DOI:
10.1039/C5LC00891C
(Paper)
Lab Chip, 2016,
16, 132-141
Self-powered switch-controlled nucleic acid extraction system†
Received
29th July 2015
, Accepted 21st October 2015
First published on 27th October 2015
Abstract
Over the past few decades, lab-on-a-chip (LOC) technologies have played a great role in revolutionizing the way in vitro medical diagnostics are conducted and transforming bulky and expensive laboratory instruments and labour-intensive tests into easy to use, cost-effective miniaturized systems with faster analysis time, which can be used for near-patient or point-of-care (POC) tests. Fluidic pumps and valves are among the key components for LOC systems; however, they often require on-line electrical power or batteries and make the whole system bulky and complex, therefore limiting its application to POC testing especially in low-resource setting. This is particularly problematic for molecular diagnostics where multi-step sample processing (e.g. lysing, washing, elution) is necessary. In this work, we have developed a self-powered switch-controlled nucleic acid extraction system (SSNES). The main components of SSNES are a powerless vacuum actuator using two disposable syringes and a switchgear made of PMMA blocks and an O-ring. In the vacuum actuator, an opened syringe and a blocked syringe are bound together and act as a working syringe and an actuating syringe, respectively. The negative pressure in the opened syringe is generated by a restoring force of the compressed air inside the blocked syringe and utilized as the vacuum source. The Venus symbol shape of the switchgear provides multiple functions including being a reagent reservoir, a push-button for the vacuum actuator, and an on–off valve. The SSNES consists of three sets of vacuum actuators, switchgears and microfluidic components. The entire system can be easily fabricated and is fully disposable. We have successfully demonstrated DNA extraction from a urine sample using a dimethyl adipimidate (DMA)-based extraction method and the performance of the DNA extraction has been confirmed by genetic (HRAS) analysis of DNA biomarkers from the extracted DNAs using the SSNES. Therefore, the SSNES can be widely used as a powerless and disposable system for DNA extraction and the syringe-based vacuum actuator would be easily utilized for diverse applications with various microchannels as a powerless fluidic pump.
Introduction
With advances in medical technology, various Point-Of-Care (POC) testings, which enable medical diagnosis to be performed near the patients, are becoming more widely available.1–3 A POC diagnostic system provides the patients' health status in a relatively faster, cheaper and easier way than existing methods. Especially, POC technology enables effective prevention and early detection of diseases.4,5 Therefore, many research groups have devoted enormous efforts to developing POC diagnostic systems for various diseases. In the first stage of POC diagnostic system development, some requirements such as (i) Affordable, (ii) Sensitive, (iii) Specific, (iv) User-friendly, (v) Rapid and robust, (vi) Equipment-free and (vii) Deliverable to end-users (ASSURED) should be considered.6,7
Microfluidic lab-on-a-chip (LOC) systems are the most suitable platform for the satisfaction of the ASSURED requirements. Basically, bio-analytical devices integrated with microfluidic components can be used for multi-step processes, which used to be separately performed on a large scale, in a miniaturized system with fast analysis time, high sensitivity and specificity.8,9 Especially, polymeric material-based microfluidic components have many advantages including low-cost fabrication, duplicability and disposability. For a few decades now, the classical POC diagnostic device, “dipstick”, has been actively used for tests for pregnancy, influenza, cardiac markers and other infectious diseases.10–12 These inventions successfully provide a cost-effective and easy-to-use method. This type of POC diagnostic system, however, has limitations when being used for multi-step and complex fluid handling required for molecular diagnostics.12 The workflow for molecular diagnostics is typically divided into few steps including sample processing for nucleic acid extraction/purification, target amplification and signal read-out. Many LOC technologies, which can be operated in a simple manner without any additional equipment, have been developed for the target amplification and signal read-out steps.13–17 Unlike other steps, sample preparation steps require the implementation of several active components (pumps, valves and reactors) onto the microfluidic component due to its multi-step protocol (e.g. separation, lysis and purification). Fluidic pumps and valves are among the key active components of an LOC system; however, they often require on-line electrical power or batteries and make the whole system bulky and complex, therefore limiting its application to POC testing. A number of pump and valve components for microfluidic control have been actively studied for the development of equipment-free and user-friendly POC diagnostic systems. Microfluidic devices that utilize capillary force18–21 such as Microfluidic Paper-based Analytical Devices (μPADs)19,20 as well as gravity-driven pumps22,23 have been widely used as actuation sources to transport samples in POC diagnostic systems. Recently, for the self-powered microfluidic device, the method to pre-load vacuum inside the microfluidic device via a degassing process has been developed and used.24,25 Several research groups have developed hand-operated pumps as powerless pumps in POC devices.26–29 The pumps are operated with direct pressure by pushing elastic polymers implemented on the inlets of POC devices. A disposable syringe has been the popular choice as a power source.30–32 It can act as a vacuum source or a pressure source. Chin et al. recently developed a POC assay that utilized tubing pre-loaded with multi-step reagents and a syringe as the vacuum source.32 They successfully demonstrated that such a device can perform immunoassay for the diagnosis of infectious diseases in POC setting. While the pumps have advantages in the low-cost and simple process of fabrication, they have limitations for the reaction steps of the multi-step protocol which require precise flow rate control for sample processing in molecular diagnostics. More importantly, different flow rates can be required for different process steps in a device, which have not been produced by these pumping devices. Together with the development of powerless pumps, various types of powerless valves have been developed for microfluidic devices.33–38 Microvalves are important as active components in the bio-analytical process to control fluid flows by opening or closing fluidic passageways. The valve operation is strongly related to an actuation mechanism and its application. Therefore, it is necessary to develop a straightforward and simple powerless controllable pump with availability of sequential injection including a washing step and a suitable microvalve to establish an equipment-free POC diagnostic system.
