DOI:
10.1039/C4IB00197D
(Paper)
Integr. Biol., 2015,
7, 112-127
A developmentally inspired combined mechanical and biochemical signaling approach on zonal lineage commitment of mesenchymal stem cells in articular cartilage regeneration
Received
12th August 2014
, Accepted 2nd November 2014
First published on 5th November 2014
Abstract
Articular cartilage is organized into multiple zones including superficial, middle and calcified zones with distinct cellular and extracellular components to impart lubrication, compressive strength, and rigidity for load transmission to bone, respectively. During native cartilage tissue development, changes in biochemical, mechanical, and cellular factors direct the formation of stratified structure of articular cartilage. The objective of this work was to investigate the effect of combined gradients in cell density, matrix stiffness, and zone-specific growth factors on the zonal organization of articular cartilage. Human mesenchymal stem cells (hMSCs) were encapsulated in acrylate-functionalized lactide-chain-extended polyethylene glycol (SPELA) gels simulating cell density and stiffness of the superficial, middle and calcified zones. The cell-encapsulated gels were cultivated in a medium supplemented with growth factors specific to each zone and the expression of zone-specific markers was measured with incubation time. Encapsulation of 60 × 106 cells per mL hMSCs in a soft gel (80 kPa modulus) and cultivation with a combination of TGF-β1 (3 ng mL−1) and BMP-7 (100 ng mL−1) led to the expression of markers for the superficial zone. Conversely, encapsulation of 15 × 106 cells per mL hMSCs in a stiff gel (320 MPa modulus) and cultivation with a combination of TGF-β1 (30 ng mL−1) and hydroxyapatite (3%) led to the expression of markers for the calcified zone. Further, encapsulation of 20 × 106 cells per mL hMSCs in a gel with 2.1 MPa modulus and cultivation with a combination of TGF-β1 (30 ng mL−1) and IGF-1 (100 ng mL−1) led to up-regulation of the middle zone markers. Results demonstrate that a developmental approach with gradients in cell density, matrix stiffness, and zone-specific growth factors can potentially regenerate zonal structure of the articular cartilage.
Insight, innovation, integration
Strategies that mimic zonal organization of the articular cartilage with complex interactions between resident cells, extracellular matrix and soluble growth factors are more likely to create an engineered tissue with more effective clinical outcome. A developmentally inspired approach is proposed to in vitro regeneration of articular cartilage tissue by recapitulating biochemical, mechanical, and cellular regulatory factors during cartilage development. Combination of TGF-β1 and BMP-7 in a soft gel with a relatively high cell seeding density stimulated chondrogenic differentiation of human mesenchymal stem cells (hMSCs) to the superficial zone phenotype of articular cartilage. Conversely, combination of TGF-β1 and hydroxyapatite in a stiff gel with a relatively low cell seeding density stimulated chondrogenic differentiation of hMSCs to the calcified zone phenotype. This work could potentially enable the design of effective multilayer grafts as a more sustainable solution to regeneration of articular cartilage defects.
|
Introduction
Articular cartilage also called hyaline cartilage is a multi-functional tissue with a highly organized structure which provides a lubricating surface for the gliding joints and a load-bearing matrix attached to the underlying bone.1,2 The postnatal articular cartilage due to its avascular nature and low metabolism lacks the ability for complete self-repair through native healing mechanisms.3 About 70% of people over the age of 65 suffer from degenerative joint disease or osteoarthritis (OA).4 The center for disease control and prevention estimates that nearly 27 million Americans suffer from joint pain and stiffness, loss of function and disability.5 Clinical approaches to articular cartilage repair include debridement, lavage and joint replacement in advanced forms of OA.3 Current treatment methods rarely restore the full function to the joint.3 The challenge in regeneration of articular cartilage is recreating the spatially variant structure of the native tissue with complex interactions between resident cells, the extracellular matrix (ECM) and soluble growth factors. Several studies have reported construction of a homogenous matrix with bulk properties of the native cartilage tissue for implantation in a cartilage defect using a top-down approach in which chondrogenic cells were seeded into preformed porous scaffolds or embedded in injectable matrices loaded with signaling factors.4,6–8 The generated tissues lacked the desired functions because top-down approaches do not mimic the intricate zonal organization of the native articular cartilage.
Articular cartilage is structurally and functionally composed of multiple distinct zones including superficial, middle, deep, and calcified zones1,2,9 with each zone exhibiting a defined ECM composition and organization.1,3 Recent studies have attempted to mimic this spatial organization using the bottom-up approach with zone-specific chondrocytes isolated from animal tissues.10,11 Ng and coworkers used zone-specific chondrocytes seeded on layered agarose hydrogels to recreate depth-dependent anisotropy of the articular cartilage.10 Sharma and collaborators used bilayer hydrogels to reorganize zone-specific chondrocytes into a stratified structure.12 Cui and collaborators printed human chondrocytes into osteochondral plugs layer-by-layer to repair cartilage defects.13 Although chondrocytes are the most relevant cell source for regeneration of articular cartilage defects, there is limited quantity of autologous chondrocytes for transplantation. In addition, expansion of chondrocytes in monolayer culture causes dedifferentiation with decreased proteoglycan and collagen type II (Col II) synthesis.4,14–16 An attractive alternative is the use of mesenchymal stem cells (MSCs) harvested from the patient's bone marrow or adipose tissue.4 MSCs can be expanded and differentiated into zone-specific chondrocytes by seeding them in scaffolds that mimic the mechanical and biochemical properties of the articular cartilage and cultivated in a medium supplemented with chondrogenic growth factors.17 Several approaches including multilayer hydrogels13,18,19 and multilayer electrospun fibers20,21 have been applied to create scaffolds mimicking the composition and mechanical properties of the native articular cartilage. Steele et al. generated bilayer poly(ε-caprolactone) (PCL) scaffolds comprised of an aligned fiber layer mimicking the morphology and mechanics of the superficial zone laminated to a porous scaffold simulating the middle zone of articular cartilage.21
The articular cartilage zones are maintained by distinct cellular phenotypes, ECM compositions, and growth factors. The superficial zone is characterized by thin collagen fibrils parallel to the articular surface and the chondrocytes in this zone have high expression of Sox-9, an early chondrogenic differentiation marker, and superficial zone protein (SZP) for lubricating the joint during movement.22 The middle zone which takes up as much as 60% of the cartilage volume is composed of collagen fibrils thicker than those in the superficial zone with oblique alignment to the cartilage surface. The middle zone provides compressive strength and dimensional stability and it is characterized by pre-hypertrophic chondrocytes with high expression of glycosaminoglycans (GAG) and aggrecans (AGC).3,18 The deep zone consists of large diameter fibrils oriented perpendicular to the articulating surface (similar to the calcified zone) with functions similar to the middle zone. Chondrocytes in the deep zone express the highest amount of proteoglycans.23 The calcified zone is an avascular tissue at the interface between uncalcified hyaline cartilage and subchondral bone and collagen fibers in this zone are oriented perpendicular to the articular surface. The calcified cartilage has a unique mineralized matrix composition, with chondrocytes expressing markers of hypertrophy including collagen type X (Col X) and alkaline phosphatase (ALP).3,5,18 To limit the scope of the study and the number of experimental groups, we focused on those zones that provided unique functions to the articular cartilage, namely superficial, middle, and calcified zones.
In mature articular cartilage tissue the average modulus increases significantly from 0.08 MPa in the superficial zone to 2.1 and 320 MPa in the middle and calcified zones, respectively.5,24 Conversely, the cell density decreases significantly from 60 × 106 cells per mL in the superficial zone to 20 × 106 and 15 × 106 cells per mL in the middle and calcified zones, respectively.25 Members of the transforming growth factor-β (TGF-β) family are used extensively in tissue engineering of articular cartilage to induce chondrogenic differentiation of MSCs. In addition to TGF-β, several other growth factors are known to direct the formation of a stratified structure of articular cartilage during native tissue development.1,2,4,26 Cultivation of MSCs with TGF-β1 (3 ng mL−1) and BMP-7 (300 ng mL−1) induced the synthesis of SZP protein, a marker for chondrocytes in the superficial zone22 whereas IGF-1 did not.27,28 Similar results were obtained when bovine articular cartilage was treated with TGF-β1 and BMP-7.29 Cultivation of chondrocytes isolated from the middle zone with TGF-β1 (30 ng mL−1) and IGF-1 (100 ng mL−1) significantly increased collagen synthesis.30–32 TGF-β1 also contributes to the maintenance of calcified cartilage zone as deletion of TGF-β1 receptor gene from chondrocytes delayed endochondral ossification33 and cultivation of bovine hypertrophic chondrocytes with a combination of TGF-β1 (30 ng mL−1) and 3% (w/v) hydroxyapatite (HA) increased matrix deposition and mineralization.33,34
Strategies that mimic zonal organization of the articular cartilage are more likely to create an engineered tissue with more effective clinical outcome. In this work, we propose a developmentally inspired approach to in vitro regeneration of articular cartilage tissue by recapitulating biochemical and biomechanical regulatory factors during cartilage development. In this regard, the superficial zone was simulated in this work by encapsulating 60 × 106 cells per mL human mesenchymal stem cells (hMSCs) in an 80 kPa gel loaded with 3 ng mL−1 TGF-β1 and 100 ng mL−1 BMP-7; the middle zone was simulated by encapsulating 20 × 106 cells per mL hMSCs in a 2.1 MPa gel loaded with 30 ng mL−1 TGF-β1 and 100 ng mL−1 IGF-1; and the calcified zone was simulated by encapsulating 15 × 106 cells per mL hMSCs in a 320 MPa gel reinforced with nanofibers aligned perpendicular to the articular surface and loaded with 30 ng mL−1 TGF-β1 and 3% HA. Although natural gels such as collagen,35 alginate,36 hyaluronic acid, and chitosan37 have been used for cartilage tissue engineering, the stiffness and resorption rate of those matrices cannot be tuned to the specific requirements of each zone. Polyethylene glycol (PEG) gels are inert, non-immunogenic, and compatible with encapsulation of MSCs.38,39 Recently, we reported that PEG macromers chain-extended with short hydroxy acid segments, like L-lactide or glycolide, generate micellar hydrogels with a wide range of stiffness from 1 to 2000 kPa and resorption times from a few days to a few months.40 In this work, we used the lactide-chain-extended PEG gels functionalized with acrylate groups (SPELA) to experimentally simulate the synergistic effect of matrix stiffness, cell density, and supplementing the culture medium with growth factors corresponding to those in the superficial, middle and calcified zones on lineage commitment of the encapsulated hMSCs.
