Oxidation behavior of zero-valent iron nanoparticles in mixed matrix water purification membranes
Received
8th October 2014
, Accepted 12th January 2015
First published on 23rd January 2015
Abstract
Morphological changes resulting from the oxidation of zero valent iron (ZVI) nanoparticles were measured as an assessment of their mechanical robustness in mixed matrix membranes for water treatment applications. Upon oxidation from metallic iron to iron oxide hydroxide, FeO(OH), particles underwent a significant transformation in size and morphology from 100 nm diameter spherical particles to plate-like crystalline particles with a hydrodynamic diameter greater than 450 nm. Atomic force microscopy (AFM) was used to mechanically degrade the FeO(OH) crystallites during repeated imaging. To determine whether similar degradation would occur during water filtration in a mixed matrix membrane, force under standard membrane operating conditions was calculated. Such force calculations were used to compare the shear forces exerted during water flux in a mixed matrix membrane to the normal forces imparted by AFM. Analysis suggested that the oxidized ZVI nanoparticles will experience a 10−19 N maximum shear force in pore channels, much lower than the imaging forces in AFM, suggesting the mechanical stability of the particles during water remediation. Additional quartz crystal microbalance experiments were performed to confirm the mechanical stability of the oxidized iron nanoparticles in the flow environments of ultrafiltration. Taken together, the results of this study demonstrate that the mechanical properties of the nanoparticle composite membranes are such that minimal mechanical degradation of the nanoparticles will occur during water filtration.
Water impact
Reactive nanoparticles can remove a wide variety of water contaminants, and a nanoparticle polymer composite membrane could serve as a reactive and physical barrier during water filtration, with the potential to improve overall contaminant removal. However, concerns arise in terms of being able to confidently immobilize nanoparticles in a filtration membrane with long-term resistance to nanoparticle leaching. Using quartz crystal microbalance, atomic force microscopy, and modeling techniques, we demonstrate that zero valent iron nanoparticles become oxidized after exposure to water yet remain mechanically robust at flow rates comparable to those used in filtration applications. Such analyses are necessary for nanoparticle bound water filtration membranes to ensure safety and long-term durability.
|
Introduction
Nanomaterials are being increasingly studied as components for next-generation drinking water purification systems, wherein high surface area atoms are used to adsorb and/or react with potential water contaminants.1–7 One promising nanomaterial is zero-valent iron (ZVI) nanoparticles, which have been previously studied as active systems for removal of contaminants from water due to their reactive properties.8–10 Oxidation of surface atoms in ZVI in aqueous environments produces radicals that can chemically breakdown drinking water pollutants. While the perception that surface oxidation of ZVI may limit the longer term use of these materials, studies focusing on the post-treatment of ZVI have demonstrated that the activity of ZVI can be renewed with post-treatment, making them attractive for long-term purification applications.11–13 For water treatment applications, ZVI nanoparticles are commonly immobilized in nanoparticle-enhanced membranes, or mixed matrix membranes, which is a novel method for immobilizing nanoparticles in a filtration membrane while simultaneously taking advantage of their reactive treatment capabilities. Mixed matrix membranes consist of a traditional ultra- or nanofiltration polymeric membrane in a composite with a second active component, in this case a reactive nanoparticle.14–17 The multifunctional nature of these membranes is attractive for water purification, as size exclusion purification and reactive contaminant remediation can be performed simultaneously.
Although ZVI nanoparticles are promising materials for removing a wide range of contaminants from drinking water, intact nanoparticles and ZVI degradation products could also become contaminants if not properly immobilized or filtered.18,19 In order for ZVI-enhanced water filtration technology to become commercially viable, nanoparticle-bound membranes must remain intact during filtration to ensure the production of safe drinking water. Moreover, the oxidation of ZVI leads to a change in volume and morphology of the nanoparticle that could affect the mechanical stability of the nanoparticle in the membrane. As such, it is important to understand the interplay between the oxidation behavior of the membrane bound ZVI and the corresponding changes in mechanical stability. In this paper, we examine the oxidation behavior of ZVI nanoparticles in multiple environments and draw conclusions regarding their viability for use in mixed matrix membranes from force measurement data.
