The pore surface diffusion model as a tool for rapid screening of novel nanomaterial-enhanced hybrid ion-exchange media†
Received
23rd April 2015
, Accepted 17th June 2015
First published on 18th June 2015
Abstract
The primary goal of this study was to examine the feasibility of using Pore Surface Diffusion Model (PSDM) as a rapid screening tool to predict breakthrough curves of short bed columns packed with nanomaterial enhanced hybrid-ion exchange media. A novel hybrid-ion exchange media was fabricated by synthesizing titanium dioxide nanostructured endoskeleton inside nitrate selective strong base ion-exchange resin. The properties of the hybrid media were characterized and batch reactor tests were conducted with arsenate (As) and nitrogen-nitrate (NO3−N) as model contaminants in 5 mM NaHCO3 buffer ultrapure water at final pH = 7.2 ± 0.3 to assess the contaminant removal capacity and develop sorption isotherms. Freudlich sorption isotherm (q = KCe1/n) model was used to provide inputs for the PSDM predictions of the contaminants' breakthrough curves. To validate the PSDM breakthrough curve predictions for both model contaminants, continuous short bed packed column tests were conducted under the modeled conditions. Hybrid media regeneration was also conducted with solution mix of 2% KOH and 1% KCl followed by rinse with 0.1% HCl, and the column was operated under same conditions to assess the regeneration efficiency of the media. The results demonstrated that 100% regeneration efficiency could be achieved for nitrate, while only 75% efficiency could be achieved for arsenate under these regeneration conditions. The PSDM was able to successfully predict the breakthrough curves for arsenic and nitrate with relatively high precision, characterized by R2 ≈ 0.977 and R2 ≈ 0.930 for arsenate and nitrate, respectively.
Water impact
Nanotechnology allows for hybridization of existing ion-exchange media and development of small point-of-use or point-of-entry packed-bed water treatment systems that have the ability to simultaneously remove multiple contaminants from groundwater with different matrices, and consequently eliminate the need for complex multi-unit treatment trains. Fabrication of nanostructured metal-oxide endoskeletons in the ion-exchange media allows the use of the pore surface diffusion model (PSDM) to be used as a rapid screening tool in engineering new nano-hybridized ion-exchange media for simultaneous removal of contaminants with different chemistries from water. As such, the PSDM model could be employed to rapidly screen potentially promising new hybrid media by also considering other factors than adsorption capacity alone.
|
Introduction
Many small, rural, and poor communities around the world, which rely on contaminated groundwater as their only available source, lack technical and financial capacities to implement, operate, and manage expensive and technologically advanced water treatment systems.1 However, with the ever-growing demand for potable water, there is potential to employ sorption-based (e.g. adsorption, ion-exchange) water treatment technologies that could offer a simple and inexpensive alternative capable of simultaneously removing multiple contaminants.2 The advent of nanotechnology allows for hybridization of such sorbents and development of small point-of-use or point-of-entry systems that have the ability to eliminate multi-unit treatment trains, which are often necessary for treatment of complex groundwater matrices containing multiple contaminants with different chemistries.3
Development and testing of these nanomaterial-enhanced hybrid sorbents frequently represent an expensive process because of substantial time requirements and high cost of nanomaterial synthesis, testing, and optimization both in laboratory and real-world environments.4–6 For example, pilot-scale testing of novel sorbent media frequently requires several months to complete, and could cost several hundred thousand dollars.7 Laboratory scale short packed bed column tests, which are significantly cheaper and offer significant advantage over the pilot-scale testing, often require several days or weeks to complete, and consequently limit their suitability as platforms for rapid performance screening of nanomaterial-enhanced hybrid sorbents.8 In contrast, batch reactor testing coupled with isotherm modeling does not provide sufficient information about performance of the media in continuous flow configurations although it allows for rapid screening and determination of sorption capacity. Several studies have demonstrated that the pore surface diffusion model (PSDM) is capable of predicting performance of continuous flow fixed bed systems packed with nanomaterial impregnated granular activated carbon or nanostructured metal (hydr)oxide media.9–12 This discernment created an opportunity to utilize the PSDM as a tool for rapid screening of novel hybrid sorbents. However, concerns may exist that the model is only suitable for predicting performance of fixed bed configurations that are packed with media characterized by constant volume, and as such, the model would not be suitable to predict the breakthrough of hybrid ion-exchange (HIX) based systems because of the ability of these ion-exchange resins to change their volume when hydrated.13
Development of nanomaterial-enhanced hybrid ion-exchange media, which incorporates an endoskeletal structure comprised of nanomaterials, could have the ability to minimize any change in ion-exchange resin volume due to hydration. As such, it could consequently serve as a platform to validate that the pore surface diffusion model could be used as a rapid screening tool when developing similar hybrid ion-exchange media. A recent study demonstrated that nanostructured endoskeleton of titanium dioxide could be fabricated inside strong base ion-exchange resin.14 This nanomaterial-enhanced HIX media could serve as a model to examine the underlying hypothesis that the PSDM is capable of providing satisfactory breakthrough curve predictions (R2 > 0.9) for contaminants with different chemistries, which are targeted for removal by the fabricated HIX. To test this hypothesis, a set of four task-oriented objectives were achieved: (1) Titanium dioxide nanomaterial-enhanced hybrid ion-exchange media was fabricated and characterized; (2) Batch reactor tests were conducted for two model contaminants (arsenate and nitrate) and isotherms were developed using the Freundlich isotherm model; (3) Continuous flow short packed bed column tests were performed under realistic hydraulic loading rates to experimentally obtain breakthrough curves for the two model contaminants; and (4) Pore Surface Diffusion Model predictions for the short packed bed column tests were compared with the experimentally obtained breakthrough curves. The Pore Surface Diffusion model has the capability to predict the performance of a packed bed reactor configuration by employing pseudo-equilibrium data, which require significantly less effort to complete. Consequently, the ultimate value of this model is to save time by completing quick batch reactors instead of lengthy column studies.