Experimental
Materials
Polyethylene glycol (PEG, nominal molecular weights 4.6 kDa), dichloromethane (DCM), N,N-dimethylformamide (DMF), diisopropylcarbodiimide (DIC), 4-dimethylaminopyridine (DMAP), trifluoroacetic acid (TFA), triisopropylsilane (TIPS), diethyl ether, and hexane were purchased from Acros (Fairfield, OH). The Rink Amide NovaGel™ resin, all Fmoc-protected amino acids, and hydroxybenzotriazole (HOBt) were purchased from Novabiochem (EMD Biosciences, San Diego, CA). Calcium hydride, triethylamine (TEA), paraformaldehyde, 4,6-diamidino-2-phenylindole (DAPI), insulin, penicillin, streptomycin, L-proline, ascorbic acid, sodium pyruvate, insulin transferrin selenium + ITS Premix, and β-glycerol phosphate were purchased from Sigma-Aldrich (St. Louis, MO). Acetomethoxy derivative of calcein (cAM) and ethidium homodimer (EthD) were purchased from Molecular Probes (Life Technologies, Grand Island, NY). Insulin growth factor-1 (IGF-1) and Transforming growth factor-β1 (TGF-β1) were purchased from Lonza (Allendale, NJ) and Bone morphogenetic protein-7 (BMP-7) was purchased from Novus (Littleton, CO). Bovine serum albumin (BSA) was purchased from Jackson ImmunoResearch (West Grove, PA). Dulbecco's phosphate-buffer saline (PBS), trypsin-EDTA, DMEM cell culture medium, fetal bovine serum (FBS), Alexa Fluor 594 Phalloidin, and the Quant-it Pico-Green dsDNA reagent kit were purchased from Invitrogen (Carlsbad, CA). The Spectro/Por dialysis tube (molecular weight cutoff 3.5 kDa) was purchased from Spectrum Laboratories (Rancho Dominquez, CA). DCM was purified by distillation over calcium hydride. All other solvents were reagent grade and were used as received without further purification.
Synthesis and gelation of SPELA hydrogels
Previous clinical studies indicate that regeneration of the superficial layer of articular cartilage occurs 3–4 weeks post-surgery41 while full thickness regeneration and complete load bearing occur at 8–12 weeks post-surgery.41,42 Therefore in this study SPELA gels with resorption times of 4, 8 and 12 weeks were synthesized to simulate the turnover rate of the superficial, middle, and calcified zones, respectively. The SPELA macromers were synthesized in two steps by condensation polymerization of PEG with the L-lactide monomer followed by reaction with acryloyl chloride as we previously described.38,43 The number of lactide monomers at each macromer chain-end was varied by changing the lactide to PEG molar ratio. Compressive modulus of the gel was tuned to the value corresponding to one of the cartilage zones by varying SPELA macromer concentration in aqueous solution as we previously described.38,43 Based on the desired resorption rate and compressive modulus, the second and third columns of Table 1 specify SPELA compositions simulating the superficial, middle, and calcified zones.40 The notation “SPELAn (m%)” represents the m% SPELA macromer in the hydrogel precursor solution and the lactide to PEG ratio of n. An acrylamide-terminated glycine-arginine-glycine-aspartic acid peptide (Ac-GRGD) was synthesized on Rink Amide NovaGel™ resin in the solid phase, purified by high-performance liquid chromatography (HPLC), and characterized by electrospray ionization (ESI) mass spectrometry as we previously described.44 The Ac-GRGD peptide in the amount of 2 wt%, based on SPELA macromer weight, was added to the hydrogel precursor solution to facilitate cell adhesion. The precursor solution was crosslinked by ultraviolet (UV) polymerization with 4-(2-hydroxyethoxy) phenyl-(2-hydroxy-2-propyl) ketone (Irgacure 2959; CIBA, Tarrytown, NY) as a photoinitiator to form a hydrogel. The precursor solution was prepared by adding the initiator solution (5 mg initiator in 1 mL PBS) to the solution of the SPELA macromer in PBS. Next, the solution was degassed, transferred to a Teflon mold, covered with a transparent glass plate and fastened with clips. Then, the precursor solution was irradiated with a BLAK-RAY 100-W mercury long wavelength (365 nm) UV lamp (Model B100-AP; UVP, Upland, CA) for 200 seconds. Fiber-reinforced hydrogels were fabricated to form matrices with compressive modulus of 320 MPa in which aligned nanofiber microsheets were electrospun from a 10 wt% solution of poly(DL-lactide) (PLA, Durect, Birmingham, AL, 95 kDa MW) in 1,1,1,3,3,3-hexafluoro-2-propanol (HFIP, VWR). The injection rate, electrical potential, and needle-to-collector distance were 1.0 mL h−1, 20 kV, and 7.5 cm, respectively, and the fibers were collected on a rotating wheel at 1200 rpm as we previously described.45,46 Next, the fiber microsheets were dip-coated in 35 wt% SPELA5 hydrogel precursor solution, wrapped around a 5 mm diameter stainless steel rod, and crosslinked as described above. After crosslinking, the disk-shape samples were cut from the gel using an 8 mm cork borer and the cut samples were equilibrated in PBS for 24 h at 37 °C.
Table 1 Gel composition and degradation time, matrix stiffness, type, concentration and exposure time of growth factors, and hMSC cell density for simulating superficial, middle, and calcified zones of articular cartilage
Simulated cartilage zone |
Gel composition and degradation time |
Matrix stiffness |
Growth factors (GF) |
GF exposure time (days) |
Cell density (cells per mL) |
Superficial |
SPELA10 (15%) 4 weeks |
80 kPa |
TGFβ1 (3 ng mL−1) BMP-7 (100 ng mL−1) |
10 |
60 × 106 |
Middle |
SPELA7.5 (50%) 8 weeks |
2.1 MPa |
TGFβ1 (30 ng mL−1) IGF-1 (100 ng mL−1) |
21 |
20 × 106 |
Calcified |
PLA/SPELA5 (35%) fiber composite 3% HA 12 weeks |
320 MPa |
TGFβ1 (30 ng mL−1) |
21 |
15 × 106 |
A rheometer (TA Instruments, New Castle, DE) was used to measure compressive modulus of the gel samples at a uniaxial displacement rate of 7.5 mm s−1. The slope of the linear line fitted to the stress–strain curve at 5–10% strain was taken as compressive modulus (E).47 To measure mass loss, the equilibrated gel samples were dried under vacuum at 40 °C. Next, the samples were incubated in the basal medium (5 mL per sample) without FBS at 37 °C and under mild agitation. At each time point, samples were washed and dried under vacuum. The dried sample weights were measured and compared with the initial dry weights to determine fractional mass remaining.38
Encapsulation and cultivation of hMSCs in SPELA hydrogels
Mechanical, biochemical, and cellular requirements for the superficial, middle, and calcified zones of articular cartilage are summarized in Table 1. hMSCs were cultivated at 5000 cells per cm2 in a high glucose DMEM medium supplemented with 10% FBS, 100 units per mL penicillin and 100 μg mL−1 streptomycin (basal medium). All hMSCs were passaged <5 times prior to cell encapsulation according to supplier's instructions (Lanza, Allendale, NJ). For cell encapsulation, the sterile initiator and macromer solutions were mixed, the suspension of hMSCs in 100 μL DMEM was added to the precursor solution and mixed with a pre-sterilized glass rod as we described previously.40 For the calcified zone gel, prior to cell addition, 3% (W/V) hydroxyapatite (HA) was suspended in the hydrogel precursor solution by sonication (0.5 h at 20 kHz) as we previously described.48 The precursor cell suspension was injected between two sterile microscope glass slides and crosslinked by UV irradiation for 200 s. After crosslinking, the disk-shape samples were incubated in the basal medium for 24 h with two medium changes. Next, the medium was replaced with the chondrogenic medium consisting of DMEM (4.5 g mL−1 glucose, 50 μg mL−1L-proline, 50 μg mL−1 ascorbic acid, 0.1 mM sodium pyruvate, 1% v/v insulin–transferrin–selenium (ITS) premix)21 and supplemented with the corresponding chondroinductive growth factors to each zone as specified in Table 1. Recent studies indicate that hypertrophy in chondrogenic differentiation of hMSCs is initiated in days 9–128,9 which is marked by a significant increase in Col X expression. Therefore, the medium for hMSCs in the superficial zone gel was supplemented with the appropriate growth factors for 10 days to prevent hypertrophy whereas the medium for hMSCs in the middle and calcified zone gels was supplemented with the appropriate growth factors for 21 days. After day 10, the medium for hMSCs in the superficial zone gel was replaced with the basal medium. Cell cultures were incubated at 37 °C and 5% CO2 for 3 weeks.
The experimental groups included hMSCs encapsulated in the gel simulating the superficial zone and cultured in the chondrogenic medium supplemented with 3 ng mL−1 TGF-β1 and 100 ng mL−1 BMP-7 (hereafter referred to as the “Superficial gel”), hMSCs encapsulated in the gel simulating the middle zone and cultured in the chondrogenic medium supplemented with 30 ng mL−1 TGF-β1 and 100 ng mL−1 IGF-1 (hereafter referred to as the “Middle gel”), hMSCs encapsulated in the gel simulating the calcified zone and cultured in the chondrogenic medium supplemented with 30 ng mL−1 TGF-β1 and 10 mM β-glycerol phosphate (hereafter referred to as the “Calcified gel”),49,50 and the control gel group. The control group was hMSCs encapsulated in the gel simulating the superficial zone (80 kPa modulus) but cultured in the basal medium (no TGF-β1 or BMP-7). The cellular hydrogels were cultured for up to 21 days. At each time point, samples were assessed with respect to cell content, total collagen content, GAG, ALP activity, and markers specific to each cartilage zone biochemically, histologically and by mRNA analysis.
Viability of the encapsulated cells
For cell viability, disks were incubated with the acetomethoxy derivative of calcein (cAM) and ethidium homodimer (EthD) (1 μg mL−1, Life Technologies, Grand Island, NY) to stain live and dead cells, respectively, as described.38 Stained samples were imaged using an inverted fluorescent microscope (Nikon Eclipse Ti-ε, Nikon, Melville, NY). For imaging the encapsulated cells, gels were rinsed twice with PBS and fixed with 4% paraformaldehyde for 3 h. After fixation, cells were permeabilized using PBS containing 0.1% Triton X-100 for 5 min, rinsed, and incubated with Alexa 488 phalloidin (1
:
200 dilution) and DAPI (1
:
5000 dilution) to stain actin filaments of the cell cytoskeleton and cell nuclei, respectively. Stained samples were imaged using a Nikon Eclipse Ti-ε inverted fluorescent microscope as described.45
Histology
The day 21 samples were fixed in formalin, embedded in paraffin, and sectioned using a microtome to a thickness of 10 μm as described.51 An improved cryosection method described by Ruan et al.52 based on a protein solution in place of sucrose solution in the dehydration step was used to produce less brittle blocks for sectioning. The sections were stained with Hematoxylin and Eosin (H&E) to ascertain morphology of the encapsulated cells and stained with Alcian blue to image GAG. Whole gels were also stained with Alcian blue and Alizarin red to image the extent of GAG accumulation and mineral deposits, respectively, in hMSC encapsulated gels.18 Histological sections were imaged using a Nikon Optiphot Epifluorescence System at the same instrument settings.