Material and methods†
Materials
ZVI nanoparticles were synthesized by precipitation of ferrous sulfate with sodium borohydride in aqueous conditions and in the presence of the nanoparticle stabilizer carboxymethyl cellulose (molecular weight = 250
000 g mol−1, degree of substitution = 0.7) according to previously published techniques.20,21 To prevent oxidation, suspensions were then centrifuged to remove water, followed by resuspension of the ZVI nanoparticles in methanol. Suspensions were sonicated in a bath sonicator for 1 h at 95 W power prior to further analysis. Suspensions were measured with dynamic light scattering (DLS) to determine the average hydrodynamic diameter. In methanol, ZVI had an average agglomerate size of 184 nm ± 6 nm and a zeta potential of −20.6 mV ± 2.9 mV.
Mixed matrix membranes were cast from a suspension with a mass fraction of 15.0% polyethersulfone, 1.0% polyvinylpyrrolidone as pore former, and 0.1% ZVI nanoparticles in N,N-dimethylacetamide. Membranes were cast at room temperature to 75 μm thickness using doctor blading. They were then exposed to air for 30 s and immersed in a non-solvent deionized water bath for phase inversion. Membranes were washed with deionized water and stored in methanol to prevent iron oxidation before use.
Atomic Force Microscopy (AFM)
Samples were prepared for AFM characterization by spray coating one layer of ZVI nanoparticles onto a silicon substrate from a suspension of 1.0 mg mL−1 ZVI in methanol. Coated substrates were immersed in water, and then a time-resolved series of topographic maps was taken, on the same nanoparticles, in AFM tapping mode over a 30 h period. Measurements were done with a cantilever with a nominal spring constant of 3 N m−1. The free oscillation amplitude was ~50 nm and the setpoint oscillation amplitude was ~20 nm. To quantify the effect of oxidation on nanoparticle size, the height of a representative nanoparticle was recorded over the measurement period.
Quartz Crystal Microbalance (QCM)
Quartz crystal sensors were coated with ZVI nanoparticles to observe mass changes during oxidation. Coatings were produced by spraying a suspension of 1.0 mg mL−1 ZVI in methanol from an air gun pressurized with nitrogen to 207 kPa (30 psi); four layers were sprayed, and methanol was allowed to evaporate between each application. Dry baseline frequency measurements of the sensors were taken before and after coating, and these were used to calculate the starting mass of ZVI based on the Sauerbrey relationship.22 The change in frequency of the sensor, equated to the change in mass of ZVI, was measured in flowing deionized water at 50 μL min−1 and 500 μL min−1 for both 2 h and 24 h. Crystals were measured dry for 30 min to reach equilibrium; at this point, water was introduced to the chamber at the desired flow rate and held at this rate for the duration of the experiment. For all experiments, purified water was used with no background salts or other dissolved components; the pH of the purified water was between 6.5 and 7.0, and all experiments were run at 25 °C. As liquid was added, crystal sensors experienced a sharp and repeatable drop in the measured resonant frequency, due to damping from the liquid, which was subtracted from QCM data. A second drop in the measured resonant frequency of the sensor corresponded to the mass gain of ZVI nanoparticles due to oxidation. The change in mass was normalized to the initial mass of ZVI on the crystal.
Additional characterization
Scanning electron microscopy (SEM) was used to image the nanoparticles both before and after oxidation. Membrane samples were fractured in liquid nitrogen and sputtered with 5 nm gold for SEM imaging. These micrographs were analyzed with ImageJ.23 The average filtration velocity of water through the membranes was calculated from permeation experiments in which the mass of water passing through a 25 mm diameter membrane in a dead-end filtration cell was measured as a function of time at 69 kPa (10 psi) constant pressure. X-ray diffraction (XRD) was used to determine the crystalline phase of the oxidized ZVI nanoparticles.