Arsenate and nitrate were selected as model contaminants because their groundwater prevalence across the globe and distinct coordination chemistry. Arsenic, a well-known toxic agent, is anthropogenic and naturally occurring contaminant that has been designated as human carcinogen.15–19 Nitrate is a stable and non-ligand forming oxyanion that is prevalent in groundwater of many agricultural areas because of extensive fertilization. In addition to causing methemoglobinemia, recent findings suggest that nitrates may act as carcinogens.20–22
Experimental and modeling approaches
Fabrication and characterization of titanium dioxide nanomaterial-enhanced hybrid ion-exchange (Ti-HIX) media
The hybrid ion-exchange media was fabricated by in situ synthesis of nanostructured endoskeleton comprised of fused titanium dioxide nanoparticles following a published protocol by Elton et al.14 In brief, 50 mL of strong base nitrate selective ion-exchange resin (Resintech SIR-100-HP) with quaternary amine functional groups were soaked in ultrapure water (<1 μS cm−1) to achieve maximum volume increase and pore opening of the resin by hydration. An aliquot of 100 mL saturated solution of TiOSO4 (ACS grade, Sigma-Aldrich) was mixed with the wet ion-exchange resin for a period of 5 minutes, followed by decanting of the excess saturated solution and 24 hour hydrolysis at 80 °C in a closed vessel. Upon hydrolysis, the new hybrid media was repeatedly rinsed with ultrapure water until the pH > 4, soaked overnight in 5% NaCl to remove excess sulfate and convert the quaternary amine sites in chloride form.
Bulk properties of the hybrid ion-exchange media, such as porosity and density, were determined using pycnometer analysis following a protocol described by Hristovski et al.23 Gravimetric analysis was used to determine TiO2 content in the hybrid resin.24 High resolution XRD (Pananalytical X'Pert Pro) was used to determine the crystalline structure and confirm formation of titanium dioxide. Focused ion beam and scanning electron microscope with energy dispersion X-ray microanalysis (FIB/SEM-EDX) techniques were employed to determine the size and shape of the hybrid ion-exchange media and confirm presence of the TiO2 nanostructured endoskeleton within media (Nova 200 NanoLab UHR FEG-SEM/FIB).
Arsenic and nitrate removal under pseudo-equilibrium conditions
Batch pseudo-equilibrium tests and isotherm modeling were conducted to develop Freundlich isotherm parameters, which are necessary input parameters for the PSDM. The batch pseudo-equilibrium tests were performed in 5 mM NaHCHO3 buffered ultrapure water at final pH = 7.2 ± 0.3 and contact time of three days, which have been demonstrated to be sufficient to achieve sorption pseudo-equilibrium in nanostructured media.14,25 Elton et al.14 demonstrated that even high pH variability of >0.3 have negligible effects on arsenic sorption capacity of this hybrid media because of the strong Donnan effect generated by the quaternary amine groups of the ion-exchange media. The initial concentration of C0 ≈ 108 μg L−1 As as arsenate (KH2AsO4 in KOH standard solution; Sigma-Aldrich) was considered because it represents a realistic arsenic concentration occurring in natural waters. Considering that linear correlation generally exists between the equilibrium and the ion-exchange (sorption) capacity in ion-exchange resins (i.e. Freundlich intensity parameter is 1/n ≈ 1), low initial nitrate concentration of ~1.1 mg L−1 NO3−-N (~5 mg L−1 NO3−) was selected (NaNO3 – nitrate standard solution for Ion Chromatography; Sigma-Aldrich).10,14,26
The Freundlich isotherm model was used to derive the sorption capacity descriptors K and 1/n necessary as input in the PSDM (eqn (1)):
where
q ≡ the sorption capacity ([M] adsorbate [M]
−1 adsorbent;
K ≡ the Freundlich sorption capacity parameter ([M] adsorbate [M]
−1 adsorbent)([L]
3 [M]
−1 adsorbate)
1/n;
CE ≡ the equilibrium concentration of adsorbate in solution ([M] adsorbate [L]
−3); and 1/
n ≡ the Freundlich sorption intensity parameter (unitless).
