
- B. Liu, E. W. Yan, X. Zhang, X. L. Yang and F. Bai, J. Colloid Interface Sci., 2012, 369, 144 CrossRef CAS PubMed.
- H. Yoshitake, New J. Chem., 2005, 29, 1107 RSC.
- I. A. Rahman, M. Jafarzadeh and C. S. Sipaut, Ceram. Int., 2009, 35, 1883 CrossRef CAS PubMed.
- Y. Naka, Y. Komori and H. Yoshitake, Colloids Surf., A, 2010, 361, 162 CrossRef CAS PubMed.
- Q. S. Huo, D. I. Margolese, U. Ciesla, D. G. Demuth, P. Y. Feng, T. E. Gier, P. Sieger, S. A. Firouzi, B. F. Chmelka, F. SchÜth and G. D. Stucky, Chem. Mater., 1994, 6, 1176 CrossRef CAS.
- Q. S. Huo, D. I. Margolese, U. Ciesla, P. Y. Feng, T. E. Gier, P. Sieger, R. Leon, P. M. Petroff, F. SchÜth and G. D. Stucky, Nature, 1994, 368, 317 CrossRef CAS.
- W. Li, Y. C. Han, J. L. Zhang, L. X. Wang and J. Song, Colloid J., 2006, 68, 304 CrossRef CAS.
- M. Bielawska, A. Chodzińska, B. Jańczuk and A. Zdziennicka, Colloids Surf., A, 2013, 424, 81 CrossRef CAS PubMed.
- H. T. Pu, X. Zhang, J. J. Yuan and Z. L. Yang, J. Colloid Interface Sci., 2009, 331, 389 CrossRef CAS PubMed.
- Z. Meng, C. Y. Xue, Q. H. Zhang, X. Yu, K. Xi and X. Jia, Langmuir, 2009, 25, 7879 CrossRef CAS PubMed.
- Z. Q. Wu, H. Chen, X. L. Liu, Y. Zhang, D. Li and H. Huang, Langmuir, 2009, 25, 2900 CrossRef CAS PubMed.
- I. O. Ucar, M. D. Doganci, C. E. Cansoy, H. Y. Erbil, I. Avramova and S. Suzer, Appl. Surf. Sci., 2011, 257, 9587 CrossRef CAS PubMed.
- W. Stöber and A. Fink, J. Colloid Interface Sci., 1968, 26, 62 CrossRef.
|
This journal is © The Royal Society of Chemistry and the Centre National de la Recherche Scientifique 2015 |
Click here to see how this site uses Cookies. View our privacy policy here.