DNA content and ALP activity
At each time point, the gel samples were homogenized and sonicated to rupture membranes of the encapsulated cells. Double stranded DNA (dsDNA) content of the samples was measured using QuantiGreen assay (Molecular Probes) according to manufacturer's instructions. Samples were homogenized using the kit lysis buffer. 100 μL of the cell lysate was added to the kit's working solution and florescence was measured after 4 min incubation under ambient conditions using a Synergy-HT plate reader at emission and excitation wavelengths of 485 and 528 nm, respectively.44 Measured intensities were correlated to the equivalent amount of dsDNA using a calibration curve constructed with DNA standard solutions.38 ALP activity was assessed using the QuantiChrom ALP Assay Kit according to manufacturer's instructions. Briefly 190 μL of the kit's reagent solution containing 10 mM p-nitrophenyl phosphate and 5 mM magnesium acetate was loaded to each well of a 96 well plate, 10 μL aliquot of the homogenized sample was added, and absorbance was recorded at time zero and again after 4 minutes at 405 nm wavelength.44 ALP activity was calculated using the equation [(At=4 − At=0)/(Acalibrator − AddH2O) × 808]. The measured ALP activities were normalized to DNA content.38
Total collagen and GAG content
Total collagen content was assayed based on selective binding of the G–X–Y amino acid sequence of collagen to Sircol dye, according to manufacturer's instructions. Briefly 1 mL of the Sircol dye was added to the sonicated cell lysate, incubated for 30 minutes and centrifuged at 10
000 rpm for 5 min to separate the collagen–dye complex. After removing supernatant, the collagen–dye complex was mixed with 1 mL Sircol Alkali reagent and absorbance was measured on a plate reader at 555 nm wavelength. The assayed collagen was calibrated using reagent blanks and the kit's standard collagen solution, and normalized to DNA content. GAG production in the gel samples was determined using a dimethylmethylene blue (DMMB) assay. Briefly, the gel samples after washing twice with PBS were digested with 1 mM EDTA, 2 mM dithiothreitol and 300 μg papain in 1 mL of 20 mM sodium phosphate buffer (pH 6.8). After incubation at 60 °C for 60 min, iodoacetic acid was added to 10 mM final concentration and the volume was adjusted to 5 mL by addition of 50 mM Tris buffer. The color reagent was prepared by dissolving 16 mg dimethylmethylene blue to 1 L water containing 3 g glycine, 2.37 g NaCl and 95 ml of 0.1 M HCl to the final pH of 3. Next, 2.5 mL of the color reagent was added to 100 μL of each sample and absorbance was recorded after 10 minutes at 525 nm wavelength. The assay was calibrated using reagent blanks and serial dilutions of the standard solution of whale chondroitin sulfate, and normalized to DNA content.18
mRNA analysis
Markers included SOX-9 as a master regulator of chondrogenesis,53 SZP as a marker for the superficial zone,22 Col II and AGC as ECM components highly expressed in the middle zone,18 and Col X and ALP as markers for cartilage hypertrophy in the calcified zone.3 At each time point, total RNA of the homogenized samples was isolated using Trizol44 and genomic DNA was removed using deoxyribonuclease I (Invitrogen) according to manufacturer's instructions. After converting RNA (250 ng) using the Promega reverse transcription system (Madison, WI), the converted cDNA was amplified by RT-qPCR with SYBR green RealMasterMix (Eppendorf, Hamburg, Germany) using the Bio-Rad CXF96 PCR system (Bio-Rad, Hercules, CA) and the appropriate gene specific primers. Primers were designed and selected using Primer3 web-based software as described.54 The list of primer sequences is provided in Table 2. Data were analyzed using the ΔΔct Real time analysis method.38 mRNA expressions were normalized against GAPDH as a house keeping reference gene and fold changes were compared to those in the same group at day zero.
Table 2 Reverse and forward sequences for PCR primers
PCR primer |
Forward |
Reverse |
SZP |
5′AACCACCACCAGACCTAACCAA3′ |
5′CAGCACCACCTGCATCTTCA3′ |
Sox-9 |
5′CCCCAACAGATCGCCTACAGT3′ |
5′GAGTTCTGGTGGTCGGTGTAGTC3′ |
AGC |
5′TCGAGGACAGCGAGGCC3′ |
5′TCGAGGGTGTAGCGTGTAGAGA3′ |
Col II |
5′AGCCTGGTGTCATGGGTTTC3′ |
5′GTCCCTTCTCACCAGCTTTGC3′ |
Col X |
5′CTGTATAAGAATGGCACCCCTGTA3′ |
5′GCACTCCCTGAAGCCTGATC3′ |
ALP |
5′ATGGGATGGGTGTCTCCACA3′ |
5′CCACGAAGGGGAACTTGTC3′ |
GAPDH |
5′CATGACAACTTTGGTATCGTGG3′ |
5′CCTGCTTCACCACCTTCTTG3′ |
Statistical analysis
All experiments were done in triplicate and quantitative data expressed as means ± standard deviation. Significant differences between groups were evaluated using a two-way ANOVA with replication test, followed by a two-tailed Students t-test. A value of p < 0.05 was considered statistically significant.
Results
Characterization of the hydrogels
Fig. 1A shows degradation rate of SPELAn hydrogels at 37 °C. Mass loss of SPELA5, SPELA7.5 and SPELA10 hydrogels after 7 days of incubation was 9%, 13% and 18%, respectively. Further, mass loss of the hydrogels was between 17% and 32% after 14 days and between 29% and 47% after 21 days (Fig. 1A). We previously showed that water content of SPELA gels did not change significantly with lactide segment length.40 Therefore, the 2- and 1.5-fold higher mass loss of SPELA10 and SPELA7.5, respectively, compared to SPELA5 after 7 days was due to lactide segment length, not due to differences in water content. The effect of macromer concentration on compressive modulus of SPELAn hydrogels is shown in Fig. 1B. The increase in modulus with concentration was attributed to higher crosslink density of the gels as modulus was directly related to density of active crosslinks in the gel network.55 The gel with 15 wt% SPELA10 concentration simulated compressive modulus of the superficial zone (80 kPa, open square). Likewise, the gel with 50 wt% SPELA7.5 concentration simulated modulus of the middle zone (2.1 MPa, open diamond). The gel with 35 wt% SPELA7.5 concentration reinforced with PLA nanofibers simulated modulus of the calcified zone (320 MPa, open circle).
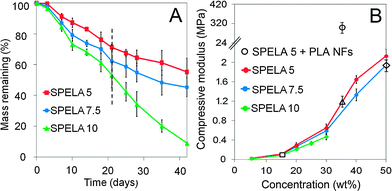 |
| Fig. 1 (A) Degradation of SPELA5 (red), SPELA7.5 (blue), and SPELA10 (green) gels with incubation time in the basal medium at 37 °C. The dashed line shows remaining mass of the gels after 21 days of incubation. (B) Compressive modulus of SPELA5 (red), SPELA7.5 (blue), and SPELA10 (green) gels versus SPELA macromer concentration. The square shows modulus of 15% SPELA10 gel simulating the superficial zone; the triangle and circle show modulus of 35% SPELA5 gel before and after reinforcement with nanofibers aligned perpendicular to the gel surface, respectively, to simulate the calcified zone; and the diamond shows modulus of 50% SPELA7.5 gel simulating the middle zone. Error bars correspond to means ± 1 SD for n = 3. | |
Cell viability and histological characterization of the cellular gels
Images in Fig. 2A–D compare live (stained green) and dead (stained red) stained hMSCs encapsulated in Superficial (B), Middle (C), and Calcified (D) gels after 21 days of incubation in the chondrogenic medium with the control group cultivated in the basal medium (A). The inset in Fig. 2D shows cell clusters around nanofibers. Images in Fig. 2E–H compare phalloidin (cytoskeleton, purple) and DAPI (nuclei, blue) stained hMSCs encapsulated in Superficial (F), Middle (G) and Calcified (H) gels after 21 days of incubation in the chondrogenic medium with the control group cultivated in the basal medium (E). Insets in Fig. 2E–H show morphology of the encapsulated cells at higher magnification. hMSCs encapsulated in Superficial (Fig. 2E) and Middle (Fig. 2F) gels were rounded consistent with their morphology in the native tissue.4,5Fig. 2H shows morphology of the cells in Calcified gel in merged bright and dark field image of the whole gel. The inset in Fig. 2H shows extensive cell clustering adjacent to aligned nanofibers in the Calcified gel.
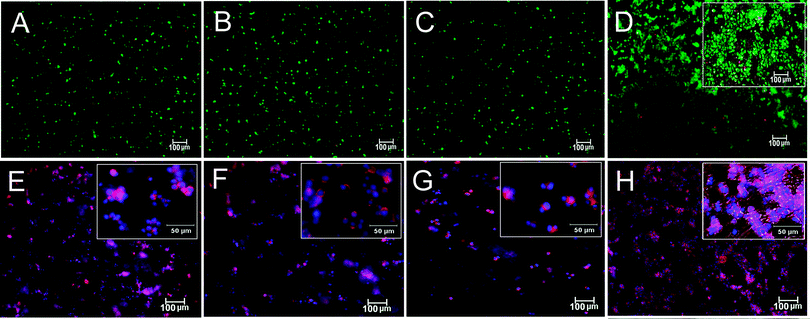 |
| Fig. 2 Calcein AM (live, green) and EthD (dead, red) staining of the hMSCs encapsulated in Superficial (B), Middle (C), and Calcified (D) gels and incubated in the chondrogenic medium supplemented with zone-specific growth factors. Phalloidin (purple for cytoskeleton) and DAPI (blue for cell nucleus) images of the hMSCs encapsulated in Superficial (F), Middle (G), and Calcified (H) gels and incubated in the chondrogenic medium supplemented with zone-specific growth factors. Images A and E are for hMSCs encapsulated in the 80 kPa gel and incubated in the basal medium as the control. The insets are higher magnification images. | |
Images in Fig. 3A–D compare GAG stained histological sections (Alcian blue) of hMSCs encapsulated in Superficial (B), Middle (C) and Calcified (D) gels after 21 days incubation in the chondrogenic medium with the control group cultivated in the basal medium (A). Fig. 3E–H compare H&E stained histological sections of hMSCs encapsulated in Superficial (F), Middle (G) and Calcified (H) gels after 21 days incubation in the chondrogenic medium with the control group cultivated in the basal medium (E). The rounded cells increased in size from Superficial (Fig. 3F) to Middle (Fig. 3G) and Calcified (Fig. 3H) gels compared to that cultivated in the basal medium (Fig. 3E). The cells in the Calcified gel were in isolated Lacunae consistent with their morphology in the native calcified layer of articular cartilage tissue. However, the observed cell clusters in images of the whole Calcified gel in Fig. 2(D) and (H) were not observed to the same extent in histological sections of Fig. 3. We believe that the fibers and their associated cell clusters, due to higher fiber stiffness, pulled out of the hydrogel matrix during sectioning, which led to unmatched microscopic images in Fig. 2 and 3 for the Calcified gel. Coburn et al.56 previously reported changes in cell morphology at the fiber/gel interface and McCullen et al.20 reported alignment of cell clusters along the fiber direction. Images in Fig. 4A–D compare GAG staining of the whole Superficial (B), Middle (C) and Calcified (D) gels after 21 days incubation in the chondrogenic medium with the control cultivated in the basal medium (A). GAG content of the whole samples progressively increased from Superficial (B with lighter blue) to Middle (C) and Calcified (D with darker blue) gels compared to those gels cultured in the basal medium (A with the lightest blue). Images in Fig. 4E–H compare Alizarin red staining for the whole Superficial (F), Middle (G), and Calcified (H) gels after 21 days incubation in the chondrogenic medium with the control cultivated in the basal medium (E). Fig. 4H shows high mineral deposits in Calcified gel with minor amounts in Superficial and Middle gels (Fig. 4F and G).