Arsenic was analyzed using Graphite Furnace Atomic Absorption Spectroscopy (GF-AAS) (GTA 110-Varian 50B) according to US EPA method 200.9.27 Nitrate was analyzed using ion chromatography (IC) (Dionex IS-2000) according to method US EPA method 300.0.28
Arsenic and nitrate removal in short packed bed columns under continuous flow regime
Short packed bed column tests were conducted to assess the arsenate and nitrate removal performance of the hybrid media under continuous flow conditions and obtain breakthrough curves for both contaminants. In brief, 4.35 g of hybrid media (dry weight) were packed atop a glass wool support in a column with diameter of 1.5 cm to constitute a packed bed with depth of 5.7 cm. Glass beads were placed under above and below the packed bed to ensure evenly distributed flow. The hybrid media size ranged between 0.3 and 1.1 mm with a geometric average of 0.7 mm and provided a dColumn/dP ratio of ~21. The wall effect on mass transfer can be neglected for dColumn/dP ratios >20 according to Benenati and Brosilow29 and Chu and Ng.30 The column was operated at a high-end realistic loading rate of 2.08 L m−2 s−1 and EBCT ≈ 27.5 s, which were sufficient to capture the entire mass transfer zone for both contaminants.
The short packed bed column test was conducted with a 5 mM NaHCO3 buffered ultrapure water with pH ≈ 7.2 and C0 ≈ 105 μg L−1 As as arsenate. Realistic nitrate concentrations of C0 ≈ 20.8 mg L−1 NO3−-N were employed in the tests to ensure that complete nitrate breakthrough occurs before the complete arsenic breakthrough. Effluent from the column test was collected in 30 mL sample aliquots, which represented an average of about 3 bed volumes.
The column tests were operated until complete breakthrough, defined as CEffluent/C0 ≥ 0.95, was achieved for both contaminants. The total sorbed contaminant mass was estimated by calculating the area above the breakthrough curve as described with eqn (2):31
|  | (2) |
where
mCont ≡ the total mass of sorbed contaminant onto the hybrid media ([M]);
Q ≡ the flowrate in the packed bed ([L]
3[T]);
CSORB(
t) ≡ the difference between the initial and effluent contaminant concentrations at time
t ([M][L]
−3).
The continuous flow sorption capacity for both contaminants was obtained by dividing the total mass of sorbed contaminant with the dry mass of the hybrid media used in the packed bed (eqn (3)):
| 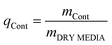 | (3) |
where
mDRY MEDIA ≡ the mass of the dry hybrid adsorbent media used in the packed bed column ([M] dry media); and
qCont ≡ the sorption capacity of the hybrid media ([M] contaminant [M] dry media
−1).
Because a complete conversion of the quaternary amines in chloride form was not achieved by overnight soaking of the hybrid media resin in NaCl after the hydrolysis of the titanium precursor, a small amount of sulfate was still present in the hybrid resin, which affected the kinetics and shape of the nitrate breakthrough curve. To address this problem, upon completion of the first column test, the media was regenerated with 2% KOH/1% KCl solution followed by 0.1% HCl solution at a hydraulic loading rate of ~0.4 L m−2 s−1 and EBCT of ~2.5 minutes for a period of 24 hours upon completion of the first column test. This process allowed for complete conversion of the hybrid ion exchange media in a chloride form, and allowed to assess the regeneration efficiency of the media. A strong base solution (KOH or NaOH) is typically used in arsenic regeneration, while chloride salt is used to regenerate the quaternary amines into chloride form.32–34 To ensure that (1) alkaline metals, which may cause TiO2 surface poisoning, are removed; and (2) the local pore pH is not alkaline, which may impair arsenic sorption, a dilute solution of hydrochloric acid was applied.35,36 The last regeneration step is not possible with iron based media because iron (hydr)oxides have the ability to dissolve in low pH. This, however, is not the case with TiO2, which is extremely stable dilute in strong acid environments. The regeneration efficiency (Eff) was calculated using eqn (4):
| 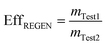 | (4) |
where
mTest1 and
mTest2 were the total mass of the removed contaminant during the initial column testing, and the testing following the regeneration procedure.