In this paper, we report a self-powered switch-controlled nucleic acid extraction system (SSNES) for the sample preparation step of a POC diagnostic process. The restoring force of the compressed air inside a blocked syringe was used as the actuating energy to achieve an equipment-free vacuum actuator. In our system, the flow rate and volume of the pulled reagent for each unit can be separately controlled by selecting syringes with different sizes and the initial location of the plunger of the vacuum actuator. Before the prototype fabrication of the SSNES, the working principle of a powerless vacuum actuator was suggested and validated by numerical modelling and experimental testing. Then, the portable and disposable SSNES was realized by assembling three sets of the vacuum actuator, the switchgear and microfluidic channels. In the DNA extraction process, DMA, which was characterized in our previous work,39 was used as a non-chaotropic reagent to eliminate some required injection steps for the extraction process and derestrict material selection of DNA extraction devices. Finally, the performance of the SSNES was demonstrated by genetic analysis of extracted DNA using the HRAS gene and the result was investigated through gel electrophoresis.
Scheme of SSNES
The structure of the SSNES is largely separated into two components. Fig. 1 presents the schematic diagram of the structures and simplified operation procedures of the SSNES. The first component of the SSNES is a vacuum actuator made of two disposable syringes, which are indicated at the left side of the schematic diagram. The actuator consists of an opened syringe, a blocked syringe, a syringe binder, a stopper and a container with supporting slots for the stopper and flanges of the syringes. The opened syringe and the blocked syringe act as a working syringe and an actuating syringe in the system, respectively. The two syringes are held together by a syringe binder which joins the plunger ends and the flanges are fixed by slots on the side wall of the container to work as a part. Then, in order to generate potential energy, the syringe binder is pushed up and fixed at a supporting slot by a stopper. The vacuum actuator is operated by the generated negative pressure due to the restoring force of the compressed air inside the blocked syringe.
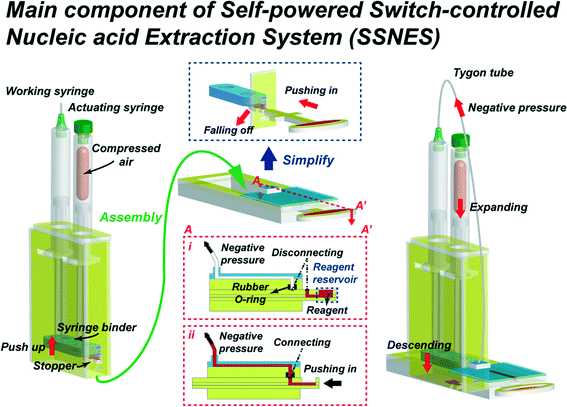 |
| Fig. 1 Schematic diagram of the structures and simplified operation procedures of the SSNES. (Left part) a powerless vacuum actuator made of two disposable syringes (working and actuating). The generation of potential energy via pushing up syringe ends held by a syringe binder and fixing at a supporting slot by a stopper. (Middle part) a multi-function switchgear component including a Venus symbol shape of the switchgear (reagent reservoir, push-button for the vacuum actuator and on–off valve function). The operation of the switchgear component by sliding the Venus symbol shape of the switchgear to connect (on) or disconnect (off) the outlet of the reservoir and the inlet of the main channel (middle insets i & ii). (Right part) a unit of SSNES assembled by inserting the powerless vacuum actuator into the switchgear component. The working process of the unit of SSNES following the sequence: removal of a stopper by pushing the switchgear, descent of two bound syringes due to the restoring force of the compressed air within the blocked syringe, generation of negative pressure in the opened syringe and pulling of the reagent fluid from the reservoir by flowing through the main channel into the opened syringe. | |
The second component is a Venus symbol shape of the switchgear where the ellipse part is used as the reagent reservoir and the cross part is used as the push-button for the vacuum actuator. The vacuum actuator is activated by pushing in the stopper to fall off from the supporting slot. When the switchgear is pushed in, the outlet of the reagent reservoir is aligned to the underneath of the liquid inlet of the main channel, which allows the reagent to flow into the main channel under negative pressure of the actuator. The detailed schematic diagram is shown in Fig. 1. For the on–off valve function, the switchgear is slid to connect (on) or disconnect (off) both coupling holes. The vacuum actuator and the switchgear are assembled together to make one unit of the SSNES as shown on the right side of Fig. 1. In a SSNES, when the switchgear part is pushed in to fall off a stopper, the plungers of two bound syringes descend due to the restoring force of the compressed air within the blocked syringe and consequently, negative pressure is generated in the opened syringe, which is connected to the outlet of the main channel via Tygon tubing. Finally, under the negative pressure, the reagent fluid is pulled from the reservoir and flows through the main channel into the opened syringe. A rubber O-ring is placed in between coupling holes of the reservoir and the main channel in order to prevent leakage of the reagent and keep the vacuum pressure within the entire unit.
Method
Modelling of powerless vacuum actuator
Prior to the experiments with the prototype, we first examined the effect of the volume of compressed air and compressibility on the velocity of descending plungers and the flow rate of the pulled reagent using a theoretical model. Fig. 2(a) presents the schematic diagram of a blocked syringe to demonstrate a plunger motion with the compressed air generated by pushing the plunger into the syringe. In order to derive the equation of the plunger motion, energy conservation under adiabatic conditions was considered as:where ΔW is the work generation by the expanded air, ΔKE is the kinetic energy change and ΔPE is the potential energy change. Due to the extremely lower value of ΔPE than ΔW, ΔPE can be ignored and ΔW (ΔW = maΔs) is directly converted to ΔKE (ΔKE = m(vl2 − vp2)/2) without ΔPE as:where vl is the later velocity of a plunger, vp is the previous velocity of a plunger, a is the acceleration of a plunger and Δs is the location change of a plunger. In addition, Δs is defined as:where sl is the later location of a plunger and sp is the previous location of a plunger. This simple eqn (2) is well-known as the Torricelli equation40 which is used to find the later velocity without having known time intervals.