Results and discussion
ZVI nanoparticles undergo oxidation in aqueous environments to FeO(OH), which causes size and morphological transformations of the nanoparticles (Fig. 1). From the SEM images in Fig. 1, the change from spherical iron particles (Fig. 1a) to tabular hydroxide is clearly visible (Fig. 1b). The change in size is confirmed by DLS (Fig. 1c), which shows that the particle size changed from a primarily monomodal broad distribution of 155 nm ± 18 nm average diameter particles to a multimodal distribution with peaks at 63 nm ± 5 nm, 353 nm ± 55 nm, and a portion of the particles are agglomerates greater than 4 μm in diameter. XRD results indicate that the original nanoparticles are metallic iron, likely a combination of both crystalline and amorphous phases, judging by the broadened peaks. As-synthesized ZVI nanoparticles also contain a magnetite component, likely a native oxide shell (Fig. 1d). Oxidized nanoparticles are primarily in the iron oxide hydroxide lepidocrocite phase. If a 100 nm spherical particle of metallic iron (density ρFe = 7870 kg m−3) was to fully oxidize to a spherical particle of lepidocrocite (ρFeO(OH) = 4000 kg m−3), the resulting diameter would be 146 nm. The large discrepancy between theoretical prediction and experimental DLS results is possibly due to the change in shape that occurs as iron particles oxidize to an orthorhombic plate-like crystal structure found in lepidocrocite. Because DLS assumes a spherical particle, plate-like oxide crystals are measured to have a much larger hydrodynamic diameter than spherical iron nanoparticles. DLS analysis can also overestimate the particle size because DLS measures the hydrodynamic diameter of the particles in solution and could include multiple solvation layers as well as particle agglomerates.
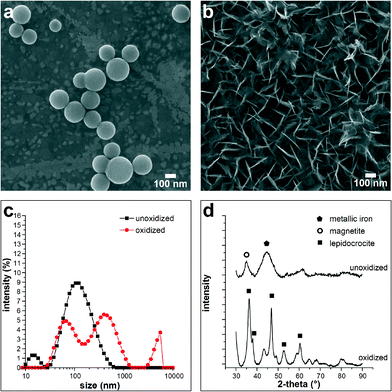 |
| Fig. 1 SEM micrograph showing (a) spherical ZVI nanoparticles and (b) the same nanoparticles after oxidation in water flowing at 500 μL min−1 for 2 h.24 (c) Measured particle size distributions confirm a shift in hydrodynamic diameter; the ZVI nanoparticles change from 155 nm ± 18 nm average diameter after synthesis to 353 nm ± 55 nm following 30 min exposure to water. (d) XRD analysis shows that metallic ZVI with a magnetite component oxidizes primarily to iron oxide hydroxide, FeO(OH), in the plate-like lepidocrocite crystal structure. | |
DLS results in methanol indicate zeta potentials of −2.3 mV ± 0.2 mV and −20.6 mV ± 2.9 mV for oxidized and metallic nanoparticles, respectively. The larger negative zeta potential of the metallic iron nanoparticles is largely due to the presence of the carboxymethyl cellulose stabilizer used during synthesis that remains adsorbed on the surface of the iron nanoparticles.21 During nanoparticle oxidation, it is likely that the stabilizer does not remain strongly adsorbed on the particle surface, particularly considering the large change in particle morphology that occurs. The lack of adsorbed stabilizer would likely cause a change in the zeta potential towards more positive values, as was observed experimentally. The zeta potential of the oxidized particles remains slightly negative at −2.3 mV ± 0.2 mV, and the slightly negative zeta potential is consistent with the presence of FeO(OH) as the primary oxidized iron phase, where the hydroxyl groups would impart a slightly negative surface charge. The reduced zeta potential after oxidation leads to a suspension that is much more susceptible to the formation of large aggregates, as was observed during DLS measurements of the solution and SEM imaging of the spray-coated samples.
