Energy-efficient desalination by forward osmosis using responsive ionic liquid draw solutes†
Received
28th October 2014
, Accepted 11th February 2015
First published on 11th February 2015
Abstract
We demonstrate a class of thermally responsive ionic liquids (ILs) as novel draw solutes for forward osmosis (FO) seawater desalination with electrical energy consumption significantly lower than that of seawater reverse osmosis (RO). These draw solutes can draw water from feed solutions of up to 1.6 M NaCl and hence have potential to treat high salinity feed streams including RO brine. Draw solution regeneration can be achieved energy-efficiently via a thermally stimulated phase separation process using less expensive low grade heat in combination with electrically driven nanofiltration. The electrical energy consumption is estimated to be as low as 16% of that needed for the state-of-the-art RO desalination leading to significant reduction of energy cost and carbon footprint.
Water impact
One of the key challenges in the forward osmosis (FO) process is the lack of suitable draw solutes that can be efficiently regenerated. Thermally responsive ionic liquids are demonstrated as novel draw solutes for FO desalination with substantially lower electrical energy consumption. These draw solutes possess high osmolality and have potential to be used for the treatment of high salinity feed streams. Their regeneration is carried out via thermally stimulated liquid–liquid phase separation, achievable using low grade waste heat in combination with nanofiltration. It is envisaged that these findings could motivate the proliferation of FO processes by offering low carbon footprint and lower-cost desalination, and also enable treatment of industrial wastewater streams which are otherwise difficult to handle.
|
Introduction
Water scarcity has been recognised as a global crisis.1 While wastewater reclamation partially relieves water shortages, only desalination technologies can broaden our supply to the ocean, which is the major source of water. One of the prevalent seawater desalination technologies is reverse osmosis (RO) whose energy consumption has been greatly reduced after decades of development.2 However, the state-of-art RO still requires >2 kWh m−3 of electrical energy at 50% recovery which is about double the theoretical minimum.2 In addition, the high pressure needed in RO and the concomitant membrane fouling3 are still inherent drawbacks of reverse osmosis. Although some membrane-free desalination technologies4,5 have been proposed, the promising forward osmosis (FO) membrane desalination technology is considered as a potentially viable energy efficient process.6 The water permeation in FO is virtually an energy-input-free process driven by the osmotic pressure difference between the draw solution and feed solution. Besides, membrane fouling is potentially much less severe due to substantially lower hydraulic pressure.7
Despite the rapid progress in membrane technology,8 viable FO applications so far are limited to specific cases where diluted draw solutions are utilized without regeneration, e.g., when fertilizers are used as draw solutes.9–11 The lack of suitable regenerable draw solutes has become the obstacle jeopardizing the future of FO desalination by negating its promised advantages. The need for an ideal balance between high osmotic pressure and ease of regeneration is the obvious target in principle, which is extremely challenging to realize in practice. So far, the choice of draw solutes is still very limited. Many non-responsive draw solutes including inorganic and organic salts,12,13 polyelectrolytes,14 glucose15 and hydroacid complexes16 can generate sufficiently high osmotic pressure for seawater desalination. However, their use is limited due to the requirement of high electrical energy-consuming processes to separate water from the diluted draw solutions. In fact, substantially more energy input is required, with the minimum dictated by the thermodynamics for solute/solvent separation. In these cases, the hydraulic pressure involved needs to be higher than the osmotic pressure of the diluted draw solution, which, by default, is higher than the osmotic pressure of the feed solution. Therefore, the net electrical energy consumption of FO desalination with such draw solutes is unlikely to be lower than that of the best RO process. One effective method to significantly lower the electrical energy consumption for draw solute regeneration is to use ‘smart’ or responsive draw solutes. Such responsive draw solutes undergo certain changes upon external stimuli which enable them to be separated from the diluted draw solutions using low energy consuming methods such as microfiltration (MF) and nanofiltration. This allows substantial reduction of draw solute concentration in the diluted draw solution. Therefore, any subsequent process is for water recovery from a solution of substantially lower osmolality than the initial feed water. Hence we believe that the use of suitable smart draw solutes in FO holds the key to actually realize its promised advantage of low electrical energy consumption and enables it to complement the state-of-the-art RO process particularly for the treatment of difficult feed streams of high salinity. Up to now, magnetically,17,18 CO2
19,20 and thermally responsive draw solutes have been proposed. However, magnetically responsive nanoparticles suffer from low osmotic pressures, nanoparticle agglomeration and ineffective separation,21 and CO2 responsive draw solutes are quite promising but require extra processes such as incorporation and removal of CO2. Thermally responsive draw solutes are therefore attractive because of their simplicity, the absence of using extra chemicals and the possibility of using less expensive and clean energy sources such as solar thermal energy and low grade industrial waste heat. We have previously reported a new concept of quasi-continuous desalination using a thermally responsive hydrogel as an FO draw agent,22 which has a distinct advantage of exempting the tedious draw solute regeneration process, although development of new materials is needed to improve the water flux.22,23 In summary, FO draw solutes can be classified as either non-responsive or responsive. The responsive solutes can be further classified depending on the methods used for regeneration, i.e., chemical regeneration using acids or bases, magnetic field regeneration and thermal stimulus regeneration. So far, thermally responsive draw solutes are the most promising.