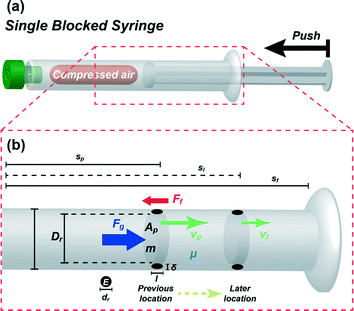 |
| Fig. 2 (a) Schematic diagram of single blocked syringe to demonstrate the plunger motion induced by the restoring force of the compressed air after pushing the plunger into the blocked syringe and (b) free-body diagram with parameters used in the modelling process to determine the forces applied to a plunger inside a cylinder of the syringe. | |
Fig. 2(b) displays a free-body diagram to determine the forces applied to a plunger inside a cylinder. As shown in Fig. 2(b), the net force applied to a compressed plunger is represented as follows:
|  | (4) |
where
where
where
Fg is the resultant force generated by a gas,
Ff is the friction force of a rubber-covered plunger with the inner wall,
m is the mass of a plunger,
Pg is the applied total pressure to a plunger,
Ap is the cross-section area of a plunger,
Pe is the expansion pressure of the compressed gas and
Patm is the atmospheric pressure.
Fg consists of
Pg and
Ap as defined in
eqn (5). In addition,
Pg is mainly influenced by
Pe and
Patm as shown in
eqn (6).
When compression or expansion takes place under adiabatic conditions inside a well-sealed cylinder, Pe varies with the volume of the compressed air by the polytropic process equation of an ideal gas, PV1.4 = Const′. In addition, the compressibility factor is the ratio between the volume of a gas and the volume of an ideal gas. The factor varies with the effect of temperature or pressure changes. Under our experimental conditions (pressure of 1 to 2 atm and isothermal at room temperature), the factor is almost 1 (0.9999), thus the compressibility of air can be negligible. The motion of a plunger induced by compressed air will be stopped at the final location of the plunger (sf) when Pe approaches Patm. For a well-sealed cylinder, sf is almost the same as the initial location before compressing the plunger. In this case, Pe along with sl is defined with known values of Patm and sf as:
| PeAp1.4sl1.4 = PatmAp1.4sf1.4 | (7) |
|  | (8) |
According to the above relationship,
Pg is redefined as
eqn (8) by substituting
Pe from
eqn (7) into
eqn (6).
As the working space is miniaturized, the contact force becomes more dominant for the motion of the plunger than the body force and is composed of complicated factors. Especially, the friction force of the rubber-sealed plunger in circular cylinders varies with the velocity and pressure of an object as follows:
|  | (9) |
where
μ is the dynamic viscosity of the flowed fluid in the friction region.
41 Constants
C and
D of the friction force are determined as:
|  | (10) |
where
B is the bore size of a cylinder,
d is the diameter of a rubber seal,
E is the Young's modulus of rubber,
ε is the elastic strain rate of a rubber seal,
Dr is the diameter of a plunger rod,
l is the contact length between a cylinder and a rubber-sealed plunger and
δ is the clearance gap between a cylinder and a plunger (
eqn (10) and
(11)).
Unlike Xia et al.'s study,41 in our system, the rubber friction of the plunger should be doubled since the actuating part (i.e. blocked syringe) and the working part (i.e. opened syringe) need to act in tandem in order to function as a vacuum actuator as described in the previous section. Therefore, from here on, the constants C and D will be replaced with 2C and 2D. By replacing Fg and Ff in eqn (4) with eqn (5) and (9), a is rewritten as eqn (13) and substituted into eqn (2) as:
|  | (12) |
where
|  | (13) |
After transposing and raising
eqn (12) to eliminate the square root, the quartic equation with respect to
vl is defined as:
|  | (14) |
Fig. 3(a) and (b) show the plots of the theoretical results for the flow rates of the pulled fluid (
Q =
vlAp)
versus the location of the compressed plunger (
sc =
sf −
sl) obtained using
eqn (14). In this work, we selected three commonly used BD disposable syringes (3, 5 and 10 mL) and other parameters were experimentally determined or obtained from the BD syringe specifications (see supplemental Table S1
† for values). We set the initial location of the plunger to 0 (the initial location means the location of the plunger when the syringe head is blocked in order to create an air chamber) and the starting location to −10 mm (the plunger is pushed in 10 mm from the initial location after the syringe head is blocked).