Short packed bed column modeling using the pore surface diffusion model
The pore surface diffusion model (PSDM) was used to predict arsenic and nitrate breakthrough curves for the experimental operating conditions, and test the underlying hypothesis that this model could be used as a rapid screening tool when developing similar hybrid ion-exchange media. The PSDM is a dynamic fixed bed model that incorporates a set of assumptions and governing partial differential equations that describe sorbent dynamics in a fixed bed setup. PSDM simulations were conducted using AdDesignS™ software (Michigan Technological University).37 The estimates for the film mass transport coefficients kf were based on the Gnielinski correlation, and the tortuosity and pore diffusion coefficient estimates were based on Mackie and Meares correlations for electrolytes.11,23 A detailed explanation of the estimates and their values are presented in the supplemental information.38–40
Results and discussion
Properties of the fabricated titanium dioxide nanomaterial-enhanced hybrid ion-exchange (Ti-HIX) media
The titanium content of the synthesized hybrid media was estimated gravimetrically at about 16.6%, and correlated well with the titanium content of about 17.8% provided by the EDX microanalysis. The FIB/SEM and EDX microanalysis mapping presented in Fig. 1 confirmed the findings by Elton et al.14 that the titanium is evenly distributed throughout the inner surface of the porous media in a form of endoskeleton comprised of fused titanium dioxide nanoparticles. The endoskeleton appears not to be chemically bound to the surface of the ion-exchange resin, but rather forms a separate physical structure that is enmeshed into the porous structure of the resin. This condition allows for simultaneous removal of contaminants because both ion-exchange groups and TiO2 nanoparticles are exposed to the contaminated liquid diffusing into the pores of the media.
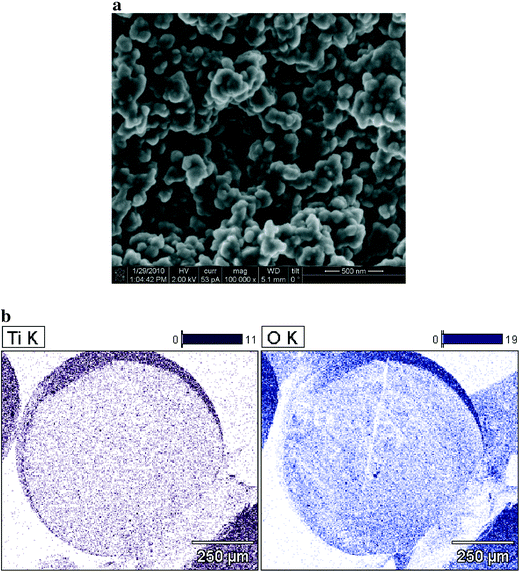 |
| Fig. 1 a. Focus Ion Beam/Scanning Electron microscope image of a pore inside the titanium-dioxide-based hybrid ion-exchange media showing white clusters of titanium dioxide nanoparticles coating the pores of the ion-exchange resin. b. Focus Ion Beam/Scanning Electron microscope image of a crossection of titanium-dioxide-based hybrid ion-exchange media. | |
The XRD analysis, presented in Fig. S1,† confirmed the presence of anatase crystalline structure; however, the nanoparticle shapes, presented in Fig. 1, suggest that large portion of the titanium dioxide has amorphous character, which is beneficial for sorption of weak acid oxyanions like arsenic.41–45
The SEM examination revealed that the particle sizes ranged between 0.5 mm and 1 mm with an estimated average size of 0.7 mm. The particle porosity and bulk density, based on the pycnometer estimates, were εP ≈ 0.75 and ρb = 1.18, respectively. High particle porosity is beneficial because it facilitates faster intraparticle mass transport. This consequently allows for shorter mass transfer zones and empty bed contact times (EBCT), which are advantageous for designing small point-of-use or point-of-entry systems capable of operating at high hydraulic loading rates and short EBCTs.11,12,46,47
Arsenic and nitrate removal performance Ti-HIX under pseudo-equilibrium conditions in a batch reactor mode
Fig. S2 and S3† illustrate the results from the batch reactor tests conducted under pseudo-equilibrium conditions. The Freundlich adsorption capacity parameters for arsenate and nitrate were estimated at K(As) ≈ 102 (μg g−1)(L μg−1)1/n and K(N-NO3−) ≈ 4.49 (mg g−1)(L mg−1)1/n, respectively. The Freundlich intensity parameter for arsenic was 1/n ≈ 0.54 (R2 = 0.968). Values for 1/n < 1 are associated with favorable sorption processes and good performances of sorbents at low influent contaminant concentrations. The Freundlich intensity parameter for nitrate was 1/n ≈ 1.02 (R2 = 0.988), indicating linear partitioning of the contaminant between the bulk solution and the surface of the media, which is typical for ion-exchange mechanisms.