Mass change during oxidation
Considering the notable change in size and shape directly observed in preliminary oxidation studies, real-time quantification of this transformation is needed. The oxidation of the ZVI nanoparticles in water also induces an increase in nanoparticle mass as metallic iron starts oxidizing. As such, QCM methods were used to monitor mass increases with ZVI oxidation over a 24 h period to capture this fast oxidation reaction. Note that QCM is an ideal method for observing mass changes in nanomaterials, as the technique is sensitive to mass changes on the order of ng cm−2. Initially two flow rates were tested with pure deionized water: 50 μL min−1 and 500 μL min−1 (Fig. 2). At the faster flow rate, oxidation reaches a peak rate of 0.530 ± 0.004 (g g−1) s−1 almost immediately. At slower flow, the onset of oxidation is delayed by approximately 5 min, at which point oxidation occurs at a peak rate of 0.256 ± 0.001 (g g−1) s−1, half the peak rate of that measured at 500 μL min−1. However, the slow flow rate appears to lead to more complete oxidation; the normalized change in mass Δm/mi would be 1.59, 2.86, and 4.15 for complete oxidation of Fe to FeO(OH), Fe2O3, and Fe3O4, respectively. We measured Δm/mi = 2.35 at 50 μL min−1 and Δm/mi = 1.88 at 500 μL min−1. This suggests that the slower flow rate leads to oxidation, at least partially, to one of the more thermodynamically stable oxide states.
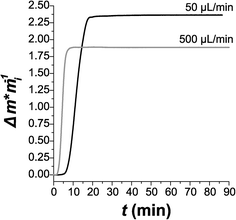 |
| Fig. 2 QCM results show the effect of flow rate on oxidation kinetics of ZVI nanoparticles. A slow flow rate (50 μL min−1 as compared to 500 μL min−1) delays the onset of oxidation and reduces the rate of oxidation by half, although the degree of oxidation is increased. | |
From the QCM results shown in Fig. 2, it is clear that one of the key challenges in using iron nanoparticles for water treatment is the short lifetime of the nanoparticles. Once the iron nanoparticles are oxidized, they are no longer reactive towards water contaminants, and the short lifetimes observed in Fig. 2 would not be practical for a real-world water treatment application. However, there are several potential strategies that might be used to extend the nanoparticle lifetime, including the addition of a second metal (e.g., nickel) as a protective but still reactive shell,24–26 the use of different stabilizer molecules to control surface reactivity,24 and the combination of iron nanoparticles with a material such as aluminum to resupply electrons to oxidized iron species for re-reduction.27,28
Post-oxidation mechanical degradation
To further examine the morphological changes in ZVI nanoparticles during oxidation and assess their mechanical properties, AFM was used to study the ZVI nanoparticles after exposure to pure water. ZVI nanoparticles were monitored over a period of 1800 min (30 h) (Fig. 3). In the period required to initiate AFM topography measurements, oxidation of ZVI nanoparticles to lepidocrocite had already occurred. Although we were unable to capture the increase in size associated with oxidation by the use of AFM, we did observe a significant decrease in the particle size over longer durations. Between 240 min and 1800 min, the height of the tracked particle decreases almost 60% from its original value (Fig. 3d). We postulate that, based on the images obtained from SEM, the platelet-like structure of the oxide is mechanically degraded by the forces exerted from tapping-mode AFM imaging. The observed damage is surprising, because tapping-mode AFM's normal forces are relatively small (maximum force ≈ 90 nN), and its shear forces are negligible. As such, the mechanical properties of these particles may not be suitable in high-flux environments of a membrane filtration system. If a nanoparticle does not remain intact and trapped in the membrane matrix, then the particle itself becomes a water contaminant. In addition, loss of iron precludes the possibility of regeneration of nanoparticles to prolong the service lifetime of the membrane.