In this paper, we discuss novel thermally responsive ionic liquids as draw solutes for FO seawater desalination with lower electrical energy consumption than RO. While the traditional NIPAm24 based copolymer and other thermally responsive non-ionic oligomers25–27 can hardly draw water from seawater, these thermally responsive ionic liquids can draw water from 1.6 M NaCl feed solution, which has almost three times the salinity of normal seawater. When the temperature is increased to above the Lower Critical Solution Temperature (LCST, 32–49 °C), the diluted draw solution undergoes liquid–liquid phase separation. While the sediment bottom phase, containing a very high draw solute concentration, can be reused directly as the draw solution again without further treatment, the water-rich supernatant phase has an osmotic pressure of less than 6 bar. Therefore, a much lower hydraulic pressure and consequently a lower electrical energy than that of seawater (osmotic pressure ~27 bar) RO are needed in the regeneration process with a nanofiltration (NF) membrane assuming higher water permeability than an RO membrane. In addition, the quality of product water is much better than that from a thermolytic CO2/NH3 system.28 Although a few ionic liquids with LCST have been reported, the interest to date has been focused on reporting the phenomenon without detailed discussion or insight into potential applications.29–31 Our investigation of their potential as new FO draw solutes is motivated by the unique combination of their ionic characteristics, low molecular weight, low LCST and very special phase transition behaviour. We have demonstrated that this new class of draw solutes not only show promise for reducing the energy cost of FO desalination to be considerably lower than that of the RO process, but also enable the treatment of difficult feed streams with higher salinity than seawater.
Experimental section
Materials and instruments
Tributyloctyl phosphonium bromide was purchased from Wako Pure Chemical Industries. Sodium mesitylenesulfonate, sodium 2,4-dimethylbenzenesulfonate and tetrabutylphosphonium bromide were purchased from Tokyo Chemical Industry CO., Ltd. Anhydrous dichloromethane (>99.8%) and sodium chloride (>99.5%) were purchased from Sigma-Aldrich. All chemicals were used without further purification. The forward osmosis membrane used is a thin film composite membrane prepared according to a reported method.32 Tests indicate that it has a high level of compatibility with the draw solutes and there is only a slight change in salt rejection after exposure to the ionic liquids (Fig. S1, ESI†). Nanofiltration membranes with molecular weight cut-off (MWCO) of 270 Da and 90 Da were purchased from Dow FilmTec. The osmolality of draw solution was measured by cryoscopy (OSMOMAT 030, Gonotech). The FO water flux was measured in a cross-flow cell with a flow rate of 4 ml s−1 by monitoring the weight decrease in feed solution with time. The effective membrane area was fixed at 15 mm × 30 mm and oriented in the PRO test mode (selective layer towards draw solution) throughout this study (Fig. S2, ESI†). In principle, both PRO and FO (selective layer towards feed stream) test modes can be used. It is known that the FO test mode results in a lower flux due to dilutive internal concentration polarization. More detailed comparisons of the two test modes have been reported in earlier publications.33,34 The water flux and hydraulic pressure correlation in NF was done in a dead-end filtration setup at room temperature. The water content in the draw solution was measured by Karl Fischer titration. Viscosity was measured by a Physica MCR 101 rheometer (Anton Paar). Back diffusion of draw solute was measured by monitoring the total organic carbon (TOC) in feed solution. The melting point of pure ionic liquids and latent heat of draw solution phase transition were determined using differential scanning calorimetry (DSC-Q10, TA instruments).