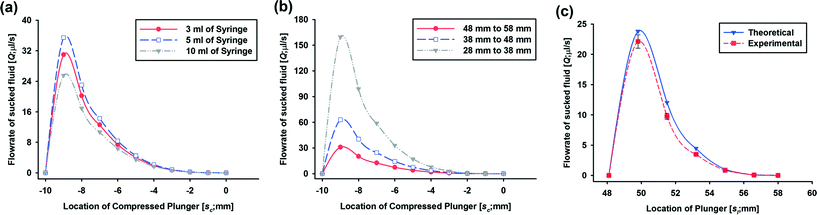 |
| Fig. 3 Resulting plots of the theoretical results for the flow rates of pulled fluid (Q = vlAp) according to the location of the compressed plunger (sc = sf − sl) from −10 mm (pushed plunger in 10 mm from the initial location after blocking the syringe head) to 0 (the blocked position of the syringe head to create an air chamber) using (a) different volumes of syringe sets (actuating & working) (3 ml (red), 5 ml (blue) and 10 ml (grey) syringes) and (b) different initial locations of plungers in a set of 3 ml BD syringes (58 mm (grey), 48 mm (blue) and 38 mm (red)) and (c) theoretical results for the flow rates of pulled fluid versus the (actual) locations of a plunger from 0.0481 m to 0.058 m with 0.0017 m increment (blue) using a set of 3 ml syringes and their comparison with experimental flow rates (red) under the same conditions. | |
The flow rates of the pulled fluid induced by the working syringe for the 3, 5 and 10 mL syringes are compared in Fig. 3(a). Even though the three sets of syringes have different dimensions, the flow rates of the different syringe sets are within a narrow range from 25 μl s−1 to 35 μl s−1 at the maximum speed. Therefore, we chose 3 mL syringes for the vacuum actuator from here on. Fig. 3(b) shows the flow rates of the pulled fluid depending on different initial locations of plungers in a set of 3 ml BD syringes. The flow rates decrease when the initial location of the plunger is farther from the syringe head (38 vs. 48 vs. 58 mm) since the ratio of the volume of compressed air to that of the air chamber in the blocked syringe decreases (0.26 vs. 0.21 vs. 0.17). These results show that the volume as well as the flow rate of the pulled liquid can be easily controlled by selecting syringes with different sizes and the initial location of the plunger of the powerless vacuum actuator. In general, a relatively low flow rate of the elution buffer is advantageous for breaking covalent bonds. When we connect the vacuum actuator to a microfluidic component with storage channels (SI, SII and SIII) for the elution of DNA, the flow rate of the elution buffer is reduced from 30 μl s−1 to below 3 μl s−1 due to fluidic resistance of the reagents. In the case of using DMA as a DNA capturing agent, we confirmed the elution performance by end-point PCR using different flow rates of the elution buffer ranging from 1 ml h−1 (0.28 μl s−1) to 3 ml h−1 (0.83 μl s−1). After flowing 50 μl of the elution buffer, the flow rate is continuously reduced and is within the range 0.28 μl s−1 to 0.83 μl s−1. In this project, we chose the initial plunger location of 58 mm since the flow rate is suitable for our DNA extraction system.
For the verification of the theoretical results, we experimentally measured the flow rate of the reagent using the powerless vacuum actuator made of a set of 3 ml syringes. The flow rate versus the (actual) location of the plunger (sl) derived from the theoretical calculation using eqn (14) and the experimental data are compared in Fig. 3(c). For the theoretical calculation, we set the value of location change, Δs, as 0.0017 m which is one increment of the 3 ml BD syringe. Finally, vl was calculated using eqn (14) and the flow rates were vl multiplied by the cross-section area of the syringe. For the experimental set-up, 3 ml of the blocked BD syringe was compressed by pushing a plunger and released. The plunger motion was recorded by means of a digital camera and the experiments were repeated three times. The captured images from the experimental video are presented in Fig. S2† to explain the detailed measurement and calculation processes. The velocity of the plunger was obtained using elapsed time for one increment movement from the recorded video and the flow rates of the pulled fluid were calculated using the velocity and the cross-section area of the cylinder. The theoretical and experimental data agree well with each other. In addition, the on–off valve function of the switchgear component was independently investigated using the PMMA prototype of the microchannel-attached switchgear component (Fig. S1†). Through functional testing, we confirmed the potential to use the switchgear as an on–off valve for a pump operated by negative pressure.
Design and fabrication
The prototype of the SSNES was constructed using disposable syringes, a PMMA sheet, double-sided tape and rubber O-rings. The SSNES consists of three sets of vacuum actuators, switchgears and microfluidic components. Fig. 4(c) presents the components and the detailed parts of each component are shown in Fig. 4(a), (b), (d) and (e). For a powerless vacuum actuator component, all parts were designed as a convex-groove structure and fabricated by cutting 2 mm thickness of a PMMA sheet using a CO2 laser cutting machine (Universal Laser Systems, AZ, USA) (Fig. 4(a)). The designs of the parts for the vacuum actuator component can be flexible depending on the dimensions of the syringes. The size of the container, however, should be large enough to prevent contact friction with the syringe binder part. In addition, the compressibility of the air inside an actuating syringe can be controlled with the fitted position of a stopper in support slots. Fabricated PMMA parts were assembled by fitting convex structures into groove structures without any adhesive. A switchgear component consists of a lower cover, an intermediate part, an upper cover with a coupling hole, switchgear assemblies, rubber O-rings, screws and nuts. Both covers and the intermediate part which includes the movement space for the switchgear assemblies were fabricated by cutting 2 mm thickness of a PMMA sheet (Fig. 4(b)). They were assembled by attaching 0.1 mm thickness of double-sided tape (3M, USA) on both sides of the intermediate part and fastening bolts with nuts. Before assembling, the switchgear assemblies were placed in the movement space of the intermediate part with rubber O-rings. The detailed composition of the switchgear assembly is a base layer, a coupling channel layer and a reagent reservoir layer as shown in Fig. 4(d). The dimensions of the reagent reservoir depend on the volume of a target reagent. The base, coupling channel and reagent reservoir layers were fabricated using 0.5 mm, 0.5 mm and 1 mm thicknesses of PMMA sheets, respectively, and assembled using 0.1 mm thickness of double-sided tape attached on both sides of the coupling channel layer. As mentioned above, the microfluidic component should include three independent inlets and outlets, a mixing channel and a binding channel for DNA extraction. Therefore, the microfluidic component was composed of five layers as shown in Fig. 4(e) and the detailed design and pathways of reagents are described in Fig. S3† (from the top, 1st layer – an APTES-treated slide glass, 2nd layer – 1 mm width and 0.5 mm thickness of a meandering channel as the binding channel, 3rd layer – 0.2 mm thickness of a coupling layer, 4th layer – 1 mm width and 0.5 mm thickness of a spiral channel as the mixing channel and 5th layer – 0.2 mm thickness of a coupling layer). In order to enhance the performance of DNA binding, the APTES-treated slide glass was fitted with an isolated binding channel. PMMA sheets sandwiched between two double-sided tapes were cut together along channel designs and the remaining sticky regions were covered by coupling layers. Finally, the SSNES was realized by inserting three powerless vacuum actuator components into a switchgear component and attaching a microfluidic component onto the front side of the switchgear component with a spacer.