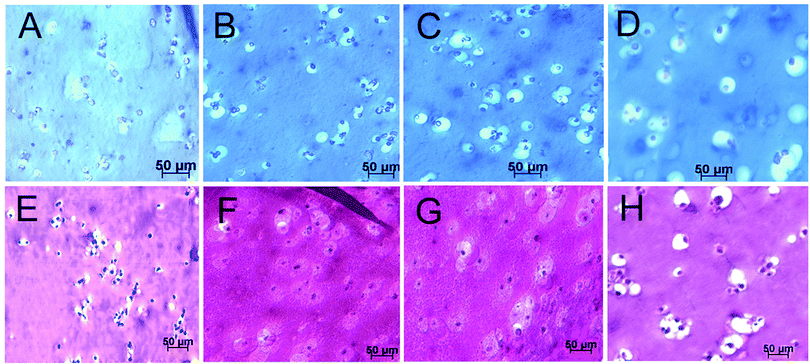 |
| Fig. 3 Alcian blue (GAG) stained histological sections of the hMSCs encapsulated in Superficial (B), Middle (C), and Calcified (D) gels and incubated in the chondrogenic medium supplemented with zone-specific growth factors. H&E stained sections for the hMSCs encapsulated in Superficial (F), Middle (G), and Calcified (H) gels and incubated in the chondrogenic medium supplemented with zone-specific growth factors. Images A and E are for the hMSCs encapsulated in the 80 kPa gel and incubated in the basal medium as the control. | |
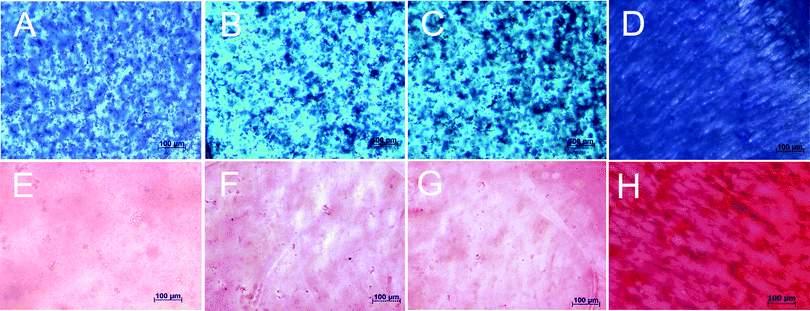 |
| Fig. 4 Alcian blue (GAG) staining of whole gels showing the extent of GAG accumulation for the hMSCs encapsulated in Superficial (B), Middle (C), and Calcified (D) gels and incubated in the chondrogenic medium supplemented with zone-specific growth factors. Alizarin red staining of whole gels showing mineral deposition of the hMSCs encapsulated in Superficial (F), Middle (G), and Calcified (H) gels and incubated in the chondrogenic medium supplemented with zone-specific growth factors. Images A and E are for hMSCs encapsulated in the 80 kPa gel and incubated in the basal medium as the control. | |
Analysis of mRNA expressions
mRNA expression of AGC, Col II, Col X, ALP, SZP, and Sox-9 for hMSCs encapsulated in the gels is shown in Fig. 5A to F, respectively. mRNA expression of AGC and Col II as the main components of cartilage ECM (Fig. 5A and B, respectively) showed an increasing trend with time for all groups. hMSCs encapsulated in the Middle gel showed highest expression levels of AGC and Col II with 112 and 45-fold increase in expression on day 21, respectively. Col X mRNA expression of hMSCs in Superficial, Middle and Calcified gels (Fig. 5C) was 0.96 ± 0.04, 4.3 ± 0.3, and 16 ± 1 on day 14, respectively, and increased to 1.05 ± 0.03, 7.3 ± 0.5, and 26 ± 2 on day 21. On days 14 and 21, Calcified and Superficial gels had highest and lowest expression of Col X, respectively. Col X mRNA expressions of hMSCs were in agreement with ALP mRNA expressions as shown in Fig. 5D. The calcified gel showed the highest ALP expression level for all time points whereas Superficial and Middle gels had ALP expression levels similar to the control for all time points. Fig. 5E shows mRNA expression of SZP, as a marker of the superficial zone, for the encapsulated hMSCs. The superficial gel showed the highest SZP expression level relative to Middle and Calcified gels and the expression level had an increasing trend with incubation time during the exposure period of BMP-7 and TGF-β (first 10 days). There was no significant difference in SZP expression of hMSCs in the Calcified gel relative to the control for all time points.
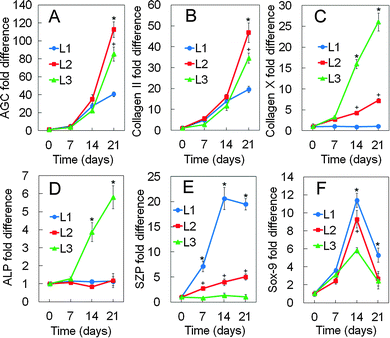 |
| Fig. 5 mRNA expression of AGC (A), Col II (B), Col X (C), ALP (D), SZP (E), and Sox-9 (F) for the hMSCs encapsulated in Superficial (L1, blue), Middle (L2, red), and Calcified (L3, green) gels with incubation time in the chondrogenic medium supplemented with zone-specific growth factors. A star indicates a statically significant difference between the test and all other groups at each time point. Error bars correspond to means ± 1 SD for n = 3. | |
SOX-9 mRNA expression of hMSCs as a major regulator of chondrogenesis showed a biphasic trend with incubation time for all gels. SOX-9 expression increased significantly in the first 14 days of incubation and then decreased on day 21 (Fig. 5F). hMSCs in the Superficial gel showed the highest SOX-9 expression level for all time points relative to Middle and Calcified gels.
Biochemical analysis of cellular gels
DNA (A), GAG (B), total collagen content (C) and ALP activity (D) of hMSCs encapsulated in Superficial (L1), Middle (L2), and Calcified (L3) gels with incubation time are shown in Fig. 6A to D, respectively. DNA content of the hMSCs decreased slightly with incubation time for all gels (Fig. 6A). GAG content of the hMSCs showed an increasing trend with incubation time for all gels with higher GAG content in Middle and Calcified gels relative to the Superficial gel (Fig. 6B). Total collagen content of the hMSCs showed an increasing trend with incubation time for all gels with higher collagen content in the Middle gel relative to Superficial and Calcified gels (Fig. 6C). ALP activity of hMSCs also showed an increasing trend with incubation time with the Calcified gel having orders of magnitude higher ALP activity relative to Superficial and Middle gels (Fig. 6D). The biochemical results for GAG/collagen (Fig. 6), in particular higher GAG/collagen of the hMSCs in the Middle gel, were consistent with mRNA expressions in Fig. 5. hMSCs encapsulated in the gel simulating the calcified zone showed the highest increase in ALP activity with time relative to Superficial and Middle gels reaching 1030 IU/mg DNA on day 21 (Fig. 6D).
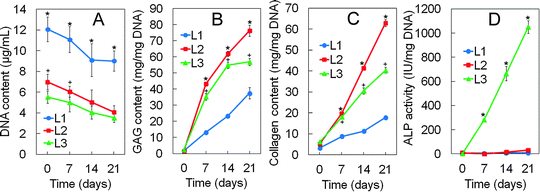 |
| Fig. 6 DNA content (A), GAG content (B), total collagen content (C), and ALP activity (D) for the hMSCs encapsulated in Superficial (L1, blue), Middle (L2, red), and Calcified (L3, green) gels with incubation time in the chondrogenic medium supplemented with zone-specific growth factors. A star indicates a statistically significant difference between the test and all other groups at each time point. Error bars correspond to means ± 1 SD for n = 3. | |
Discussion
The structure of articular cartilage tissue is anisotropic and spatially variant with complex interactions between resident cells, ECM, and soluble growth factors. This anisotropy is integral of the function of cartilage tissue including lubrication, compressive strength, and a rigid interface for load transmission to the underlying bone.1,4,20 Despite the importance of zonal organization of articular cartilage on tissue function, a viable approach to zonal regeneration of the tissue is not established. In this work, we propose a developmental approach to zonal regeneration of articular cartilage by recapitulating the contribution of biochemical, mechanical, and cellular regulatory factors during cartilage development. The organization of articular cartilage is initiated from the early stages of development by stimulation of undifferentiated MSCs in the lateral plate mesoderm followed by condensation, lineage commitment and eventually differentiation into mature chondrocytes (Fig. 7A).2,57 Wu et al.57 isolated different layers of articular cartilage from femoral epiphysis of 5, 8, 12, and 17 weeks old human embryos by the laser capture dissection method and measured changes in the expression pattern of surface markers. Results of his study show that de novo chondrocyte condensation of MSCs is observable up to 11–12 weeks of gestation and fetal articular cartilage is not a homogenous tissue from week 12.57 Chondrocytes isolated from the superficial zone of the condensates were significantly different from the more differentiated and hypertrophic chondrocytes in the middle zone. Further, his results show that the vast majority of isolated chondrocytes from femoral epiphysis at week 17 of human development were zonally specified.57 Subsequently, during fetal and postnatal development and in puberty,58 the developing chondrocytes secrete specific marker proteins to remodel the zonal structure of articular cartilage (Fig. 7B).2,59 Results of Wu et al. also indicate that BMP-7 and IGF-1 play critical roles during early cartilage development.57
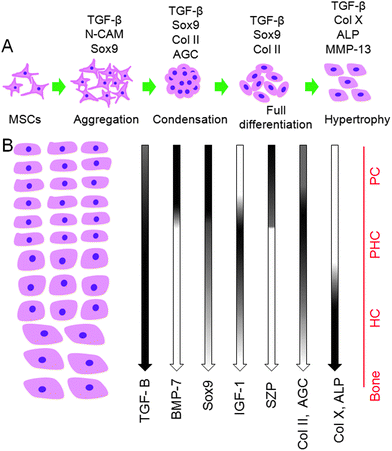 |
| Fig. 7 Schematic representation of the events during chondrogenesis. (A) Chondrogenesis consists of a dynamic series of events including stimulation of mesenchymal stem cells followed by mesenchymal aggregation and condensation and finally cartilage tissue differentiation and maturation. Expression patterns of specific cartilage genes at different stages of tissue development are shown. (B) Overview of the effect of specific growth factors on zonal organization of the articular cartilage tissue including superficial, middle/deep and calcified cartilage zones. The spatial distribution of specific growth factors in each zone is illustrated. The distribution of cartilage specific proteins is indicated by gradients of gene expression.2,59 PC, PHC, and HC stand for pre-chondrocytes, pre-hypertrophic chondrocytes, and hypertrophic chondrocytes, respectively. | |
In the superficial zone, BMP-7 in combination with TGF-β1 and low matrix stiffness up-regulate the expression of SZP in resident chondroprogenitor cell population.22,60 In the middle zone, IGF-1 as an anabolic growth factor in combination with TGF-β1 and a moderately stiff matrix have a direct effect on up-regulation of Col II and GAG in pre-hypertrophic chondrocytes.30 The calcified zone contains developmentally mature chondrocytes expressing proteins associated with hypertrophy such as Col X and ALP in a mineralized matrix.3,4 The combination of TGF-β1 signaling and high matrix stiffness is essential for the formation of calcified cartilage.5,18 We simulated the three zones of articular cartilage with hydrogels mimicking mechanical and biochemical signaling factors involved in articular cartilage tissue development and homeostasis. Specifically, stiffness and degradation of the constructs as biophysical cues, concentrations of three growth factors (TGF-β1, IGF-1 and BMP-7) as biochemical cues, and cell density as the cellular cue were used to control differentiation of the encapsulated human MSCs to the chondrocyte lineage corresponding to superficial, middle, and calcified zones. Results of previous clinical studies indicate that regeneration of the superficial zone occurs 3–4 weeks post-surgery41 while regeneration of load-bearing full-thickness cartilage happens after 8–12 weeks.41,42 Therefore, SPELA gels with resorption times of 4, 8 and 12 weeks were used to simulate the turnover rate of superficial, middle and calcified zones, respectively. Furthermore, compressive modulus of the gels was adjusted to 0.08, 2.1 and 320 MPa based on biomechanics of the superficial, middle and calcified zones in the native articular cartilage tissue, respectively.5
DNA content of the hMSCs in Superficial, Middle, and Calcified gels decreased slightly with incubation time which was attributed to cell differentiation leading to telomere shortening and a decrease in cell replication.61 The decrease in DNA content was consistent with the previously reported reduction in cell density with chondrogenic differentiation of MSCs in alginate gels with incubation time.62 mRNA results in Fig. 5 showed an increase in the expression of chondrogenic markers AGC and Col II of the hMSCs with incubation time for all three gels which was attributed to the exposure of encapsulated hMSCs to TGF-β. TGF-β stimulates chondrogenic differentiation of MSCs in a 3D culture environment4,63–65 in crosstalk with the Wnt/β-catenin pathway through Smad 2/3 phosphorylation. Next, phosphorylated Smad 2/3 forms a complex with Smad 4 and translocates to the nucleus to serve as a transcription factor for activation of Collagen II and AGC genes. In addition, Smad 3 and 4 interact with β-catenin66 and form a complex for protection of β-catenin from ubiquitin–proteasome dependent degradation and mediation of β-catenin nuclear translocation. Smad3-mediated stability of β-catenin activates down-steam chondrogenic target genes including Col II and AGC.66 TGF-β and Wnt/β-catenin pathways synergistically regulate expression of downstream chondrogenic target genes through transcription factors TCF/Lef1.67