Arsenic and nitrate removal performance of original and regenerated hybrid Ti-HIX under continuous flow regime
Fig. 2 illustrates the breakthrough curve for original and regenerated titanium dioxide nanomaterial-enhanced hybrid ion-exchange (Ti-HIX) media and summarizes the maximum sorption capacity at C/C0 = 95%, which is considered a complete breakthrough ratio.48
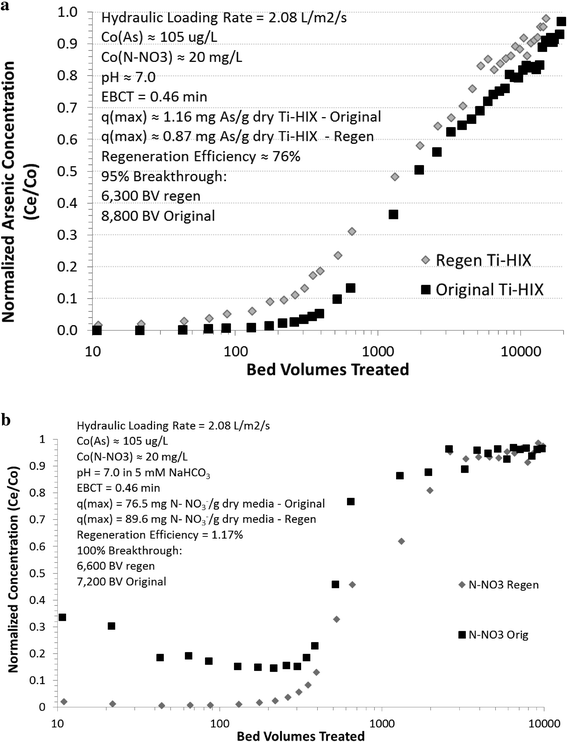 |
| Fig. 2 a. Arsenic breakthrough curves for original and regenerated titanium dioxide nanomaterial-enhanced hybrid ion exchange media under continuous flow regime. b. Nitrogen-nitrate breakthrough curves for original and regenerated titanium dioxide nanomaterial-enhanced hybrid ion exchange media under continuous flow regime. | |
At arsenic influent concentration of 105 μg L−1, the original Ti-HIX media was able to treat about 8800 bed volumes (BVs) before a complete breakthrough occurred, which corresponded to sorption capacity of qmax ≈ 1.16 mg As g−1 dry Ti-HIX media. The sorption capacity at 95% breakthrough represented >92% of the maximum sorption capacity (q0 ≈ 1.25 mg As g−1 dry media) predicted by the Freundlich isotherm model (q = 102 (μg g−1)(L μg−1)1/nC0.540) for pseudo-equilibrium conditions. Considering that the column test was not operated to 100% breakthrough and pseudo-equilibrium conditions, the <3% difference could be characterized as small and realistic experimental error.
Upon regeneration, the maximum arsenic sorption capacity of the Ti-HIX media under continuous flow conditions decreased to qR ≈ 0.87 mg As g−1 dry Ti-HIX media or ~6300 BVs, which represented about 76% of the original Ti-HIX's capacity. To achieve such high regeneration efficiencies with iron (hydr)oxide media, a high concentration mixtures of NaOH and NaCl at elevated temperatures are often required.49–51 Considering that it was beyond the scope of this study to optimize regeneration processes, it could be postulated that optimization of the regeneration process could yield much higher regeneration efficiencies under optimized conditions.
In contrast to the decrease in arsenic sorption capacity, nitrate removal capacity did not decrease upon regeneration of the media. Interestingly, Ti-HIX media capacity increased from 76.5 mg N-NO3− g−1 dry media before regeneration to 89.6 mg N-NO3− g−1 dry media after the regeneration, which yielded ~117% regeneration efficiency. This difference of about 17% in the nitrate removal capacity is sufficiently large to be overlooked as simply being an experimental variability although a large portion of this percentage could be attributed to experimental error. Comparison of the breakthrough curves in Fig. 4, however, suggests that this difference could be attributed to variances in film mass-transport or ion-exchange capacity limitations resulting from competition by a strong acid oxyanion for the available ion-exchange sites.8,52–54 Considering that the breakthrough curve after regeneration did not exhibit any characteristics of film mass-transport limitation under the same operating conditions, the shape of the breakthrough curve before regeneration was attributed to limitations in ion-exchange capacity. Examination of sulfate elution data and comparison of the maximum sorption capacity after regeneration with the one predicted under pseudo-equilibrium conditions confirmed these postulations. As illustrated in Fig. S4 (ESI†), the NaCl wash did not completely remove the sulfate, which is generated during hydrolysis of the titanium precursor. However, dilute HCl was applied during the regeneration process, which was able to exchange the sulfate for chloride ions and eliminate this impediment. This was supported by the finding that the nitrogen as nitrate capacity at breakthrough after regeneration with HCl represented >94% of the maximum sorption capacity (q0 ≈ 95.3 mg N-NO3− g−1 dry media) as predicted by the Freundlich isotherm model (q = 4.49 (mg g−1)(L mg−1)1/nC1.020). Although the overall nitrate removal capacity was not permanently impaired, these findings implied that incomplete rinsing and conversion of the quaternary amine sites back to chlorine form during fabrication could significantly affect the overall kinetics of this hybrid media.