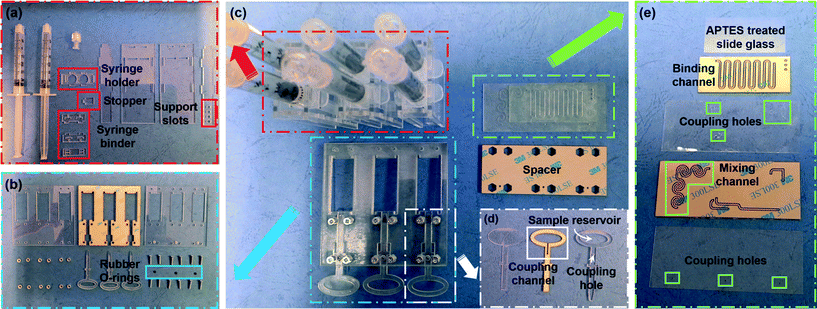 |
| Fig. 4 Breakdown of the illustrated parts of the SSNES (c) and its components: a powerless vacuum actuator component (a: an actuating syringe, a working syringe, a syringe holder, a stopper, a syringe binder assembly and four syringe container walls), a switchgear component (b: a lower cover, an intermediate part, an upper cover with coupling holes, Venus symbol shape of switchgear assemblies, rubber O-rings, screws and nuts), detailed parts of the switchgear assemblies (d: a base layer, a coupling channel layer and a reagent reservoir layer) and a microfluidic component (e: an APTES-treated slide glass, two coupling layers, a binding channel layer and a mixing channel layer). The powerless vacuum actuator is constructed by fitting convex-groove structures and the other components are assembled by attachment using double-sided tape. | |
Experimental
Materials
Distilled (DI) water and phosphate-buffered saline (1× PBS) were purchased from Invitrogen (Carlsbad, CA). 3-Aminopropyltriethoxysilane (APTES), bovine serum albumin (BSA), dimethyl adipimidate (DMA), sodium bicarbonate, agarose and ethidium bromide (EtBr) were purchased from Sigma-Aldrich (St. Louis, MO, USA). Lysis buffer (AL buffer and proteinase K), 10× PCR buffer, MgCl2, deoxynucleotide triphosphate and Taq DNA polymerase were purchased from Qiagen (Hilden, Germany). Other chemicals were of analytical reagent grade and were used as-received. All reagents and buffers were prepared using DI water and PBS.
DMA-based DNA extraction using a disposable microfluidic chip
In this work, we performed DMA-based DNA extraction in the microfluidic device. DMA is a non-chaotropic DNA capturing reagent, where its bi-functional imidoesters reversibly covalently bind with the amine groups on the sticky ends of the fragmented DNA.39 In the DMA-based DNA extraction process, the cell lysis and pre-modification steps can be carried out as a single step in a mixing channel. In addition, for a urine sample, a separation channel or a separation technique is not required to be integrated into a microfluidic component for the DNA extraction process since urine is relatively clean compared to blood with abundant red blood cells. For the mixing of reagents during the DNA extraction process, a spiral-shaped micromixer using the transverse Dean-vortex phenomenon is frequently used.39,42 Therefore, we designed spiral micromixers in a microfluidic component for the cell lysis and pre-modification steps. The detailed design of four sets of spiral micromixers is presented in Fig. S3.† For the purification of extracted DNAs, binding the DNA and washing the bound DNA are necessary. In this case, amine modification is frequently carried out on the surface of a binding channel to capture the DNA. In this work, 3-aminopropyltriethoxysilane (APTES) solution is used for the amine modification. In addition, covalent bonds of silane in APTES solution are easily formed with materials with a silanol group-containing surface such as glass or silicon. Thus, we chose a slide glass as the binding region and modified the surface using APTES. The process of amine modification with APTES was performed as follows: (i) the slide glass was treated with oxygen plasma to activate silanol groups using an NT-2 plasma cleaning system (Anatech Ltd., CA) under 100 W of treating power in an O2 flow rate of 80 sccm for 60 s, (ii) the activated glass was immersed in 2% APTES solution in 95% ethanol (v/v; 5% H2O with 95% ethanol) for 2 h, (iii) the APTES-treated glass was washed with ethanol and DI water for 5 min three times and dried under a nitrogen stream and (iv) the surface was cured at 120 °C for 15 min in a convection oven (Memmert, Frankfurt, Germany). In order to enhance the binding efficiency, we designed a wide meandering channel and covered the modified slide glass onto the isolated meandering channel.
Operating procedure
Fig. 5 shows the photographs of the assembled SSNES. When switchgears are pushed in, the stoppers, held in support slots, fall off by the prominent ends of the switchgears. As a result, a syringe binder descends due to compressed air pressure and reagents are pulled from the reservoir passing through a microchannel by negative pressure (Fig. 5(a)). Fig. 5(b) shows the finished prototype of the SSNES. RI, RII and RIII represents the first, second and third reservoirs for reaction agents, respectively. RI contains the mixture of lysis buffer (50 μl of AL buffer with 5 μl of proteinase K) with 100 μl of DMA for the cell lysis and DNA binding steps. In addition, 300 μl of PBS buffer is placed into RII to ready for washing and purification of the sample, and 150 μl of elution buffer (pH 10.6, 10 mM sodium bicarbonate) is placed into RIII to ready for the elution of extracted DNAs. Finally, RI, RII and RIII are covered with polycarbonate tape (PC tape) (3M, USA) to prevent the contamination of buffers and agents before operation. For the collection of extracted DNA, a thin plastic container is attached to the third working syringe. The container is divided into three sections (50 μl each) in order to investigate the amount of extracted DNA in different portions of elution buffer (front (SI), middle (SII) and end (SIII)) exiting from the microchannel.