hMSCs in the gel simulating the middle zone (Middle gel) had highest AGC and Col II expression with 112- and 45-fold increase on day 21 (Fig. 5A and B), respectively, which was consistent with the biochemical results in Fig. 6 and zonal composition of AGC and Col II in the native cartilage tissue. The Middle gel had significantly higher Col II and AGC expression relative to Superficial and Calcified gels which could be attributed to the combined effect of TGF-β1 and IGF-1 growth factors and the moderately stiff gel (2.1 MPa). IGF-1 has been shown to stimulate synthesis of proteoglycans and collagen in pre-hypertrophic chondrocytes of the middle zone in vivo and in vitro.7,30,68 In an in vivo study to investigate the effect of IGF-1 on prenatal and postnatal cartilage tissue development, cartilage specific IGF-1 knockout mice (Cart/igf-1−/−) died shortly after birth and showed disorganized chondrocyte columns, decreased cell proliferation, and increased apoptosis in their cartilage tissue.69 Further, exposure of 3D MSC cultures or chondrocyte grafts to IGF-1 significantly increased GAG and collagen synthesis,70 and IGF-1 stimulated synthesis of proteoglycans in pre-hypertrophic chondrocytes in vitro.30,71
Expression of SZP and Sox-9 was used as a marker for differentiation of hMSCs to chondrocyte lineage of the superficial zone. The Superficial gel had significantly higher expression of SZP relative to Superficial and Middle gels which could be attributed to the combined effect of TGF-β1 and BMP-7 growth factors and relatively low matrix compressive modulus (80 kPa).22,72 To engineer superficial zone chondrocytes, Coates co-cultured alginate-encapsulated MSCs with explants from the superficial or the middle/deep zone of bovine articular cartilage.73 Co-cultures with the superficial zone explants without the addition of TGF-β3 to the medium resulted in up-regulation of chondrogenic markers Sox-9 and Col II and a modest increase in SZP in the encapsulated MSCs after 21 days compared to the standard chondrogenic medium. Remarkably, the middle/deep zone explants did not induce expression of chondrogenic markers in co-cultured MSCs.73 The results of Coates et al. highlight the importance of growth factors secreted in the superficial zone on chondrogenesis whereas our results highlight the importance of BMP-7 in combination with TGF-β1 for directing differentiation of hMSCs to the superficial zone phenotype. Another factor in our experiments that may have contributed to the high SZP expression (21 fold increase) in Superficial gel was the relatively high cell density of hMSCs (60 × 106 cells per mL) consistent with that in the native cartilage tissue.74 GAG content of the superficial zone in native articular cartilage is lower than the remaining zones, consistent with our results (Fig. 6B).75 However contrary to our results (Fig. 6C), collagen content of the superficial zone is higher in the native tissue compared to the remaining zones75 and the orientation of collagen fibers is parallel to the cartilage surface.58 Julkunen et al. reported significant changes in the orientation of collagen fibers in femoral medial condyles of rabbits with postnatal development.58 Collagen content and orientation in the superficial zone are regulated by external load during postnatal skeletal maturation to limit deformation of the relatively compliant superficial zone in the direction parallel to the cartilage surface.76 Since the cell-seeded gels in our experiments were not loaded externally, we simulated compressive stiffness perpendicular to the cartilage surface as opposed to tensile modulus parallel to the surface. As a result, collagen content of the superficial zone was lower than the remaining zones. Steward et al.77 investigated the effect of matrix stiffness and hydrostatic pressure, with or without an integrin blocker, on matrix production and gene expression of MSCs encapsulated in agarose gels. They reported that stiffer gels suppressed cartilage matrix production in the chondrogenic medium but matrix synthesis returned to the levels in softer gels by blocking integrin receptor–ligand interaction with the RGDS peptide.77 It should be noted that the concentration of agarose in Steward et al. experiments ranged 1–4%77 which corresponded to moduli of 10–70 kPa.78 Conversely in our experiments, production of the chondrogenic matrix (total collagen and GAG) by the hMSCs was suppressed in the compliant Superficial gel compared to stiff Middle and Calcified gels. The discrepancy between our results and those of Steward et al.77 could be attributed to significantly higher modulus of the gels (80 kPa, 2.1 MPa, 320 MPa) in our experiments versus <70 kPa in Steward et al.77 and the absence of hydrostatic pressure in our experiments.
It is well established that TGF-β1 signaling regulates Sox-9 expression in all chondroprogenitor cells and developing chondrocytes but not in mature hypertrophic chondrocytes.2,79 Further, It has been shown that inactivation of BMP receptors in mice leads to loss of Sox-9 expression.80 Since both TGF-β1 and BMP-7 were used, the high expression of Sox-9 in the Superficial gel could be contributed to the TGF-β1/BMP-7 pathway through downstream Smad and TAK1 (TGF-β1 activated kinase) factors.80
The Calcified gel had significantly higher Col X and ALP expression relative to Middle and Superficial gels which could be attributed to the combined effect of high matrix modulus (320 MPa), TGF-β1, and HA. Khanarian et al. observed highest ALP activity in pre-hypertrophic chondrocytes derived from the deep zone of bovine articular cartilage and seeded in the agarose gel with the addition of 3% HA.81 Based on Huang et al. results, HA concentrations higher than 75 μg mL−1 induced expression of ALP in 3D cultures of rat MSCs at both mRNA and protein levels.82 In addition, mechanical strain along with HA addition had a positive effect on mRNA expression of ALP and matrix mineralization.82 Several studies have shown the significance of matrix stiffness on ALP expression. Chatterjee et al. detected no mineralization in MC3T3-E1 osteoblasts encapsulated in polyethylene glycol (PEG) gels with <100 kPa compressive modulus.101 The relatively low cell density of the Calcified gel could have also contributed to up-regulation of Col X and ALP expression of the hMSCs in the Calcified gel. Lower cell density can lead to a lower oxygen consumption rate and hence higher oxygen partial pressure in the gel, thus reducing the risk of hypoxia. Leijten et al. have shown that hypoxia suppresses markers of hypertrophy and endochondral ossification in fetal mouse tibia explants.84 Further, Eslaminejad and Nadri reported significantly higher mineralization by murine MSCs in 2D culture at lower cell seeding density.85 In fabrication of the Calcified gel, nanofiber microsheets were wrapped around a metallic rod sufficiently tight such that the suspended hMSCs came partially in contact with the surface of nanofibers. In other words, cells were encapsulated in the gel but could sense the fiber stiffness. Therefore, the fibrous nature of the Calcified gel could have also contributed to hypertrophy in encapsulated hMSCs as it has been reported that a 3D apatite-fiber scaffold structure enhanced endochondral ossification by bone marrow-derived stem cells.86
Previous studies revealed that substrate stiffness can direct lineage commitment of MSCs through a modulus-sensing mechanism mediated by integrin engagement and myosin-based contraction of actin cytoskeleton.87 Shih et al. observed up-regulation of α2 integrin and kinase activation of ROCK, FAK, and ERK1/2 of MSCs in stiff matrices.88 Further, matrix rigidity affects phosphorylation of multiple proteins such as Akt, β-catenin, Jnk, and STAT5a.89 Akt activation and increased β-catenin expression are implicated in the induction of the skeletal phenotype,90,91 whereas STAT5a has been shown to participate in up-regulation of 1,25-dihydroxyvitamin D3 which is critical to mineral deposition.92,93
Matrix stiffness plays an important role in regeneration of all cartilage zones. In a previous study, total collagen content of the chondrocytes encapsulated in PEG gels decreased with increasing compressive modulus from 30 to 950 kPa in the absence of chondrogenic growth factors whereas expression of Col II peaked in moderately crosslinked gels with compressive modulus of 360 kPa.94 The lower optimum compressive modulus for Col II expression in the previous study94 compared to that in the middle zone of the native articular cartilage could be attributed to suboptimal in vitro conditions with respect to biochemical signaling. Expression of TGF-β is regulated at multiple levels some of which intersect with mechanotransduction pathways.83 Previous studies indicate that TGF-β1 and optimum substrate stiffness have equivalent inductive effects on ATDC5 chondroprogenitor cells but the effect is synergistic when both are applied.83 Previous studies also indicate that integrin receptors and cell-generated tension mediate the conversion of TGF-β receptors from a latent to an active form.95 After receptor activation, TGF-β signaling propagates through multiple pathways including Rho/Rho-associated protein kinase (Rock) and mitogen-activated protein kinase (MAPK) pathways, and the canonical effectors of TGF-β signaling such as receptor-regulated Smad 2, 3 and 4, which leads to up-regulation of Sox-9 and Col II expression.96,97
Recently, Nguyen et al. used different combinations of PEG, chondroitin sulfate, and hyaluronic acid gels with compressive modulus of 100, 120 and 300 kPa, respectively, and equal TGF-β concentration and MSC density in all three layers to regenerate superficial, middle and deep zones of articular cartilage.98 Chondroitin sulfate and hyaluronic acid provided ECM signaling to the encapsulated MSCs. The calcified zone which has orders of magnitude higher modulus relative to the deep zone was not simulated in that study.98 Considering that only gradients in matrix stiffness and ECM signaling were used to guide differentiation of MSCs in Nguyen experiments, he reported highest expression of Col II and GAG by MSCs encapsulated in the gels simulating superficial and deep zones and a significant increase in Col X expression in all three gels. Our results with gradients in cell density, matrix stiffness, and growth factors (TGF-β, BMP-7, and IGF-1) showed highest expression of Col II and AGC in the Middle gel and highest Col X expression in the Calcified gel, consistent with the observed zonal expression of Col II, AGC, and Col X in the native articular cartilage tissue.3,5,18
Steele et al. generated bilayer scaffolds by electrostatic deposition of PCL microfibers onto porous scaffolds produced by porogen-leaching.21 After seeding the bilayer scaffolds with chondrocytes and culturing the constructs in the chondrogenic medium supplemented with 10 ng mL−1 TGF-β3, he observed higher sulfated glycosaminoglycan (sGAG) production in the zones with aligned fibers. However unlike our results, he observed uniform expression of SZP in all zones.21 McCullen et al. recently fabricated tri-laminar scaffolds composed of a layer of aligned fibers parallel to the scaffold surface laminated to two other randomly-oriented fiber layers to mimic microstructure and stiffness of the superficial, middle, and deep zones of articular cartilage, respectively.20 Col II mRNA expression of the chondrocytes seeded on the tri-laminar scaffolds increased with incubation time but there was no significant difference in Col II expression and GAG accumulation between the scaffold layers.