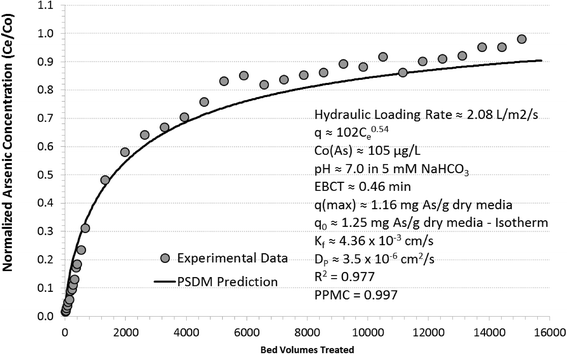 |
| Fig. 3 Arsenic breakthrough curves according to experimental data and Pore Surface Diffusion Model predictions under the same experimental conditions. | |
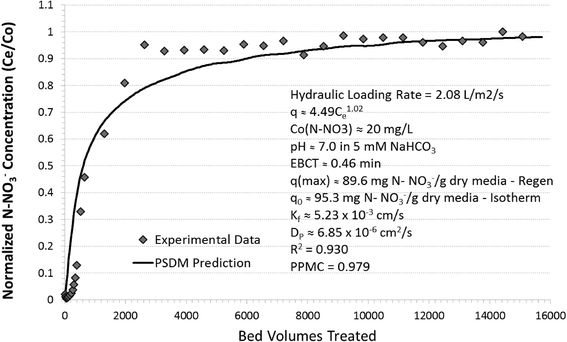 |
| Fig. 4 Nitrogen-nitrate breakthrough curves according to experimental data and Pore Surface Diffusion Model predictions under the same experimental conditions. | |
Pore surface model predictions and their short packed Bed column verification
Fig. 3 illustrates the arsenic breakthrough curve experimental data and PSDM prediction for the same experimental conditions. A relatively high goodness of fit parameter of R2 ≈ 0.977 and Pearson Product-Moment Correlation coefficient of PPMC ≈ 0.997 supported the underlying hypothesis that the model is able to provide satisfactory breakthrough curve predictions with respect to arsenic.
Although it appears that the model was able to predict the initial rapid breakthrough section of the curve (Ce/C0 < 0.5) slightly better than the latter, intraparticle mass-transport limited section of the curve, this negligible difference in the corresponding goodness of fit values (RI2 ≈ 0.981 and RL2 ≈ 0.973) could be attributed to variability of analytics.26,52–55
The correlation between the experimental data and the PSDM prediction for the nitrate breakthrough curve was slightly lower (R2 ≈ 0.930 and PMCC ≈ 0.979) than the one for arsenic, but still sufficiently high to support the underlying hypothesis. Fig. 4 illustrates a PSDM prediction characterized by a rapid initial increase in nitrate concentration followed by a gradual breakthrough. The rapid increase is anticipated with metal (hydr)oxide media, which is surface diffusion restrictive. The limited sorption sites located in the outermost surfaces of the metal (hydr)oxides become occupied by inner-sphere complexes, providing for longer times to be needed for oxyanions to diffuse inside the media and interacts with available sites. Interestingly, however, there is a slight difference of 20 BVs between the experimental data and model predictions in the initial section of the nitrate breakthrough curve.
Implications for development of nanomaterial enabled hybrid ion-exchange media
Development and optimization of novel hybrid-ion exchange media is a costly and time-consuming process because it necessitates examination of the media's performance in continuous flow configurations. Packed bed simulations are significantly and shorter than fabricating large quantities of new hybrid media to be tested in a long-duration continuous flow tests, which often may require several weeks or months of performance monitoring, sample collection and analysis. By demonstrating that the PSDM could successfully be used to simulate performance of hybrid ion-exchange media in a packed bed configurations, this model could be employed to rapidly screen potentially promising new hybrid media by also considering other factors than adsorption capacity alone. Factors, such as particle size and configuration, porosity, and nanoparticle distribution inside the media, significantly affect the limiting transport mechanisms in continuous configurations and could render any promising hybrid ion-exchange media unsuitable for use in packed bed systems although they exhibit high capacity for removal of contaminants under-pseudo-equilibrium conditions.
Conclusions
Titanium dioxide nanomaterial-enhanced hybrid ion-exchange media represent a new class of hybrid materials capable of simultaneous removal of multiple contaminants. The stability of titanium dioxide nanostructured endoskeleton minimizes the change of resin's volume due to hydration and allows for media regeneration using HCl. This acid rinse, which could not be applied iron based hybrid ion exchange media, is essential in (1) decreasing the local pore pH after the alkaline regeneration; and (2) removing any potential metal oxide surface poisoning resulting from high concentration of alkaline ions. Both high pH and alkaline metal poisoning have the potential to significantly decrease sorption capacity for oxyanion.56 Although high regeneration efficiency for arsenate was demonstrated with the regeneration process used in this study, further process optimization could lead to achieve 100% regeneration efficiencies for both for arsenate and nitrate.
The pore surface diffusion model could successfully be used to predict the performance of the packed bed column test and reduce the time necessary for development and optimization of new hybrid media that incorporate similar nanostructured endoskeleton structures. The model was able to predict the experimental data with values for R2 > 0.9 for both nitrate and arsenate. However, the findings clarified that simple soaking and rinsing in 5% NaCl may not be sufficient to remove excess sulfate and convert the quaternary amine sites in chloride form. Instead, a thorough regeneration of the quaternary amine groups needs to be conducted before using the novel media.