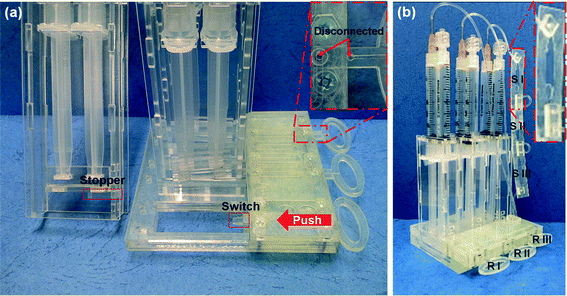 |
| Fig. 5 Photographs of the assembled SSNES with (a) the working principle of the SSNES following the sequence: fall of the stopper by pushing switchgears with prominent ends (switch), descent of the syringe binder induced by compressed air pressure and pulling of reagents from the reservoirs passing through a microchannel by negative pressure under connected conditions and (b) procedure instructions of DNA extraction with different roles of reservoirs and storages in the system. Three reservoirs contain (RI) the mixture of lysis buffer (50 μl of AL buffer with 5 μl of proteinase K) with 100 μl of DMA, (RII) 300 μl of PBS buffer and (RIII) 150 μl of an elution buffer. The DNA extraction process is initiated by placing 50 μl of urine into RI and the three solutions inside the reservoirs are sequentially injected. At the end of the process, 50 μl each of eluted DNA is separately collected in SI, SII and SIII as shown in the enlarged view (red-colored box). | |
The development of the SSNES aims it to be a user-friendly system which can be dealt by a non-professional user with simple instructions. Typically, urine can be easily self-collected by users at home and is therefore a suitable sample for point-of-care diagnosis. The operation procedure for the SSNES is as follows. Firstly, the user is to detach the PC tape from RI and add 50 μl of urine into RI using a disposable pipette and then push in RI. When the RI is pushed in, the powerless vacuum actuator I is initiated and starts pulling the reagent from RI. A mixture of lysis buffer, DMA and urine is injected into the first inlet of the microchannels and the solution is mixed in the spiral micromixers. The lysis reaction and simultaneous binding of DMA and extracted DNA occur during this step. While the mixture solution is passed through a binding channel, the DNA/DMA complexes are captured onto the APTES-treated slide glass. After passing through the binding channel, the rest of the mixture is flowed into a working syringe in the first vacuum actuator. At this point, the user should pull out RI to complete the first step. Then, the user is to detach the PC tape from RII and push in RII. In a similar way as the first step, 300 μl of PBS buffer is introduced into the second inlet of the microchannels to wash away non-specific and unbound molecules. Then, the waste is flowed into a working syringe in the second vacuum actuator. For the last step of the DNA extraction process, the user pushes RIII in after pulling RII out and detaching the PC tape from RIII. 150 μl of an injected elution buffer is flowed through a binding channel to elute DNA by breaking the covalent bonds between the amine groups (of DNA as well as APTES) and DMA. Each 50 μl of eluted DNA is separately collected in SI, SII and SIII as shown in the enlarged view (red-colored box) in Fig. 5(b). The entire process takes 10 min or less. In short, the user would operate the SSNES by simple steps of detaching tape, adding urine, and pushing and pulling switchgears. It provides quite an easy process and automatically guided flows to destinations (waste or storage) via separating actuation elements for the user-friendly sample preparation of POC diagnostics.
Results and discussions
Downstream analysis of extracted DNAs
In order to confirm the DNA extraction performance of the SSNES, downstream analysis of extracted DNAs was carried out with the HRAS gene, which is one of the DNA biomarkers for bladder cancer, using a PCR technique.43 The eluted DNA was amplified using primers for the HRAS gene (forward: 5′-TTTTCCCATCACTGGGTCAT-3′, reverse: 5′-GCCTCTCCCTGGTACCTCTC-3′) by conventional PCR and the results were confirmed through gel electrophoresis. The eluted DNA was collected from SI, SII and SIII as shown in Fig. 6(a). Firstly, conventional PCR was performed with a Taq PCR core kit. The reaction mix included 2.5 μl of 10× PCR buffer, 2 μl of 2.5 mM MgCl2, 2 μl of 0.25 mM deoxynucleotide triphosphate, 2 μl each of 25 pmol forward and reverse primers for the HRAS gene and 0.5 μl of Taq DNA polymerase (1 unit). The amplification of the master mix was performed with 35 cycles at 95 °C, 60 °C and 72 °C for 30 s, 30 s and 30 s, respectively. After 35 cycles, an elongation step was carried out at 72 °C for 7 min. Secondly, the PCR amplicons were visualized by gel electrophoresis. The gel was formed by curing 2 g ml−1 (2%) agarose pre-polymer with 1.5 μl of ethidium bromide (EtBr) for 60 min. Fig. 6(b) presents the electropherogram result of stained PCR amplicons according to different sized molecules with EtBr on an agarose matrix. M, SI, SII, SIII and N lanes in the result indicate the DNA ladder, amplified DNA samples from SI, SII and SIII, and no DNA template as a negative control, respectively. Band intensities directly indicate the quantity of HRAS genes in the PCR amplicons. From the dark bands of all extracted DNAs (SI, SII and SIII) unlike the negative control (N), we can confirm the existence of abundant HRAS genes in the extracted samples and the successful DNA extraction using the SSNES. In addition, extraction processes and visualizations were repeated three times and the results were confirmed to have similarities. Therefore, we expect that the SSNES can be widely used as a user-friendly operated and disposable powerless DNA extraction system and the syringe-based vacuum actuator would be easily utilized for diverse applications with various microchannels as a powerless fluidic pump.