In this work, we focused on the effect of combined gradients in cell density, matrix stiffness, and zone-specific growth factors on differentiation and marker expression of hMSCs. Due to the multifactorial nature of the study, it was not possible to decouple the effect of individual factors (matrix stiffness, cell density, growth factors) on zone-specific differentiation and marker expression of the hMSCs. Inclusion of control groups for the effect of individual factors would have resulted in a very large number of samples. Nonetheless, there are very few studies on the combined effect of biochemical (growth factor), mechanical (matrix stiffness), and cellular (cell density) factors on differentiation of human MSCs to zone-specific chondrocytes. We recognize that zonal organization of articular cartilage is regulated by gradients of other equally important factors including tissue strain, oxygen tension, and glucose. Thorpe et al.99 confined MSC-encapsulated constructs radially to half their thickness and applied dynamic compression to create gradients in oxygen tension and strain in the direction of construct thickness. The gradients (low oxygen tension in the bottom and high strain at the top) increased GAG in the bottom, increased collagen accumulation at the top, and suppressed hypertrophy and calcification throughout the construct, which led to the formation of an engineered tissue mimicking some aspects of the zonal organization of articular cartilage. Spitters et al. cultivated bovine chondrocytes in a gradient of glucose concentrations and found that the GAG production rate was highest at the 5 mM glucose level.100 Recapitulating the gradients in all regulatory signals including matrix stiffness, cell density, growth factors, tissue strain, oxygen tension, and glucose concentration in a tissue engineered cell-seeded matrix has the potential to regenerate zonal complexity of the articular cartilage. In summary, our results demonstrate that the zonal structure of the native articular cartilage can be generated by adopting a developmental approach with gradients in cell density, matrix stiffness, and zone-specific growth factors.
Conclusions
We investigated the combined effect of cell density, matrix stiffness, and zone-specific growth factors within a 3D PEG-based hydrogel microenvironment on chondrogenic differentiation of encapsulated hMSCs into phenotypes corresponding to the superficial, middle, and calcified zones of articular cartilage. The superficial zone was simulated by encapsulating 60 × 106 cells per mL hMSCs in an 80 kPa SPELA gel loaded with 3 ng mL−1 TGF-β1 and 100 ng mL−1 BMP-7. The middle zone was simulated by encapsulating 20 × 106 cells per mL hMSCs in a 2.1 MPa SPELA gel loaded with 30 ng mL−1 TGF-β1 and 100 ng mL−1 IGF-1. The calcified zone was simulated by encapsulating 15 × 106 cells per mL hMSCs in a 320 MPa gel reinforced with nanofibers aligned perpendicular to the gel surface and loaded with 30 ng mL−1 TGF-β1 and 3% HA. hMSCs in the simulated superficial zone gel showed up-regulation of Sox-9, the early-stage marker of chondrogenesis, and SZP whereas hMSCs in the simulated calcified zone gel showed up-regulation of Col X, the late-stage marker of hypertrophic chondrocytes, and ALP. Further, hMSCs in the simulated middle zone gel had highest expression of chondrogenic markers Col II, AGC, and GAG among all three gels. The results of this work can potentially lead to the design of more effective multilayer grafts with mechanical, biochemical, and cellular gradients for the treatment of articular cartilage defects.
Acknowledgements
This work was supported by research grants to E. Jabbari from the National Science Foundation under grant No. DMR1049381, IIP-1357109, and CBET1403545, the National Institutes of Health under grant No. AR063745, the Arbeitsgemeinschaft Fur Osteosynthesefragen (AO) Foundation under grant No. C10-44J, and the University of South Carolina office of VP for Research under grant no. 15510-14-36182. The authors thank Samaneh K. Sarvestani and William C. Welsh for assistance with cell cultivation and analysis.
References
- S. P. Grogan, S. F. Duffy, C. Pauli, J. A. Koziol, A. I. Su, D. D. D'Lima and M. K. Lotz, Zone-specific gene expression patterns in articular cartilage, Arthritis Rheum., 2013, 65, 418–428 CrossRef CAS PubMed.
- F. Las Heras, H. K. Gahunia and K. P. Pritzker, Articular cartilage development: A molecular perspective, Orthop. Clin. North Am., 2012, 43, 155–171 CrossRef PubMed.
- T. J. Klein, J. Malda, R. L. Sah and D. W. Hutmacher, Tissue engineering of articular cartilage with biomimetic zones, Tissue Eng., Part B, 2009, 15, 143–157 CrossRef CAS PubMed.
- L. Kock, C. C. van Donkelaar and K. Ito, Tissue engineering of functional articular cartilage: The current status, Cell Tissue Res., 2012, 347, 613–627 CrossRef CAS PubMed.
- P. J. Yang and J. S. Temenoff, Engineering orthopedic tissue interfaces, Tissue Eng., Part B, 2009, 15, 127–141 CrossRef CAS PubMed.
- P. H. Chao, S. Yodmuang, X. Wang, L. Sun, D. L. Kaplan and G. Vunjak-Novakovic, Silk hydrogel for cartilage tissue engineering, J. Biomed. Mater. Res., Part B, 2010, 95, 84–90 CrossRef PubMed.
- K. L. Spiller, S. A. Maher and A. M. Lowman, Hydrogels for the repair of articular cartilage defects, Tissue Eng., Part B, 2011, 17, 281–299 CrossRef CAS PubMed.
- C. S. Bahney, C. W. Hsu, J. U. Yoo, J. L. West and B. Johnstone, A bioresponsive hydrogel tuned to chondrogenesis of human mesenchymal stem cells, FASEB J., 2011, 25, 1486–1496 CrossRef CAS PubMed.
- M. Pacifici, E. Koyama, Y. Shibukawa, C. Wu, Y. Tamamura, M. Enomoto-Iwamoto and M. Iwamoto, Cellular and molecular mechanisms of synovial joint and articular cartilage formation, Ann. N. Y. Acad. Sci., 2006, 1068, 74–86 CrossRef CAS PubMed.
- K. W. Ng, G. A. Ateshian and C. T. Hung, Zonal chondrocytes seeded in a layered agarose hydrogel create engineered cartilage with depth-dependent cellular and mechanical inhomogeneity, Tissue Eng., Part A, 2009, 15, 2315–2324 CrossRef CAS PubMed.
- S. Moon, S. K. Hasan, Y. S. Song, F. Xu, H. O. Keles, F. Manzur, S. Mikkilineni, J. W. Hong, J. Nagatomi, E. Haeggstrom, A. Khademhosseini and U. Demirci, Layer by layer three-dimensional tissue epitaxy by cell-laden hydrogel droplets, Tissue Eng., Part C, 2010, 16, 157–166 CrossRef CAS PubMed.
- B. Sharma, C. G. Williams, T. K. Kim, D. Sun, A. Malik, M. Khan, K. Leong and J. H. Elisseeff, Designing zonal organization into tissue-engineered cartilage, Tissue Eng., 2007, 13, 405–414 CrossRef CAS PubMed.
- X. Cui, K. Breitenkamp, M. G. Finn, M. Lotz and D. D. D'Lima, Direct human cartilage repair using three-dimensional bioprinting technology, Tissue Eng., Part A, 2012, 18, 1304–1312 CrossRef CAS PubMed.
- E. M. Darling and K. A. Athanasiou, Rapid phenotypic changes in passaged articular chondrocyte subpopulations, J. Orthop. Res., 2005, 23, 425–432 CrossRef CAS PubMed.
- U. R. Goessler, K. Bieback, P. Bugert, R. Naim, C. Schafer, H. Sadick, K. Hormann and F. Riedel, Human chondrocytes differentially express matrix modulators during in vitro expansion for tissue engineering, Int. J. Mol. Med., 2005, 16, 509–515 CAS.
- U. R. Goessler, P. Bugert, K. Bieback, A. Baisch, H. Sadick, T. Verse, H. Kluter, K. Hormann and F. Riedel, Expression of collagen and fiber-associated proteins in human septal cartilage during in vitro dedifferentiation, Int. J. Mol. Med., 2004, 14, 1015–1022 CAS.
- S. Boeuf and W. Richter, Chondrogenesis of mesenchymal stem cells: Role of tissue source and inducing factors, Stem Cell Res. Ther., 2010, 1, 31 CrossRef PubMed.
- L. H. Nguyen, A. K. Kudva, N. L. Guckert, K. D. Linse and K. Roy, Unique biomaterial compositions direct bone marrow stem cells into specific chondrocytic phenotypes corresponding to the various zones of articular cartilage, Biomaterials, 2011, 32, 1327–1338 CrossRef CAS PubMed.
- J. A. Hendriks, L. Moroni, J. Riesle, J. R. de Wijn and C. A. van Blitterswijk, The effect of scaffold-cell entrapment capacity and physico-chemical properties on cartilage regeneration, Biomaterials, 2013, 34, 4259–4265 CrossRef CAS PubMed.
- S. D. McCullen, H. Autefage, A. Callanan, E. Gentleman and M. M. Stevens, Anisotropic fibrous scaffolds for articular cartilage regeneration, Tissue Eng., Part A, 2012, 18, 2073–2083 CrossRef CAS PubMed.
- J. A. Steele, S. D. McCullen, A. Callanan, H. Autefage, M. A. Accardi, D. Dini and M. M. Stevens, Combinatorial scaffold morphologies for zonal articular cartilage engineering, Acta Biomater., 2014, 10, 2065–2075 CrossRef CAS PubMed.
- J. A. Andrades, S. C. Motaung, P. Jimenez-Palomo, S. Claros, J. M. Lopez-Puerta, J. Becerra, T. M. Schmid and A. H. Reddi, Induction of superficial zone protein (szp)/lubricin/prg 4 in muscle-derived mesenchymal stem/progenitor cells by transforming growth factor-beta1 and bone morphogenetic protein-7, Arthritis Res. Ther., 2012, 14, R72 CAS.