Draw solute synthesis
Three thermally responsive draw solutes were studied in this paper. Their structures are illustrated in Fig. 1. Tetrabutylphosphonium 2,4-dimethylbenzenesulfonate (P4444DMBS) was synthesized through ion exchange reactions. Aqueous solutions of tetrabutylphosphonium bromide and a slightly more than equal molar amount of sodium 2,4-dimethylbenzenesulfonate were mixed to form roughly 40 wt% solution and stirred at room temperature for 24 hours. The ionic liquid was extracted with dichloromethane and washed with deionized water several times. The dichloromethane phase was then dried in vacuum (~1 mbar) at 100 °C until constant weight was reached to remove the organic solvent. Tetrabutylphosphonium mesitylenesulfonate (P4444TMBS) was prepared using a similar method to that for tetrabutylphosphonium bromide and sodium mesitylenesulfonate. The third ionic liquid tributyloctyl-phosphonium bromide (P4448Br) was used as received. P4444TMBS (1H, 400 MHz, CDCl3, δ/ppm relative to TMS): 0.89–0.92 (t, 12H, CH3), 1.42–1.47 (m, 16H, CH2), 2.17 (s, 3H, CH3), 2.24–2.31 (m, 8H, CH2), 2.66 (s, 6H, CH3), 6.75 (s, 2H, Ar–H). P4444DMBS (1H, 400 MHz, CDCl3, δ/ppm relative to TMS): 0.72–0.76 (t, 12H, CH3), 1.23–1.27 (m, 16H, CH2), 2.00–2.07 (m, 8H, CH2), 2.11 (s, 3H, CH3), 2.50 (s, 3H, CH3), 6.72–6.74 (d, 1H, Ar–H), 6.78 (s, 1H, Ar–H), 7.66–7.68 (d, 1H, Ar–H). At room temperature, P4444DMBS is a colorless viscous liquid while P4444TMBS and P4448Br are in wax form (DSC results in Fig. S3, ESI†).
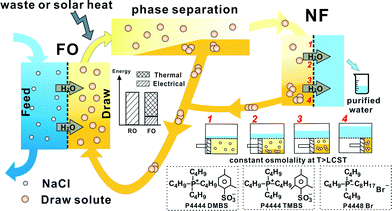 |
| Fig. 1 Schematics of FO desalination using thermally responsive ionic liquid draw solutes. | |
Results and discussion
Fig. 1 represents the entire FO desalination process using, as an example, 70 wt% aqueous solution of the thermally responsive ionic liquid as the draw solution. Driven by the osmotic pressure difference, desalinated water automatically permeates through the membrane from the feed solution, e.g., seawater, to dilute the draw solution in the FO process. While in the draw solute regeneration process, the diluted, e.g., 50 wt%, draw solution after FO undergoes liquid–liquid phase separation at a temperature above its LCST. The draw solute concentration in the supernatant is much reduced to typically less than 10 wt% and high quality water is obtained by a highly energy efficient low pressure nanofiltration (NF) process. Both the retentate in NF and the sediment from phase separation are pumped back to be used as draw solutions again, without the need for any further treatment, in the FO process to form a close loop. We envision a significant energy cost reduction by replacing the bulk of the electrical energy consumption with less expensive thermal energy, e.g., low grade waste heat.
Draw solute performance in the FO process
The design of the draw solutes conforms to the required balance for generating high osmotic pressure and easy regeneration. Unlike traditional ionic liquids which either dissolve in (hydrophilic) or repel water (hydrophobic), the thermally responsive ionic liquids are amphiphilic. The hydrophobicity from the alkyl groups in cations combined with the hydrophilicity from suitable anions imparts the LCST to their aqueous solutions. It is worth noting that the balance between hydrophilicity and hydrophobicity is extremely subtle that very small molecular structure modification might lead to a totally different solution behaviour. For instance, tetrabutylphosphonium benzenesulfonate (P4444BS) is highly soluble while tributylhexylphosphonium 2,4-dimethylbenzenesulfonate (P4446DMBS) is virtually insoluble in water. The unique characteristics of these ionic liquids as draw solutes can also be seen in Fig. 2a. The osmolality of these ionic liquid draw solutions increases monotonically but not linearly with molality unlike other conventional electrolytes.13 At low concentrations (e.g., <0.1 mol kg−1 or ~5 wt%), all three ionic liquids fully dissociate into relatively ‘free’ cations and anions so that their osmolality is approximately twice that of the molality expected for monovalent electrolytes. As their concentrations increase, the osmolality versus molality plots deviate from linearity. This is believed to be due to the hydrophobic association of the ionic liquid molecules.35 It also indicates that the hydrophobic interaction between P4448Br molecules is the strongest resulting in the most severe deviation from the ideal linear relationship between osmolality and molality. P4444DMBS and P4444TMBS are more hydrophilic and the osmolality values of their 30 wt% (~0.95 mol kg−1) solutions are 1.34 osmol kg−1 and 1.08 osmol kg−1, respectively, which are already