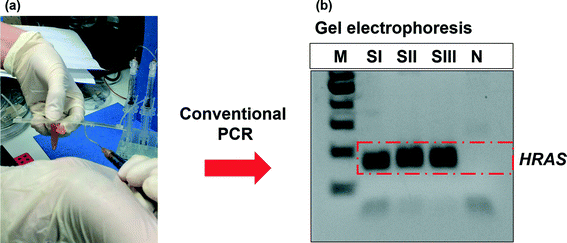 |
| Fig. 6 Genetic analysis of extracted DNAs with the HRAS gene (450 bp) by conventional PCR and gel electrophoresis. Images of (a) collection of eluted DNA from the first 50 μl of eluted DNA (SI), the second 50 μl of eluted DNA (SII), the third 50 μl of eluted DNA (SIII) and the master mix without DNA as a negative control (N), and (b) electropherogram results of stained PCR amplicons using SI, SII, SIII and N according to different sized molecules with EtBr on an agarose matrix. Extraction processes and visualizations were repeated three times and the results were confirmed to have similarities. | |
Conclusion
In this paper, we present the development of a self-powered switch-controlled nucleic acid extraction system (SSNES) that can extract DNA from body fluids in 10 min with simple operation steps. The entire system is mainly made of disposable syringes and plastic substrates and could be manufactured at low cost. The SSNES consists of a series of powerless vacuum actuator and switchgear units. The syringe-based vacuum actuator is used to generate negative pressure to pull samples and reagents through a microchannel. The flow rate and volume of the pulled reagent for each unit can be controlled by selecting syringes with different sizes and the initial location of the plunger of the vacuum actuator. The on–off valve function is provided by the Venus symbol shape of the switchgear which can also function as a reagent reservoir and a start-button for the vacuum actuator. The end-user can easily operate the SSNES through simple steps of detaching tape, and pushing and pulling switchgears for clinical sample processing. The actuating syringe can be pre-loaded to provide the required flow rate by the manufacturer of the SSNES. The pre-loaded vacuum actuator can be stored in a normal environment for over a month without losing the pressure. The maintenance performance of the pre-loaded vacuum actuator is evaluated by flow rate testing a month after loading the actuating syringe. Further optimization and storage condition studies are ongoing. Our device has great potential to be used for POC applications because it is disposable, self-powered, fast, inexpensive and simple to use. Furthermore, its simple structure makes it easy to integrate with other LOC systems for POC molecular diagnostics.
Acknowledgements
This work was supported by the Agency for Science, Technology and Research (A*STAR) Joint Council Office (JCO) Development Programme Grant (1234e00018), Singapore. The authors are also thankful for the financial support from a Ph.D. scholarship granted by the School of Mechanical and Aerospace Engineering at Nanyang Technological University and Nanyang Environment and Water Research Institute (NEWRI).
References
- R. W. Peeling and D. Mabey, Clin. Microbiol. Infect., 2010, 16, 1062–1069 CrossRef CAS PubMed.
- P. Yager, G. J. Domingo and J. Gerdes, Annu. Rev. Biomed. Eng., 2008, 10, 107–144 CrossRef CAS PubMed.
- C. P. Chan, W. C. Mak, K. Y. Cheung, K. K. Sin, C. M. Yu, T. H. Rainer and R. Renneberg, Annu. Rev. Anal. Chem., 2013, 6, 191–211 CrossRef CAS PubMed.
- R. Mukhopadhyay, Chem. World, 2010, 7, 50–53 CAS.
- D. C. H. Burgess, J. Wasserman and C. A. Dahl, Nature, 2006, 444, 1–2 CrossRef PubMed.
- A. W. Martinez, S. T. Phillips, G. M. Whitesides and E. Carrilho, Anal. Chem., 2010, 82, 3–10 CrossRef CAS PubMed.
- W. G. Lee, Y.-G. Kim, B. G. Chung, U. Demirci and A. Khademhosseini, Adv. Drug Delivery Rev., 2010, 62, 449–457 CrossRef CAS PubMed.
- S. K. Sia and G. M. Whitesides, Electrophoresis, 2003, 24, 3563–3576 CrossRef CAS PubMed.
- K. A. Heyries, M. G. Loughran, D. Hoffmann, A. Homsy, L. J. Blum and C. A. Marquette, Biosens. Bioelectron., 2008, 23, 1812–1818 CrossRef CAS PubMed.
- A. Chua, C. Y. Yean, M. Ravichandran, B. Lim and P. Lalitha, Biosens. Bioelectron., 2011, 26, 3825–3831 CrossRef CAS PubMed.
- B. Ngom, Y. Guo, X. Wang and D. Bi, Anal. Bioanal. Chem., 2010, 397, 1113–1135 CrossRef CAS PubMed.
- G. A. Posthuma-Trumpie, J. Korf and A. van Amerongen, Anal. Bioanal. Chem., 2009, 393, 569–582 CrossRef CAS PubMed.
- C. Liu, M. G. Mauk, R. Hart, X. Qiu and H. H. Bau, Lab Chip, 2011, 11, 2686–2692 RSC.
- B. A. Rohrman and R. R. Richards-Kortum, Lab Chip, 2012, 12, 3082–3088 RSC.