- A. D. Pearle, R. F. Warren and S. A. Rodeo, Basic science of articular cartilage and osteoarthritis, Clin. J. Sport Med., 2005, 24, 1–12 CrossRef PubMed.
- R. M. Schinagl, D. Gurskis, A. C. Chen and R. L. Sah, Depth-dependent confined compression modulus of full-thickness bovine articular cartilage, J. Orthop. Res., 1997, 15, 499–506 CrossRef CAS PubMed.
- R. A. Stockwel, Interrelationship of cell density and cartilage thickness in mammalian articular cartilage, J. Anat., 1971, 109, 411–421 Search PubMed.
- V. Lefebvre and P. Bhattaram, Vertebrate skeletogenesis, Curr. Top. Dev. Biol., 2010, 90, 291–317 Search PubMed.
- E. M. Darling and K. A. Athanasiou, Growth factor impact on articular cartilage subpopulations, Cell Tissue Res., 2005, 322, 463–473 CrossRef CAS PubMed.
- S. V. Eleswarapu, N. D. Leipzig and K. A. Athanasiou, Gene expression of single articular chondrocytes, Cell Tissue Res., 2007, 327, 43–54 CrossRef CAS PubMed.
- A. Khalafi, T. M. Schmid, C. Neu and A. H. Reddi, Increased accumulation of superficial zone protein (szp) in articular cartilage in response to bone morphogenetic protein-7 and growth factors, J. Orthop. Res., 2007, 25, 293–303 CrossRef CAS PubMed.
- E. Coates and J. P. Fisher, Gene expression of alginate-embedded chondrocyte subpopulations and their response to exogenous igf-1 delivery, J. Tissue Eng. Regener. Med., 2012, 6, 179–192 CrossRef CAS PubMed.
- Z. Lin, C. Willers, J. A. Xu and M. H. Zheng, The chondrocyte: Biology and clinical application, Tissue Eng., 2006, 12, 1971–1984 CrossRef CAS PubMed.
- D. G. Hickey, S. R. Frenkel and P. E. Di Cesare, Clinical applications of growth factors for articular cartilage repair, Am. J. Orthop., 2003, 32, 70–76 Search PubMed.
- T. Sueyoshi, K. Yamamoto and H. Akiyama, Conditional deletion of tgfbr2 in hypertrophic chondrocytes delays terminal chondrocyte differentiation, Matrix Biol., 2012, 31, 352–359 CrossRef CAS PubMed.
- T. Niikura and A. H. Reddi, Differential regulation of lubricin/superficial zone protein by transforming growth factor beta/bone morphogenetic protein superfamily members in articular chondrocytes and synoviocytes, Arthritis Rheum., 2007, 56, 2312–2321 CrossRef CAS PubMed.
- H. J. Pulkkinen, V. Tiitu, P. Valonen, J. S. Jurvelin, M. J. Lammi and I. Kiviranta, Engineering of cartilage in recombinant human type II collagen gel in nude mouse model in vivo, Osteoarthritis Cartilage, 2010, 18, 1077–1087 CrossRef CAS PubMed.
- J. Kundu, J. H. Shim, J. Jang, S. W. Kim and D. W. Cho, An additive manufacturing-based PCL–alginate–chondrocyte bioprinted scaffold for cartilage tissue engineering, J. Tissue Eng. Regener. Med., 2013 DOI:10.1002/term.1682.
- H. Park, B. Choi, J. Hu and M. Lee, Injectable chitosan hyaluronic acid hydrogels for cartilage tissue engineering, Acta Biomater., 2013, 9, 4779–4786 CrossRef CAS PubMed.
- S. Moeinzadeh, D. Barati, X. He and E. Jabbari, Gelation characteristics and osteogenic differentiation of stromal cells in inert hydrolytically degradable micellar polyethylene glycol hydrogels, Biomacromolecules, 2012, 13, 2073–2086 CrossRef CAS PubMed.
- C. Cha, W. B. Liechty, A. Khademhosseini and N. A. Peppas, Designing biomaterials to direct stem cell fate, ACS Nano, 2012, 6, 9353–9358 CrossRef CAS PubMed.
- S. Moeinzadeh, D. Barati, S. K. Sarvestani, O. Karaman and E. Jabbari, Nanostructure formation and transition from surface to bulk degradation in polyethylene glycol gels chain-extended with short hydroxy acid segments, Biomacromolecules, 2013, 14, 2917–2928 CrossRef CAS PubMed.
- C. H. Lee, J. L. Cook, A. Mendelson, E. K. Moioli, H. Yao and J. J. Mao, Regeneration of the articular surface of the rabbit synovial joint by cell homing: A proof of concept study, Lancet, 2010, 376, 440–448 CrossRef CAS.
- M. Falah, G. Nierenberg, M. Soudry, M. Hayden and G. Volpin, Treatment of articular cartilage lesions of the knee, Int. Orthop., 2010, 34, 621–630 CrossRef PubMed.
- S. Moeinzadeh, S. N. Khorasani, J. Ma, X. He and E. Jabbari, Synthesis and gelation characteristics of photo-crosslinkable star poly(ethylene oxide-co-lactide-glycolide acrylate) macromonomers, Polymer, 2011, 52, 3887–3896 CrossRef CAS PubMed.
- X. He, J. Ma and E. Jabbari, Effect of grafting rgd and bmp-2 protein-derived peptides to a hydrogel substrate on osteogenic differentiation of marrow stromal cells, Langmuir, 2008, 24, 12508–12516 CrossRef CAS PubMed.
- O. Karaman, A. Kumar, S. Moeinzadeh, X. He, T. Cui and E. Jabbari, Effect of surface modification of nanofibers with glutamic acid peptide on calcium phosphate nucleation and osteogenic differentiation of marrow stromal cells, J. Tissue Eng. Regener. Med., 2013 DOI:10.1002/term.1775.
- W. Xu, J. Ma and E. Jabbari, Material properties and osteogenic differentiation of marrow stromal cells on fiber-reinforced laminated hydrogel nanocomposites, Acta Biomater., 2010, 6, 1992–2002 CrossRef CAS PubMed.
- X. Yang, S. K. Sarvestani, S. Moeinzadeh, X. He and E. Jabbari, Three-dimensional-engineered matrix to study cancer stem cells and tumorsphere formation: Effect of matrix modulus, Tissue Eng., Part A, 2013, 19, 669–684 CrossRef CAS PubMed.
- A. S. Sarvestani and E. Jabbari, Modeling and experimental investigation of rheological properties of injectable poly(lactide ethylene oxide fumarate)/hydroxyapatite nanocomposites, Biomacromolecules, 2006, 7, 1573–1580 CrossRef CAS PubMed.
- S. Kern, H. Eichler, J. Stoeve, H. Kluter and K. Bieback, Comparative analysis of mesenchymal stem cells from bone marrow, umbilical cord blood, or adipose tissue, Stem Cells, 2006, 24, 1294–1301 CrossRef CAS PubMed.
- X. Zhang, M. Hirai, S. Cantero, R. Ciubotariu, L. Dobrila, A. Hirsh, K. Igura, H. Satoh, I. Yokomi, T. Nishimura, S. Yamaguchi, K. Yoshimura, P. Rubinstein and T. A. Takahashi, Isolation and characterization of mesenchymal stem cells from human umbilical cord blood: Reevaluation of critical factors for successful isolation and high ability to proliferate and differentiate to chondrocytes as compared to mesenchymal stem cells from bone marrow and adipose tissue, J. Cell. Biochem., 2011, 112, 1206–1218 CrossRef CAS PubMed.
- C. R. Correia, L. S. Moreira-Teixeira, L. Moroni, R. L. Reis, C. A. van Blitterswijk, M. Karperien and J.
F. Mano, Chitosan scaffolds containing hyaluronic acid for cartilage tissue engineering, Tissue Eng., Part C, 2011, 17, 717–730 CrossRef CAS PubMed.
- J. L. Ruan, N. L. Tulloch, V. Muskheli, E. E. Genova, P. D. Mariner, K. S. Anseth and C. E. Murry, An improved cryosection method for polyethylene glycol hydrogels used in tissue engineering, Tissue Eng., Part C, 2013, 19, 794–801 CrossRef CAS PubMed.
- L. Gao, T. J. Sheu, Y. Dong, D. M. Hoak, M. J. Zuscik, E. M. Schwarz, M. J. Hilton, R. J. O'Keefe and J. H. Jonason, Tak1 regulates sox9 expression in chondrocytes and is essential for postnatal development of the growth plate and articular cartilages, J. Cell Sci., 2013, 126, 5704–5713 CrossRef CAS PubMed.
- J. A. Henderson, X. He and E. Jabbari, Concurrent differentiation of marrow stromal cells to osteogenic and vasculogenic lineages, Macromol. Biosci., 2008, 8, 499–507 CrossRef CAS PubMed.
- L. G. Cima and S. T. Lopina, Network structures of radiation-cross-linked star polymer gels, Macromolecules, 1995, 28, 6787–6794 CrossRef CAS.
- J. Coburn, M. Gibson, P. A. Bandalini, C. Laird, H. Q. Mao, L. Moroni, D. Seliktar and J. Elisseeff, Biomimetics of the extracellular matrix: An integrated three-dimensional fiber-hydrogel composite for cartilage tissue engineering, Smart Struct. Syst., 2011, 7, 213–222 CrossRef PubMed.
- L. Wu, C. Bluguermann, L. Kyupelyan, B. Latour, S. Gonzalez, S. Shah, Z. Galic, S. Ge, Y. Zhu, F. A. Petrigliano, A. Nsair, S. G. Miriuka, X. Li, K. M. Lyons, G. M. Crooks, D. R. McAllister, B. Van Handel, J. S. Adams and D. Evseenko, Human developmental chondrogenesis as a basis for engineering chondrocytes from pluripotent stem cells, Stem Cell Rev. Rep., 2013, 1, 575–589 CrossRef CAS PubMed.
- P. Julkunen, J. Iivarinen, P. A. Brama, J. Arokoski, J. S. Jurvelin and H. J. Helminen, Maturation of collagen fibril network structure in tibial and femoral cartilage of rabbits, Osteoarthritis Cartilage, 2010, 18, 406–415 CrossRef CAS PubMed.
- I. Gadjanski, K. Spiller and G. Vunjak-Novakovic, Time-dependent processes in stem cell-based tissue engineering of articular cartilage, Stem Cell Rev., 2012, 8, 863–881 CrossRef CAS PubMed.
- T. Iwakura, R. Sakata and A. H. Reddi, Induction of chondrogenesis and expression of superficial zone protein in synovial explants with tgf-beta 1 and bmp-7, Tissue Eng., Part A, 2013, 19, 2638–2644 CrossRef CAS PubMed.
- H. Lans, J. M. Lindvall, K. Thijssen, A. E. Karambelas, D. Cupac, O. Fensgard, G. Jansen, J. H. Hoeijmakers, H. Nilsen and W. Vermeulen, DNA damage leads to progressive replicative decline but extends the life span of long-lived mutant animals, Cell Death Differ., 2013, 20, 1709–1718 CrossRef CAS PubMed.
- V. Dexheimer, S. Frank and W. Richter, Proliferation as a requirement for in vitro chondrogenesis of human mesenchymal stem cells, Stem Cells Dev., 2012, 21, 2160–2169 CrossRef CAS PubMed.