higher than or approaching that of seawater (1.13 osmol kg−1). The osmolality of the draw solutions with higher concentrations cannot be measured by the cryoscopy method (ESI†). Therefore, we fitted the osmolality–molality correlation curves of these three draw solutes at lower concentrations and extrapolated them to higher concentrations (Fig. 2b and S4, ESI†). The estimated osmolality values in Fig. 2b give a reasonable trend at higher concentrations showing that P4444DMBS and P4444TMBS can generate very high osmotic pressures. For instance, the osmolality of 70 wt% solution is 5.4 osmol kg−1 for P4444DMBS and 3.6 osmol kg−1 for P4444TMBS, which is 4.5 and 3 times of seawater's osmolality, respectively. Such a high level of osmolality is attributed to the ionic nature of this class of responsive draw solutes. To further investigate this, the FO water flux as a function of draw solution concentration was measured with feed solutions of different salinities for P4444DMBS and P4444TMBS (Fig. 2c and S5, ESI†). FO measurement was not systematically carried out using P4448Br because of its low osmolality. Fig 2c shows that P4444DMBS generates a higher water flux than P4444TMBS with the same feed solution salinity since P4444DMBS is more hydrophilic. In fact, both P4444DMBS and P4444TMBS can generate reasonable FO water flux against seawater while the most hydrophobic P4448Br can only draw water from low salinity feed solutions (Fig. S6, ESI†). The water flux study corroborates well with the trend predicted using osmolality values. It is worth mentioning that the osmolality was measured by a cryoscopic method at subzero degree Celsius, thus the actual osmolality values of such LCST-type draw solutions at room temperature might be lower27 due to stronger molecular interactions at higher temperatures. It can be observed in Fig. S5† that, at room temperature, P4444DMBS at concentrations below 50 wt% is unable to generate a measurable FO flux against seawater feed even though at 30 wt% (~0.95 mol kg−1) the P4444DMBS draw solution already has an osmolality higher than that of seawater as shown in Fig. 2a. Therefore, we anticipate that at reduced temperatures, such LCST-type draw solutes would generate even higher FO drawing ability. Indeed the water fluxes of both P4444DMBS and P4444TMBS measured at 14 ± 1 °C are significantly higher than the corresponding values measured at 24 ± 1 °C (Fig. S5, ESI†). Furthermore, Fig. 2d shows that 70 wt% P4444DMBS draw solution can generate a water flux of 1.5 LMH against a feed of 1.2 M NaCl at 14 ± 1 °C. In fact, it can even generate a measureable water flux from 1.6 M NaCl feed solution whose salinity is 2.7 times higher than that of seawater. The results indicate that this new class of thermally responsive ionic liquid draw solutes may have potential to be utilized for treatment of difficult streams such as RO brine and other produced water including industrial waste streams or product streams such as those from mining and oil and shale gas industries. Another desirable characteristic of these draw solutes is their low back diffusion, i.e., below 30 mg L−1 (Fig. S7, ESI†) which is much lower than those reported for NaCl (750 mg L−1) and NH4HCO3 (>2000 mg L−1).36
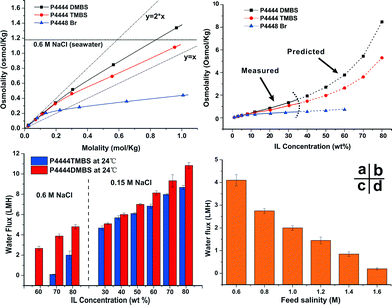 |
| Fig. 2 (a) Measured nonlinear correlation between osmolality and molality of the three draw solutes indicating different levels of molecular association. (b) Prediction of osmolality as a function of draw solute concentration beyond the instrument limit of cryoscopy. Prediction is according to the simulated functions as shown in Fig. S4, ESI.† (c) Water flux profile of P4444TMBS and P4444DMBS draw solutions at various concentrations against artificial seawater (0.6 M NaCl) and brackish water (0.15 M NaCl) feed solutions. (d) Water flux profile of 70 wt% P4444DMBS draw solution against feed solutions of higher salinity than seawater tested at 14 ± 1 °C. | |
Draw solute regeneration
After the FO process, the diluted draw solution needs to go through a regeneration process where the draw solute is separated from water. The phase diagrams of the three ILs in Fig. 3a show a U-shaped concentration dependence of their LCSTs. The absence of an LCST upturn for P4448Br at higher concentrations might be attributed to its relatively low solubility. The mechanism of LCST in solutions has been intensively studied and was found to be driven by unfavourable entropy of mixing,37 and the presence of LCST in an aqueous solution hinges on a subtle balance between hydrophilicity and hydrophobicity of solute molecules. The typical representatives are non-ionic PNIPAm, pluronics and cellulose derivatives.38–40 