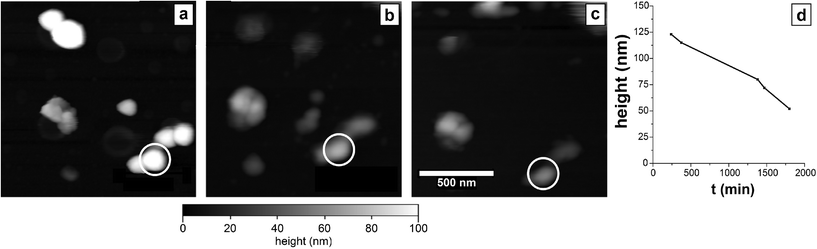 |
| Fig. 3 AFM topographs show changes in size of ZVI nanoparticles when exposed to high force tapping (force F ~ 90 nN) in water after (a) 240 min, (b) 1470 min, and (c) 1800 min. A summary of the change in height as a function of time for the encircled nanoparticle is shown in (d). This change in size was likely due to damage and removal of the oxide layer. | |
Nanoparticle durability in a composite membrane
To further assess the nanoparticle erosion behavior observed in AFM imaging, model membranes containing ZVI nanoparticles were prepared. As shown in the SEM micrograph in Fig. 4a, the membranes have a hierarchical pore structure similar to that found in neat polyethersulfone membranes. This indicates that the low particle loading used here does not substantially affect the membrane morphology. Although prepared from a nominally uniform suspension, iron nanoparticles are not found in the top skin layer but rather are incorporated into the pore walls of the membranes (Fig. 4b). The iron nanoparticles retain the morphology observed in Fig. 1a but are often covered with a layer of polyethersulfone polymer, as is observed in Fig. 4b; elemental analysis during SEM imaging was performed to confirm the presence of iron in the unoxidized and oxidized nanoparticles observed in Fig. 4b and c. After oxidation resulting from immersion of the membrane in pure water, crystallites have a plate-like morphology similar to that observed for oxidized free particles. Moreover, it appears that the particles remain largely intact even though there is a significant change in particle morphology as a result of oxidation, and the oxidized particles remain attached to membrane pore walls (Fig. 4c) after exposure to pure water.
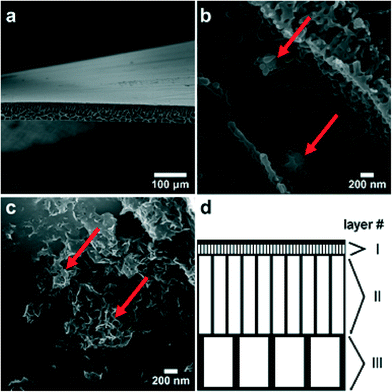 |
| Fig. 4 SEM images show (a) a cross section of the polyethersulfone membrane containing ZVI nanoparticles and high magnification views of (b) unoxidized ZVI nanoparticles and (c) oxidized ZVI nanoparticles in the polymer matrix (unoxidized and oxidized nanoparticles indicated by red arrows). (d) Schematic of the 3-layer hierarchical membrane pore structure as derived from SEM images that were used for force calculations. | |
The presence of the polymer and the interaction between the polymer and iron nanoparticles may enhance the mechanical stability of the nanoparticles and keep the oxidizing nanoparticles from migrating and growing into larger oxide particles, as was observed during QCM studies. Moreover, the nanoparticle concentration used in this study (0.1%) may not be the optimal nanoparticle concentration for nanoparticle–polymer composite membranes. It would be desirable to increase the nanoparticle loading in the membrane to achieve a higher removal of target water contaminants, but an increase in nanoparticle loading could also result in nanoparticle agglomeration. Another key challenge for nanoparticle-embedded filtration membranes is the difference between the nanoparticle lifetime (e.g., minutes to days or months) and membrane lifetime (e.g., typically five to 10 years or more). There are several possible strategies that could be used for nanoparticle regeneration, depending on the specific nanoparticle composition and membrane polymers used. In the case where iron nanoparticles are allowed to completely oxidize in a membrane, it would be possible to regenerate the iron nanoparticles through a series of acid and reducing agent rinses; the acid rinse would dissolve the iron oxides or hydroxides that form, and the reducing agent (e.g., sodium borohydride) would re-reduce the iron cations to iron metal and re-form the nanoparticles within the membrane. It is also possible to use strategies that involve the nanoparticle stabilizers and polymers in the membrane, where the stabilizer11 or additives to the membrane system such as polyacrylic acid12 can be used