- M. L. Alves da Silva, A. Martins, A. R. Costa-Pinto, P. Costa, S. Faria, M. Gomes, R. L. Reis and N. M. Neves, Cartilage tissue engineering using electrospun PCL nanofiber meshes and MSCs, Biomacromolecules, 2010, 11, 3228–3236 CrossRef CAS PubMed.
- A. N. Buxton, C. S. Bahney, J. U. Yoo and B. Johnstone, Temporal exposure to chondrogenic factors modulates human mesenchymal stem cell chondrogenesis in hydrogels, Tissue Eng., Part A, 2011, 17, 371–380 CrossRef CAS PubMed.
- R. L. Mauck, X. Yuan and R. S. Tuan, Chondrogenic differentiation and functional maturation of bovine mesenchymal stem cells in long-term agarose culture, Osteoarthritis Cartilage, 2006, 14, 179–189 CrossRef CAS PubMed.
- M. Zhang, M. Wang, X. Tan, T. F. Li, Y. E. Zhang and D. Chen, Smad3 prevents beta-catenin degradation and facilitates beta-catenin nuclear translocation in chondrocytes, J. Biol. Chem., 2010, 285, 8703–8710 CrossRef CAS PubMed.
- E. Labbe, A. Letamendia and L. Attisano, Association of smads with lymphoid enhancer binding factor 1/t cell-specific factor mediates cooperative signaling by the transforming growth factor-beta and wnt pathways, Proc. Natl. Acad. Sci. U. S. A., 2000, 97, 8358–8363 CrossRef CAS PubMed.
- F. Z. Lu, Z. Y. Jiang, X. X. Wang, Y. H. Luo, X. F. Li and H. L. Liu, Role of the insulin-like growth factor system in epiphyseal cartilage on the development of langshan and arbor acres chickens, gallus domesticus, Poult. Sci., 2010, 89, 956–965 CrossRef CAS PubMed.
- Y. Wang, Z. Cheng, H. Z. Elalieh, E. Nakamura, M. T. Nguyen, S. Mackem, T. L. Clemens, D. D. Bikle and W. Chang, IGF-1r signaling in chondrocytes modulates growth plate development by interacting with the pthrp/ihh pathway, J. Bone Miner. Res., 2011, 26, 1437–1446 CrossRef CAS PubMed.
- A. A. Worster, B. D. Brower-Toland, L. A. Fortier, S. J. Bent, J. Williams and A. J. Nixon, Chondrocytic differentiation of mesenchymal stem cells sequentially exposed to transforming growth factor-beta1 in monolayer and insulin-like growth factor-I in a three-dimensional matrix, J. Orthop. Res., 2001, 19, 738–749 CrossRef CAS PubMed.
- L. Longobardi, L. O'Rear, S. Aakula, B. Johnstone, K. Shimer, A. Chytil, W. A. Horton, H. L. Moses and A. Spagnoli, Effect of igf-I in the chondrogenesis of bone marrow mesenchymal stem cells in the presence or absence of tgf-beta signaling, J. Bone Miner. Res., 2006, 21, 626–636 CrossRef CAS PubMed.
- S. Y. Lee, T. Nakagawa and A. H. Reddi, Induction of chondrogenesis and expression of superficial zone protein (szp)/lubricin by mesenchymal progenitors in the infrapatellar fat pad of the knee joint treated with tgf-beta1 and bmp-7, Biochem. Biophys. Res. Commun., 2008, 376, 148–153 CrossRef CAS PubMed.
- E. E. Coates and J. P. Fisher, Engineering superficial zone chondrocytes from mesenchymal stem cells, Tissue Eng., Part C, 2014, 20, 630–640 CrossRef CAS PubMed.
- T. M. Quinn, E. B. Hunziker and H. J. Hauselmann, Variation of cell and matrix morphologies in articular cartilage among locations in the adult human knee, Osteoarthritis Cartilage, 2005, 13, 672–678 CrossRef PubMed.
- A. R. Gannon, T. Nagel and D. J. Kelly, The role of the superficial region in determining the dynamic properties of articular cartilage, Osteoarthritis Cartilage, 2012, 20, 1417–1425 CrossRef CAS PubMed.
- R. K. Korhonen, M. Wong, J. Arokoski, R. Lindgren, H. J. Helminen, E. B. Hunziker and J. S. Jurvelin, Importance of the superficial tissue layer for the indentation stiffness of articular cartilage, Med. Eng. Phys., 2002, 24, 99–108 CrossRef CAS PubMed.
- A. J. Steward, D. R. Wagner and D. J. Kelly, The pericellular environment regulates cytoskeletal development and the differentiation of mesenchymal stem cells and determines their response to hydrostatic pressure, Eur. Cells Mater., 2013, 25, 167–178 CAS.
- Q. S. Chen, B. Suki and K. N. An, Dynamic mechanical properties of agarose gels modeled by a fractional derivative model, J. Biomech. Eng., 2004, 126, 666–671 CrossRef PubMed.
- H. Akiyama and V. Lefebvre, Unraveling the transcriptional regulatory machinery in chondrogenesis, J. Bone Miner. Metab., 2011, 29, 390–395 CrossRef PubMed.
- B. S. Yoon, D. A. Ovchinnikov, I. Yoshii, Y. Mishina, R. R. Behringer and K. M. Lyons, Bmpr1a and bmpr1b have overlapping functions and are essential for chondrogenesis in vivo, Proc. Natl. Acad. Sci. U. S. A., 2005, 102, 5062–5067 CrossRef CAS PubMed.
- N. T. Khanarian, N. M. Haney, R. A. Burga and H. H. Lu, A functional agarose-hydroxyapatite scaffold for osteochondral interface regeneration, Biomaterials, 2012, 33, 5247–5258 CrossRef CAS PubMed.
- Y. Huang, X. Niu, W. Song, C. Guan, Q. Feng and Y. Fao, Combined effect of mechanical strain and hydroxyapatite/collagen composite on osteogenic differentiation of rat bone marrow derived mesenchymal stem cells, J. Nanomater., 2013, 2013, 343909 Search PubMed.
- J. L. Allen, M. E. Cooke and T. Alliston, Ecm stiffness primes the tgfbeta pathway to promote chondrocyte differentiation, Mol. Biol. Cell, 2012, 23, 3731–3742 CrossRef CAS PubMed.
- J. C. H. Leijten, L. S. M. Teixeira, E. B. M. Landman, C. A. van Blitterswijk and M. Karperien, Hypoxia inhibits hypertrophic differentiation and endochondral ossification in explanted tibiae, PLoS One, 2012, 7, e49896, DOI:10.1371/journal.pone.0049896.
- M. B. Eslaminejad and S. Nadri, Murine mesenchymal stem cell isolated and expanded in low and high density culture system: Surface antigen expression and osteogenic culture mineralization, In Vitro Cell. Dev. Biol.: Anim., 2009, 45, 451–459 CrossRef CAS PubMed.
- M. Honda, Y. Watanabe, T. Tsuchiya, N. Kanzawa and M. Aizawa, Selective differentiation of bone marrow-derived mesenchymal stromal cells into osteocytes via endochondral ossification in an apatite-fiber scaffold, J. Ceram. Soc. Jpn., 2013, 121, 759–765 CrossRef CAS.
- A. J. Engler, S. Sen, H. L. Sweeney and D. E. Discher, Matrix elasticity directs stem cell lineage specification, Cell, 2006, 126, 677–689 CrossRef CAS PubMed.
- Y. R. Shih, K. F. Tseng, H. Y. Lai, C. H. Lin and O. K. Lee, Matrix stiffness regulation of integrin-mediated mechanotransduction during osteogenic differentiation of human mesenchymal stem cells, J. Bone Miner. Res., 2011, 26, 730–738 CrossRef CAS PubMed.
- S. Liu, D. A. Calderwood and M. H. Ginsberg, Integrin cytoplasmic domain-binding proteins, J. Cell Sci., 2000, 113, 3563–3571 CAS.
- A. Mukherjee and P. Rotwein, Akt promotes bmp2-mediated osteoblast differentiation and bone development, J. Cell Sci., 2009, 122, 716–726 CrossRef CAS PubMed.
- G. Mbalaviele, S. Sheikh, J. P. Stains, V. S. Salazar, S. L. Cheng, D. Chen and R. Civitelli, Beta-catenin and bmp-2 synergize to promote osteoblast differentiation and new bone formation, J. Cell. Biochem., 2005, 94, 403–418 CrossRef CAS PubMed.
- C. Chenu, A. Valentin-Opran, P. Chavassieux, S. Saez, P. J. Meunier and P. D. Delmas, Insulin like growth factor I hormonal regulation by growth hormone and by 1, 25(oh)2d3 and activity on human osteoblast-like cells in short-term cultures, Bone, 1990, 11, 81–86 CrossRef CAS PubMed.
- G. Morel, P. Chavassieux, B. Barenton, P. M. Dubois, P. J. Meunier and G. Boivin, Evidence for a direct effect of growth hormone on osteoblasts, Cell Tissue Res., 1993, 273, 279–286 CrossRef CAS PubMed.
- S. J. Bryant and K. S. Anseth, Hydrogel properties influence ECM production by chondrocytes photoencapsulated in poly(ethylene glycol) hydrogels, J. Biomed. Mater. Res., 2002, 59, 63–72 CrossRef CAS PubMed.
- P. J. Wipff, D. B. Rifkin, J. J. Meister and B. Hinz, Myofibroblast contraction activates latent tgf-beta1 from the extracellular matrix, J. Cell Biol., 2007, 179, 1311–1323 CrossRef CAS PubMed.
- J. L. Wrana, Regulation of smad activity, Cell, 2000, 100, 189–192 CrossRef CAS PubMed.
- T. Furumatsu, M. Tsuda, N. Taniguchi, Y. Tajima and H. Asahara, Smad3 induces chondrogenesis through the activation of sox9 via creb-binding protein/p300 recruitment, J. Biol. Chem., 2005, 280, 8343–8350 CrossRef CAS PubMed.
- L. H. Nguyen, A. K. Kudva, N. S. Saxena and K. Roy, Engineering articular cartilage with spatially-varying matrix composition and mechanical properties from a single stem cell population using a multi-layered hydrogel, Biomaterials, 2011, 32, 6946–6952 CrossRef CAS PubMed.
- S. D. Thorpe, T. Nagel, S. F. Carroll and D. J. Kelly, Modulating gradients in regulatory signals within mesenchymal stem cell seeded hydrogels: A novel strategy to engineer zonal articular cartilage, PLoS One, 2013, 8, e60764, DOI:10.1371/journal.pone.0060764.
- T. W. Spitters, C. M. Mota, S. C. Uzoechi, B. Slowinska, D. E. Martens, L. Moroni and M. Karperien, Glucose gradients influence zonal matrix deposition in 3D cartilage constructs, Tissue Eng., Part A, 2014 DOI:10.1089/ten.tea.2014.0059.
- K. Chatterjee, S. Lin-Gibson, W. E. Wallace, S. H. Parekh, Y. J. Lee, M. T. Cicerone, M. F. Young and C. G. Simon, Jr., The effect of 3D hydrogel scaffold modulus on osteoblast differentiation and mineralization revealed by combinatorial screening, Biomaterials, 2010, 31, 5051–5062 CrossRef CAS PubMed.
|
This journal is © The Royal Society of Chemistry 2015 |