Although ionic molecules are rarely reported to possess LCST, we believe that the overall driving force of phase separation in our IL draw solutes is similar. The observed LCSTs between 30 to 50 °C for P4444DMBS or P4444TMBS are in the desirable range for thermally stimulated phase separation to take place using solar energy or waste heat. Moreover, the LCST actually decreases monotonically when 70 wt% draw solutions of P4444DMBS or P4444TMBS are diluted to 30 wt%, which means that only low grade heating is needed for phase separation during the regeneration process. For example, Fig. 3b shows that when the three draw solutions (30 wt%) are heated above their LCSTs, a clear liquid–liquid phase separation occurs in each of the draw solutions to form an IL-rich sediment and a water-rich supernatant phase. The most intriguing and also desirable point is that the draw solute concentration in the IL-rich phase increases to a plateau while that of the water-rich phase decreases to a plateau, when the phase separation is towards completion at higher temperatures, e.g., at about 16 °C above its corresponding LCST. Because the LCST transition behaviour of these thermally responsive ionic liquids has not been thoroughly studied, we also examined the thermally stimulated phase separation behaviour of the draw solutions with different initial concentrations. Fig. 3c shows that, at 16 °C above the corresponding LCST, the draw solute concentrations in the IL-rich sediment and water-rich supernatant are virtually constant regardless of the initial concentrations of these three draw solutes. In fact, stable phase separation can readily occur with a very mild temperature stimulus, e.g., at 35 and 40 °C for P4444TMBS and P4444DMBS, respectively, according to Fig. 3a. This allows the ionic liquids to be efficiently regenerated and then reused using low grade solar heat or industrial waste heat. For better clarity, a full cycle of FO, draw solute regeneration via liquid–liquid phase separation, and draw solute reuse is depicted on a schematic phase diagram in Fig. 3d. Using the most hydrophilic P4444DMBS as an example for practical FO application, the diluted draw solution at almost any concentration between 10 and 60 wt% (note: the actual level of dilution depends on the salinity of the feed stream.) phase separates at 55 °C into an IL-rich phase (70 wt% IL) which can be directly pumped back to be used again as the draw solution in FO without further treatment. In the meantime, the water-rich phase which has only 7.5 wt% IL and hence substantially lower osmotic pressure can be separated energy-efficiently by NF or RO. These characteristics are also favourable from a practical viewpoint because they enable a closed-loop process.
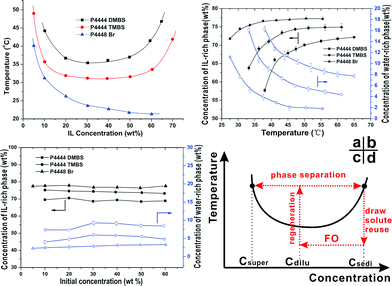 |
| Fig. 3 (a) LCSTs of the draw solutes at different concentrations. (b) Draw solute concentrations in the water-rich supernatants and IL-rich sediments after phase separation at different temperatures (initial concentration before phase separation fixed at 30 wt%). (c) Draw solute concentrations in the water-rich supernatants and IL-rich sediments at 16 °C above the LCST at different initial concentrations. (d) The entire desalination process illustrated in phase diagram. Csedi, Csuper and Cdilu represent the concentrations of IL-rich sediment phase, water-rich supernatant phase and diluted draw solution after FO, respectively. Note that Csedi coincides with the initial concentration of the FO draw solution. | |
Energy consumption estimation
Although the total energy needed for FO desalination may not be lower than that of RO, FO consumes much less electrical energy when a suitable responsive or regenerable draw solute is used. Among all the draw solutes studied to date, few have discussed energy issue with the exception of the ammonium bicarbonate system.41 In that work, thermal energy was used to raise the temperature above 60 °C to decompose the draw solute into carbon dioxide and ammonium and this was accompanied by significant water evaporation. A low equivalent energy consumption of 0.84 kWh m−3 was reported which raised some questions as it was perceived as having violated thermodynamics.42 Actually, the theoretical minimum energy required to separate solute from water is related to the solution's temperature, water activity and water recovery:2 | Wmin = − RT × ln(aw) × ln(1 / (1 − Y)) / Y | (1) |
where R is the gas constant, T is temperature, aw is the water activity and Y is the water recovery rate. By replacing water activity with osmotic pressure, which is an easily measured and often used parameter in desalination, we then introduce the following derivation.