References
- B. Petrusevski, J. Boere, S. M. Shahidullah, S. K. Sharma and J. C. Schippers, J. Water Supply: Res. Technol.--AQUA, 2002, 51.3, 135–144 Search PubMed.
- D. Mohan and C. U. Pittmann Jr., J. Hazard. Mater., 2007, 142, 1–53 CrossRef CAS PubMed.
- S. Sarkar, P. K. Chatterjee, L. H. Cumbal and A. K. SenGupta, Chem. Eng., 2010, 166, 923–931 CrossRef PubMed.
- N. Savage and M. S. Diallo, J. Nanopart. Res., 2005, 7, 331–342 CrossRef CAS.
- A. A. L. S. Duarte, S. J. A. Cardoso and A. J. Alcada, Sustainability, 2009, 1, 1288–1304 CrossRef CAS PubMed.
- I. Ali, Chem. Rev., 2012, 112(10), 5073–5091, DOI:10.1021/cr300133d.
- J. C. Crittenden, N. J. Hutzler and D. G. Geyer, Water Resour. Res., 1986, 22, 271–284 CrossRef CAS.
- K. Hristovski, P. Westerhoff and J. Crittenden, J. Hazard. Mater., 2008, 156, 604–611 CrossRef CAS PubMed.
- K. Hristovski, A. Baumgardner and P. Westerhoff, J. Hazard. Mater., 2007, 147, 265–274 CrossRef CAS PubMed.
- K. Hristovski, P. Westerhoff, T. Möller, P. Sylvester, W. Condit and H. Mash, J. Hazard. Mater., 2008, 152, 397–406 CrossRef CAS PubMed.
- K. D. Hristovski, P. K. Westerhoff, J. C. Crittenden and L. W. Olson, Sep. Sci. Technol., 2008, 43, 3154–3167 CrossRef CAS PubMed.
- D. Yan, D. D. Gang, N. Zhang and L. Lin, J. Environ. Eng., 2013, 139, 213–219, DOI:10.1061/(ASCE)EE.1943-7870.0000633.
- K. W. Pepper, D. Reichenberg and D. K. Hale, J. Chem. Soc., 1952, 3129–3136 RSC.
-
J. Elton, K. Hristovski and P. Westerhoff, in Novel Solutions to Water Pollution, Book Series: ACS Symposium Series, ed. S. Ahuja and K. Hristovski, 2013, vol. 1123, pp. 223–236, DOI:10.1021/bk-2013-1123.ch013.
- T. S. Y. Choong, T. G. Chuah, Y. Robiah, F. L. G. Koay and I. Anzi, Desalination, 2007, 217, 139–156 CrossRef CAS PubMed.
- M. Bissen and F. H. Frimmel, Acta Hydrochim. Hydrobiol., 2003, 31, 9–18 CrossRef CAS PubMed.
- M. F. Hughes, Toxicol. Lett., 2009, 133, 1–16 CrossRef.
- P. L. Smedley and D. G. Kinniburgh, Appl. Geochem., 2002, 17, 517–568 CrossRef CAS.
- B. K. Mandal and K. T. Suzuki, Talanta, 2002, 58, 201–235 CrossRef CAS.
- P. Santamaria, J. Sci. Food Agric., 2006, 86, 10–17 CrossRef CAS PubMed.
- M. Cornblath and A. F. Hartmann, J. Pediatr., 1948, 33, 421–425 CrossRef CAS.
- G. E. Njeze, U. Dilibe and C. Ilo, Niger. J. Clin. Pract., 2014, 17(2), 178–182 CrossRef CAS PubMed.
- K. D. Hristovski, P. K. Westerhoff, J. Crittenden and L. Olson, Environ. Sci. Technol., 2008, 42, 3786–3790 CrossRef CAS.
- L. T. Black, G. E. Hamerstrand, F. S. Nakayama and B. A. Rasnik, Rubber Chem. Technol., 1983, 56(2), 367–371 CrossRef CAS.
- K. D. Hristovski, P. K. Westerhoff, T. Möller and P. Sylvester, Chem. Eng. J., 2009, 146, 237–243 CrossRef CAS PubMed.
-
J. C. Crittenden, D. W. Trussell, K. J. Hand and G. Howe, in Water Treatment: Principles and Design, ed. Tchobanoglous, Wiley & Sons, Inc., Hoboken, New Jersey, USA, 2nd edn, 2005 Search PubMed.
-
J. T. Creed, T. D. Martin and J. W. O'Dell, Determination of trace elements by stabilized temperature Graphite Furnace Atomic Absorption, US. Environmental Protection Agency- Method 200.9, 1994 Search PubMed.
-
J. D. Pfaff, Determination of inorganic anions by ion chromatography, US. Environmental Protection Agency- Method 300.0, 1993 Search PubMed.
- R. F. Benenati and C. B. Brosilow, AIChE J., 1962, 8(3), 351–361 CrossRef PubMed.
- C. F. Chu and K. M. Ng, AIChE J., 1989, 35(1), 148–158 CrossRef CAS PubMed.