to extend the lifetime and prevent iron oxide precipitation. In these cases, the iron would still need to be re-reduced with a reducing agent, but oxide precipitates are largely prevented. Another possible strategy is to combine the iron with another material that would either extend the nanoparticle lifetime (e.g., nickel) or provide a source of electrons for re-reduction of iron cations (e.g., aluminum), as mentioned previously.24,27,28 In addition to extending the nanoparticle lifetime, further work on this system would necessarily include optimization of nanoparticle concentration and evaluation of the composite membranes for pure water flux performance and removal of one or more target water contaminants. Even with the material challenges of nanoparticle lifetime and optimization of the composite nanoparticle–polymer structure, this type of composite membrane does hold promise as a water treatment technology, and results from contaminant degradation studies suggest that immobilization of nanoparticles in a membrane matrix not only keeps the nanoparticles contained within the treatment system but may actually enhance contaminant removal due to differences in partitioning coefficients of water and contaminants (e.g., trichloroethylene) in the polymer matrix.29,30
Another potential challenge for nanoparticle-embedded membranes is the possibility that either whole nanoparticles or dissolved metals might leach from the membrane during water filtration. While previous work suggests that little to no metal leaches from membranes during water filtration,11 this result could be different depending on the nanoparticle–polymer systems used and would need to be tested for any material that is under development for use in a water treatment system. Based on our initial work, it seems unlikely that whole nanoparticles would become dislodged but that some leaching of iron is possible as the nanoparticles oxidize. To work towards determining if there is dissolved iron or nanoparticles leaching from our membranes, the filtrate from future pure water flux experiments would need to be analyzed for metal content.
Force calculations can be used to compare the shear forces exerted during water flux in a mixed matrix membrane to the normal forces imparted by AFM. The membrane porosity was analyzed with ImageJ, and a simplified pore structure was developed for force approximations. Three main layers of porosity are observed. The layer just beneath the top skin (Fig. 4d, layer I) contains pores with an average diameter davg = 0.65 μm ± 0.19 μm and length l = 2.4 μm ± 1.4 μm. Two additional support layers contain pores with davg = 3.2 μm ± 0.94 μm (layer II in Fig. 4d) and l = 20.6 μm ± 7.8 μm in the second layer and davg = 7.9 μm ± 1.3 μm and l = 13.2 μm ± 2.5 μm (layer III in Fig. 4d). Although the struts of the membrane are also porous on a much finer scale, these are considered to be solid in all approximations. The average calculated filtration velocity υ of water through the mixed matrix membranes is υ = 2.2 × 10−4 m s−1 ± 0.28 × 10−4 m s−1, as determined by permeation experiments. Assuming a constant pressure across the surface of the membrane and a uniform flow of water through each pore, the force exerted on an oxide platelet with an area A = 100 nm × 100 nm can be approximated from31
where
F is force,
P is pressure, and
Cd = 2 is the drag coefficient for a flat plate.
P = 1/2
ρυ2, where
ρ is the density of water. The maximum of
F corresponds to the smallest pore diameter, where the smallest pores at the top surface of the membrane control the water velocity through the membrane;
Fmax = 4.8 × 10
−19 N for
υ = 2.2 × 10
−4 m s
−1, which is considerably lower than the maximum forces estimated for AFM experiments. As such, oxidized FeO(OH) should be mechanically stable within a membrane material, and thus can be potentially used for water purification applications.