By combining eqn (1) with eqn (2) which is the definition of osmotic pressure,
| Π = − RT × ln(aw) / Vw | (2) |
where
Vw is the molar volume of water, and Π is the osmotic pressure, we then have a new equation expressing the theoretical minimum energy requirement for desalination as a function of osmotic pressure and water recovery.
| Wmin = Vw × Π × ln(1 / (1 − Y)) / Y | (3) |
If water recovery approaches zero, or if osmotic pressure remains constant with water recovery, the theoretical minimum energy is:
Currently, the state-of-the-art RO energy consumption for seawater desalination is above 2 kWh m−3 with an imposed hydraulic pressure of up to 60 bar.2 For the thermally responsive draw solutes studied in this paper, their osmotic pressure in the water-rich supernatant was reduced to below 6 bar after the phase separation. As shown in Fig. 4a, a low hydraulic pressure of 7 bar is sufficient to produce a water flux of 17 LMH (for the water-rich phase of P4444TMBS) and 7.6 LMH (for the water-rich phase of P4444DMBS) using an NF membrane (MWCO 270 D). At 10 bar, a very high flux of 31 and 18 LMH were attained correspondingly, and this is comparable with, if not higher than, the water flux in a state-of-the-art seawater RO plant.43 It is worth emphasizing that in seawater RO, the hydraulic pressure needs to be progressively increased as the feed becomes concentrated to eventually become the brine; this is the reason why the theoretical minimum energy required increases with water recovery in RO. However, because of the phase behaviour of our responsive IL draw solutions, their osmotic pressure remains constant during water recovery cum draw solute regeneration process via NF or RO at temperatures above their respective LCST. As illustrated in Fig. 4b, a similar phase separation resumes or occurs again as water is recovered from the feed (which is the water-rich supernatant coming from FO) during NF. In this case, the IL concentrations of the water-rich phase and the IL-rich phase also remain constant (e.g., at 7.5 and 70 wt% for P4444DMBS). The only parameter that changes during NF is the declining volume ratio of the water-rich phase to the IL-rich phase as water is recovered (Fig. 4b and also inset in Fig. 1). At the end of NF, the retentate will be the IL-rich phase that can be reused directly as draw solution without further treatment. For energy estimation, we assume that the thermal energy needed in the phase separation process is provided by low grade waste heat or solar heat and high grade (electrical) energy is only required for the constant-pressure NF process. We take the needed constant NF hydraulic pressure of 6 bar (which is an overestimation, especially at high temperatures) as the osmotic pressure of the P4444DMBS water-rich phase into eqn (4). Based on this estimation, the minimum electrical energy required using the P4444DMBS draw solute in FO seawater desalination is 0.17 kWh m−3, only 16% of that needed for typical seawater RO which is 1.09 kWh m−3.2 We further estimated the thermal energy requirement based on the experimentally measured enthalpy of phase separation of the diluted draw solution (50% P4444DMBS, Fig. S8, ESI†). Only 1.63 kWh m−3 is required theoretically to affect the liquid–liquid phase separation. Hence the theoretical total energy needed, including the heat needed for phase separation plus the electricity needed for NF, is 1.80 kWh m−3. This value is higher than, but of the same order as the theoretical minimum of 1.09 kWh m−3. Details of the calculation are given in the ESI (page 8 in the ESI†). It is therefore believed that the utilization of thermal energy from low grade industrial waste heat (pages 7 and 8 in the ESI†) not only potentially lowers the energy cost of seawater desalination, but leads to reduced carbon footprint even though there might be an increase in the initial capital investment. While more detailed cost analysis of using such novel draw solutes needs to be conducted in the future in comparison with RO, it is important to mention that such responsive draw solutes also enable the treatment of high salinity feed streams. Such feed streams are difficult to treat using RO. Finally, it should be noted that if an NF membrane with a lower cut-off (MWCO 90 D) is used to polish water, the total organic carbon (TOC) in the product water is less than 20 ppm. This can be reduced to as low as 3 ppm when an RO membrane is used in place of the NF membrane. Also the new draw solutes are believed to be of very low toxicity (ESI†). We therefore believe that our new responsive IL draw solutes offer great promise to enable the proliferation of the current FO technology in desalination and water treatment.