- R. Sandoval, A. M. Cooper, K. Aymar, A. Jain and K. Hristovski, J. Hazard. Mater., 2011, 193, 296–303 CrossRef CAS PubMed.
- D. Mohan and C. U. Pittman Jr., J. Hazard. Mater., 2007, 142, 1–53 CrossRef CAS PubMed.
- S. Tresintsi, K. Simeonidis, M. Katsikini, E. C. Paloura, G. Bantsis and M. Mitrakas, J. Hazard. Mater., 2014, 265, 217–225 CrossRef CAS PubMed.
- J. C. Lin and A. K. SenGupta, Environ. Eng. Sci., 2009, 26(11), 1673–1683 CrossRef CAS.
- P. K. Dutta, A. K. Ray, V. K. Sharma and F. J. Millero, J. Colloid Interface Sci., 2004, 278, 270–275 CrossRef CAS PubMed.
- G. Jegadeesan, S. R. Al-Abed, V. Sundaram, H. Choi, K. G. Scheckel and D. D. Dionysiou, Water Res., 2010, 44, 965–973 CrossRef CAS PubMed.
-
K. A. Mertz, F. Gobin, D. W. Hand, D. R. Hokanson and J. C. Crittenden, Manual: adsorption design software for Windows (Ad-DesignS), Michigan Technological University, Houghton, Michigan, 1999 Search PubMed.
-
H. Sontheimer, J. Crittenden and S. Summers, in Activated Carbon for Water Treatment, DVGW-Forschungsstelle, Engler- Bunte Institut, Universitat Karlsruhe, Karlsruhe, Germany, 2nd edn, 1988 Search PubMed.
-
D. Lide, in CRC Handbook of Chemistry and Physics, ed. Taylor and Francis Group, 87th edn, Boca Raton, FL, 2006 Search PubMed.
-
D. M. LeVan, G. Carta and C. M. Yon, in Perry's Chemical Engineers' Handbook, ed. R. D. Perry and D. W. Green, McGraw-Hill, New York, 7th edn, 1997, ch. 16 Search PubMed.
- A. S. Barbard and L. A. Curtiss, Nano Lett., 2005, 7(5), 1261–1266 CrossRef.
-
X. G. Meng, M. Dadachov, G. P. Korfiatis and C. Christodoulatos, US Pat. 7497952 B2, 2009 Search PubMed.
- M. E. Pena, G. P. Korfiatis, M. Patel, L. Lippincott and X. Meng, Water Res., 2005, 39, 2327–2337 CrossRef CAS PubMed.
- B. Prasai, B. Cai, M. K. Underwood, J. P. Lewis and D. A. Drabold, J. Mater. Sci., 2012, 47(21), 7515–7521, DOI:10.1007/s10853-012-6439-6.
- M. Pena, X. Meng, G. P. Korfiatis and C. Jing, Environ. Sci. Technol., 2006, 40, 1257–1262 CrossRef CAS.
- N. V. Medvidović, J. Perić and M. Trgo, Sep. Purif. Technol., 2006, 49, 237–244 CrossRef PubMed.
-
U.S. Environmental Protection Agency (EPA), in Arsenic Treatment Technology Evaluation Handbook for Small Systems, Office of Water (4606 M), EPA 816-R-03-014, 2003.
-
F. Cecen and Ö. Aktas, Activated Carbon for Water and Wastewater Treatment, Integration of Adsorption and Biological Treatment, Wiley-VCH Verlag & Co. KGaA, Boschstr. 12, 69469 Weinheim, Germany, 2012 Search PubMed.
- P. Sylvester, P. Westerhoff, T. Möller, M. Badruzzaman and O. Boyd, J. Environ. Eng. Sci., 2007, 24(1), 104–112 CrossRef CAS.
- S. Kundu and A. K. Gupta, J. Colloid Interface Sci., 2005, 290, 52–60 CrossRef CAS PubMed.
- A. Hutson, S. Ko and S. G. Huling, Chemosphere, 2012, 89, 1218–1223 CrossRef CAS PubMed.
- Z. Ma, R. D. Whitley and N.-H. L. Wang, AIChE J., 2004, 42(5), 1244–1262 CrossRef PubMed.
- E. Furuya, Y. Takeuchi and K. E. Noll, J. Chem. Eng. Jpn., 1989, 22(6), 670–676 CrossRef CAS.
- J. A. Berninger, R. D. Whitley, X. Zhang and N.-H. L. Wang, Comput. Chem. Eng., 1991, 15(11), 749–768 CrossRef CAS.
- S. M. Robinson, W. D. Arnold and C. H. Byers, AIChE J., 1994, 40(12), 2045–2064 CrossRef CAS PubMed.
- L. Chen, J. Li and M. Ge, Chem. Eng. J., 2011, 70(2–3), 531–537 CrossRef PubMed.
Footnote |
† Electronic supplementary information (ESI) available. See DOI: 10.1039/c5ew00108k |
|
This journal is © The Royal Society of Chemistry 2015 |
Click here to see how this site uses Cookies. View our privacy policy here.