A comparison to the QCM results (Fig. 2) further indicates the mechanical robustness of these materials in filtration applications. Volumetric flow rates of 50 μL min−1 and 500 μL min−1 in a QCM chamber with dimensions 1.33 mm × 0.64 mm (A = 0.85 mm2) are used to calculate filtration velocity. Eqn (1) is then used to calculate the corresponding force on a hypothetical 100 nm2 oxide particle located at the sensor surface in the QCM chamber. This approximation yields F = 1.0 × 10−17 N and F = 1.0 × 10−15 N for 50 μL min−1 and 500 μL min−1, respectively. These values are two to four orders of magnitude higher than that estimated for an oxidized nanoparticle embedded in a membrane. However, after an initial rapid mass gain, a less than 1% change in mass is measured between 1 h and 24 h for either flow rate. This indicates that, for flow velocities typical for ultrafiltration membranes, ZVI nanoparticles are mechanically robust after oxidation which bodes well for the use of ZVI nanoparticles in mixed matrix membranes.
4. Conclusions
Zero-valent iron nanoparticles embedded in mixed matrix membranes for water purification were assessed for oxidation and mechanical robustness. Upon oxidation from metallic iron to FeO(OH), the particles undergo a significant transition in size and morphology, from spherical particles of 100 nm diameter to tabular crystallites with a hydrodynamic diameter greater than 450 nm. AFM measurements mechanically degrade these crystallites and decrease the particle size by up to 65% from the original size. While this finding may suggest that ZVI are not suitable for water purification applications, force approximations based on a single oxide platelet in a representative pore structure for a mixed matrix membrane suggest that the oxidized ZVI nanoparticles will be mechanically stable in the flow environments of ultrafiltration. This is further corroborated by QCM measurements. These results suggest that ZVI nanoparticles are mechanically suitable for future water purification applications.
Acknowledgements
The authors acknowledge Justin Shaw for his assistance with XRD measurements. This research was performed while J. D. Torrey and L. F. Greenlee held National Research Council Research Associateship Awards at the National Institute of Standards and Technology.
References
- T. Humplik, J. Lee, S. C. O'Hern, B. A. Fellman, M. A. Baig, S. F. Hassan, M. A. Atieh, F. Rahman, T. Laoui, R. Karnik and E. N. Wang, Nanostructured materials for water desalination, Nanotechnology, 2011, 22(29), 292001 CrossRef CAS PubMed.
-
N. F. Savage, Nanotechnology applications for clean water, W. Andrew, Norwich, N.Y., 2009 Search PubMed.
- J. Theron, J. A. Walker and T. E. Cloete, Nanotechnology and water treatment: Applications and emerging opportunities, Crit. Rev. Microbiol., 2008, 34(1), 43–69 CrossRef CAS PubMed.
- M. A. Shannon, P. W. Bohn, M. Elimelech, J. G. Georgiadis, B. J. Marinas and A. M. Mayes, Science and technology for water purification in the coming decades, Nature, 2008, 452(7185), 301–310 CrossRef CAS PubMed.
- J. Narr, T. Viraraghavan and Y. C. Jin, Applications of nanotechnology in water/wastewater treatment: A review, Fresenius Environ. Bull., 2007, 16(4), 320–329 CAS.
- N. Savage and M. S. Diallo, Nanomaterials and water purification: Opportunities and challenges, J. Nanopart. Res., 2005, 7(4–5), 331–342 CrossRef CAS.
- D. G. Rickerby, Nanotechnologies and nanoparticles in remediation and pollution prevention, Chim. Oggi, 2007, 25(6), 24–26 CAS.
- Z. Xiong, D. Zhao and G. Pan, Rapid and complete destruction of perchlorate in water and ion-exchange brine using stabilized zero-valent iron nanoparticles, Water Res., 2007, 41(15), 3497–3505 CrossRef CAS PubMed.
- M. Zhang, F. He, D. Zhao and X. Hao, Degradation of soil-sorbed trichloroethylene by stabilized zero valent iron nanoparticles: Effects of sorption, surfactants, and natural organic matter, Water Res., 2011, 45(7), 2401–2414 CrossRef CAS PubMed.