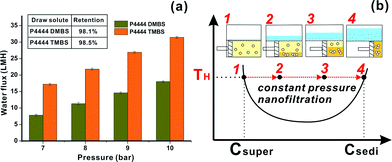 |
| Fig. 4 (a) Water flux during water recovery via low pressure NF. The retention rates of draw solutes after NF at 10 bar are tabulated in the inset. (b) Schematic depiction of further phase separation during low pressure NF which enables the constant pressure NF process even as the feed is being concentrated and water removed. | |
Conclusions
Thermally responsive ionic liquids are used as new draw solutes for FO desalination. These draw solutes can extract water from difficult feed streams such as brine with salinity as high as 1.6 M NaCl. At the same time, the diluted draw solutions have LCSTs below 50 °C above which they undergo liquid–liquid phase separation. The IL-rich sediment phase can be directly recycled as draw solution without further treatment, while the low concentration water-rich supernatant has an osmotic pressure of less than 6 bar. Water recovery is achievable via a low pressure NF process during which the osmotic pressure of the water-rich supernatant remains virtually constant because of the characteristic phase separation behaviour of these smart draw solutions. Therefore the estimated electrical energy consumption for water recovery is only a fraction of that for current RO seawater desalination. The rest of the energy needed can be in the form of low grade industrial waste heat or solar thermal energy. We envisage that these new draw solutes could motivate the proliferation of FO processes, pave the way towards low cost and low carbon footprint desalination, and enable the treatment of difficult industrial wastewater streams with high salinities via forward osmosis.
Acknowledgements
The authors would like to thank Nanyang Technological University for supporting this work. Two of the authors YFC and WMS would like to thank the University for providing PhD scholarships.
Notes and references
- P. Aldhous, Nature, 2003, 422, 251 CrossRef CAS PubMed.
- M. Elimelech and W. A. Phillip, Science, 2011, 333, 712 CrossRef CAS PubMed.
- M. F. A. Goosen, S. S. Sablani, H. Al-Hinai, S. Al-Obeidani, R. Al-Belushi and D. Jackson, Sep. Sci. Technol., 2005, 39, 2261 CrossRef PubMed.
- A. Bajpayee, T. Luo, A. Muto and G. Chen, Energy Environ. Sci., 2011, 4, 1672 CAS.
- K. N. Knust, D. Hlushkou, R. K. Anand, U. Tallarek and R. M. Crooks, Angew. Chem., Int. Ed., 2013, 52, 8107 CrossRef CAS PubMed.
- C. Klaysom, T. Y. Cath, T. Depuydt and I. F. J. Vankelecom, Chem. Soc. Rev., 2013, 42, 6959 RSC.
- B. Mi and M. Elimelech, J. Membr. Sci., 2010, 348, 337 CrossRef CAS PubMed.
-
R. Wang, L. Setiawan and A. Fane, Forward Osmosis: Current Status and Perspectives, J. Membr. Sci., 2012, Virtual Special Issue Search PubMed.
- S. Phuntsho, H. K. Shon, S. Hong, S. Lee, S. Vigneswaran and J. Kandasamy, Rev. Environ. Sci. Bio/Technol., 2011, 11, 147 CrossRef.
- L. A. Hoover, W. A. Phillip, A. Tiraferri, N. Y. Yip and M. Elimelech, Environ. Sci. Technol., 2011, 45, 9824 CrossRef CAS PubMed.
- J. Duan, E. Litwiller, S.-H. Choi and I. Pinnau, J. Membr. Sci., 2014, 453, 463 CrossRef CAS PubMed.
- C. Tan and H. Ng, Desalin. Water Treat., 2010, 13, 356 CrossRef CAS.
- M. L. Stone, A. D. Wilson, M. K. Harrup and F. F. Stewart, Desalination, 2013, 312, 130 CrossRef CAS PubMed.
- Q. Ge, J. Su, G. L. Amy and T. S. Chung, Water Res., 2012, 46, 1318 CrossRef CAS PubMed.
- R. E. Kravath and J. A. Davis, Desalination, 1975, 16, 151 CrossRef CAS.
- Q. Ge and T.-S. Chung, Chem. Commun., 2013, 49, 8471 RSC.
- Q. Zhao, N. Chen, D. Zhao and X. Lu, ACS Appl. Mater. Interfaces, 2013, 5, 11453 CAS.