- M. N. Tsaloglou, R. J. Watson, C. M. Rushworth, Y. Zhao, X. Niu, J. M. Sutton and H. Morgan, Analyst (Cambridge, U. K.), 2015, 140, 258–264 RSC.
- P. Valentini, R. Fiammengo, S. Sabella, M. Gariboldi, G. Maiorano, R. Cingolani and P. P. Pompa, ACS Nano, 2013, 7, 5530–5538 CrossRef CAS PubMed.
- R. Higuchi, C. Fockler, G. Dollinger and R. Watson, Biotechnology, 1993, 11, 1026–1030 CrossRef CAS PubMed.
- M. Zimmermann, H. Schmid, P. Hunziker and E. Delamarche, Lab Chip, 2007, 7, 119–125 RSC.
- A. W. Martinez, S. T. Phillips and G. M. Whitesides, Proc. Natl. Acad. Sci. U. S. A., 2008, 105, 19606–19611 CrossRef CAS PubMed.
- X. Mao and T. J. Huang, Lab Chip, 2012, 12, 1412–1416 RSC.
- K. Glynou, P. C. Ioannou, T. K. Christopoulos and V. Syriopoulou, Anal. Chem., 2003, 75, 4155–4160 CrossRef CAS PubMed.
- B. H. Weigl, R. Badrell, T. Schulte, F. Battrell and J. Hayenga, Biomed. Microdevices, 2001, 3, 267–274 CrossRef.
- B. Yao, G. A. Luo, X. Feng, W. Wang, L. X. Chen and Y. M. Wang, Lab Chip, 2004, 4, 603–607 RSC.
- N. J. Cira, J. Y. Ho, M. E. Dueck and D. B. Weibel, Lab Chip, 2012, 12, 1052–1059 RSC.
- I. K. Dimov, L. Basabe-Desmonts, J. L. Garcia-Cordero, B. M. Ross, Y. Park, A. J. Ricco and L. P. Lee, Lab Chip, 2011, 11, 845–850 RSC.
- K. Iwai, K. C. Shih, X. Lin, T. A. Brubaker, R. D. Sochol and L. Lin, Lab Chip, 2014, 14, 3790–3799 RSC.
- W. Yang, Y. G. Nam, B.-K. Lee, K. Han, T. H. Kwon and D. S. Kim, Jpn. J. Appl. Phys., 2010, 49, 06GM01 Search PubMed.
- X. Qiu, J. A. Thompson, Z. Chen, C. Liu, D. Chen, S. Ramprasad, M. G. Mauk, S. Ongagna, C. Barber, W. R. Abrams, D. Malamud, P. L. A. M. Corstjens and H. H. Bau, Biomed. Microdevices, 2009, 11, 1175–1186 CrossRef CAS PubMed.
- W. Li, T. Chen, Z. Chen, P. Fei, Z. Yu, Y. Pang and Y. Huang, Lab Chip, 2012, 12, 1587–1590 RSC.
- W. Wu, K. T. Trinh and N. Y. Lee, Analyst, 2012, 137, 983–990 RSC.
- M. M. Gong, B. D. Macdonald, T. Vu Nguyen and D. Sinton, Biomicrofluidics, 2012, 6, 44102 CrossRef PubMed.
- C. D. Chin, T. Laksanasopin, Y. K. Cheung, D. Steinmiller, V. Linder, H. Parsa, J. Wang, H. Moore, R. Rouse, G. Umviligihozo, E. Karita, L. Mwambarangwe, S. L. Braunstein, J. van de Wijgert, R. Sahabo, J. E. Justman, W. El-Sadr and S. K. Sia, Microfluidics-based diagnostics of infectious diseases in the developing world, Nat. Med., 2011, 17(8), 1015–1019 CrossRef CAS PubMed.
- X. Yang, C. Grosjean, Y. C. Tai and C. M. Ho, Sens. Actuators, A, 1998, 64, 101–108 CrossRef CAS.
- A. Meckes, J. Behrens, O. Kayser, W. Benecke, T. Becker and G. Muller, Sens. Actuators, A, 1999, 76, 478–483 CrossRef CAS.
- J. W. Choi, K. W. Oh, J. H. Thomas, W. R. Heineman, H. B. Halsall, J. H. Nevin, A. J. Helmicki, H. T. Henderson and C. H. Ahn, Lab Chip, 2002, 2, 27–30 RSC.
- W. van der Wijngaart, H. Ask, P. Enoksson and G. Stemme, Sens. Actuators, A, 2002, 100, 264–271 CrossRef CAS.
- M. M. Teymoori and E. Abbaspour-Sani, Sens. Actuators, A, 2005, 117, 222–229 CrossRef CAS.
- J. Peirs, D. Reynaerts and H. Van Brussel, Sens. Actuators, A, 2000, 85, 409–417 CrossRef CAS.
- Y. Shin, A. P. Perera, C. C. Wong and M. K. Park, Lab Chip, 2014, 14, 359–368 RSC.
-
N. De Nevers and R. Grahn, Fluid mechanics for chemical engineers, McGraw-Hill, 1991 Search PubMed.
-
J. Xia and W. K. Durfee, 52nd National Conference of Fluid Power, 2011 Search PubMed.
- H. M. Ji, V. Samper, Y. Chen, W. C. Hui, H. J. Lye, F. B. Mustafa, A. C. Lee, L. Cong, C. K. Heng and T. M. Lim, Sens. Actuators, A, 2007, 139, 139–144 CrossRef CAS.
- Y. Shin, A. P. Perera and M. K. Park, Sens. Actuators, B, 2013, 178, 200–206 CrossRef CAS.
Footnote |
† Electronic supplementary information (ESI) available. See DOI: 10.1039/c5lc00891c |
|
This journal is © The Royal Society of Chemistry 2016 |
Click here to see how this site uses Cookies. View our privacy policy here.