- Z. Liu, H. Bai, J. Lee and D. D. Sun, Energy Environ. Sci., 2011, 4, 2582 CAS.
- Y. Cai, W. Shen, R. Wang, W. B. Krantz, A. G. Fane and X. Hu, Chem. Commun., 2013, 49, 8377 RSC.
- M. L. Stone, C. Rae, F. F. Stewart and A. D. Wilson, Desalination, 2013, 312, 124 CrossRef CAS PubMed.
- Y. C. Kim, S. Han and S. Hong, Water Sci. Technol., 2011, 64, 469 CrossRef CAS.
- Y. Cai, W. Shen, S. L. Loo, W. B. Krantz, R. Wang, A. G. Fane and X. Hu, Water Res., 2013, 47, 3773 CrossRef CAS PubMed.
- A. Razmjou, Q. Liu, G. P. Simon and H. Wang, Environ. Sci. Technol., 2013, 47, 13160 CrossRef CAS PubMed.
- A. Razmjou, M. R. Barati, G. P. Simon, K. Suzuki and H. Wang, Environ. Sci. Technol., 2013, 47, 6297 CrossRef CAS PubMed.
-
B. K. Jung, J. E. Kim, S. S. Han, H. Kang, H. R. Kang and J. Im HAN, US Pat. App. 13/845,471, 2013 Search PubMed.
- Y. Mok, D. Nakayama, M. Noh, S. Jang, T. Kim and Y. Lee, Phys. Chem. Chem. Phys., 2013, 15, 19510 RSC.
- D. Nakayama, Y. Mok, M. Noh, J. Park, S. Kang and Y. Lee, Phys. Chem. Chem. Phys., 2014, 16, 5319 RSC.
- J. R. McCutcheon, R. L. McGinnis and M. Elimelech, Desalination, 2005, 174, 1 CrossRef CAS PubMed.
- K. E. Gutowski, G. A. Broker, H. D. Willauer, J. G. Huddleston, R. P. Swatloski, J. D. Holbrey and R. D. Rogers, J. Am. Chem. Soc., 2003, 125, 6632 CrossRef CAS PubMed.
- K. Fukumoto and H. Ohno, Angew. Chem., Int. Ed., 2007, 46, 1852 CrossRef CAS PubMed.
- S. Saita, Y. Kohno and H. Ohno, Chem. Commun., 2013, 49, 93 RSC.
- J. Wei, C. Qiu, C. Y. Tang, R. Wang and A. G. Fane, J. Membr. Sci., 2011, 372, 292 CrossRef CAS PubMed.
- T. Cath, A. Childress and M. Elimelech, J. Membr. Sci., 2006, 281, 70 CrossRef CAS PubMed.
- J. R. McCutcheon, R. L. McGinnis and M. Elimelech, J. Membr. Sci., 2006, 278, 114 CrossRef CAS PubMed.
- L. Zhang, Z. Xu, Y. Wang and H. Li, J. Phys. Chem. B, 2008, 112, 6411 CrossRef CAS PubMed.
- A. Achilli, T. Y. Cath and A. E. Childress, J. Membr. Sci., 2010, 364, 233 CrossRef CAS PubMed.
- I. C. Sanchez, Polym. Blends, 1978, 1, 115 CAS.
- E. C. Cho, J. Lee and K. Cho, Macromolecules, 2003, 36, 9929 CrossRef CAS.
- H. O. Johansson, G. Karlstroem and F. Tjerneld, Macromolecules, 1993, 26, 4478 CrossRef CAS.
- P. Zheng, X. Hu, X. Zhao, L. Li, K. C. Tam and L. H. Gan, Macromol. Rapid Commun., 2004, 25, 678 CrossRef CAS.
- R. L. McGinnis and M. Elimelech, Desalination, 2007, 207, 370 CrossRef CAS PubMed.
- R. Semiat, Environ. Sci. Technol., 2008, 42, 8193 CrossRef CAS.
- G. Pearce, S. Talo, K. Chida, A. Basha and A. Gulamhusein, Desalination, 2004, 167, 175 CrossRef CAS PubMed.
Footnote |
† Electronic supplementary information (ESI) available: Water flux of P4448Br, P4444TMBS and P4444DMBS as the draw solute, osmolality prediction, back diffusion and viscosity of draw solutions, and FO economy and draw solute toxicity analyses. See DOI: 10.1039/c4ew00073k |
|
This journal is © The Royal Society of Chemistry 2015 |
Click here to see how this site uses Cookies. View our privacy policy here.