- Y.-P. Sun, X.-Q. Li, J. Cao, W.-X. Zhang and H. P. Wang, Characterization of zero-valent iron nanoparticles, Adv. Colloid Interface Sci., 2006, 120(1–3), 47–56 CrossRef CAS PubMed.
- V. Smuleac, R. Varma, S. Sikdar and D. Bhattacharyya, Green synthesis of Fe and Fe/Pd bimetallic nanoparticles in membranes for reductive degradation of chlorinated organics, J. Membr. Sci., 2011, 379(1–2), 131–137 CrossRef CAS PubMed.
- V. Smuleac, L. Bachas and D. Bhattacharyya, Aqueous-phase synthesis of PAA in PVDF membrane pores for nanoparticle synthesis and dichlorobiphenyl degradation, J. Membr. Sci., 2010, 346(2), 310–317 CrossRef CAS PubMed.
- B. W. Zhu and T. T. Lim, Catalytic reduction of Chlorobenzenes with Pd/Fe nanoparticles: reactive sites, catalyst stability, particle aging, and regeneration, Environ. Sci. Technol., 2007, 41(21), 7523–7529 CrossRef CAS.
- M. M. Pendergast and E. M. V. Hoek, A review of water treatment membrane nanotechnologies, Energy Environ. Sci., 2011, 4(6), 1946–1971 CAS.
- S. Mozia, Photocatalytic membrane reactors (PMRs) in water and wastewater treatment. A review, Sep. Purif. Technol., 2010, 73(2), 71–91 CrossRef CAS PubMed.
- J. Kim and B. Van der Bruggen, The use of nanoparticles in polymeric and ceramic membrane structures: Review of manufacturing procedures and performance improvement for water treatment, Environ. Pollut., 2010, 158(7), 2335–2349 CrossRef CAS PubMed.
- K. Zodrow, L. Brunet, S. Mahendra, D. Li, A. Zhang, Q. L. Li and P. J. J. Alvarez, Polysulfone ultrafiltration membranes impregnated with silver nanoparticles show improved biofouling resistance and virus removal, Water Res., 2009, 43(3), 715–723 CrossRef CAS PubMed.
- S. K. Brar, M. Verma, R. D. Tyagi and R. Y. Surampalli, Engineered nanoparticles in wastewater and wastewater sludge - Evidence and impacts, Waste Manage., 2010, 30(3), 504–520 CrossRef CAS PubMed.
- M. R. Wiesner, Responsible development of nanotechnologies for water and wastewater treatment, Water Sci. Technol., 2006, 53(3), 45–51 CrossRef CAS.
- F. He and D. Y. Zhao, Manipulating the size and dispersibility of zerovalent iron nanoparticles by use of carboxymethyl cellulose stabilizers, Environ. Sci. Technol., 2007, 41(17), 6216–6221 CrossRef CAS.
- L. F. Greenlee and S. A. Hooker, Development of stabilized zero valent iron nanoparticles, Desalin. Water Treat., 2012, 37, 114–121 CrossRef CAS.
- G. Sauerbrey, Verwendung von Schwingquarzen zur Wägung dünner Schichten und zur Mikrowägung, Z. Phys., 1959, 155(2), 206–222 CrossRef CAS.
- M. D. Abramoff, P. J. Magelhaes and S. J. Ram, Image Processing with ImageJ, Biophotonics Intern., 2004, 11(7), 36–42 Search PubMed.
- L. F. Greenlee, J. D. Torrey, R. L. Amaro and J. M. Shaw, Kinetics of zero valent iron nanoparticle oxidation in oxygenated water, Environ. Sci. Technol., 2012, 46(23), 12913–12920 CrossRef CAS PubMed.
- C. L. Chun, D. R. Baer, D. W. Matson, J. E. Amonette and R. L. Penn, Characterization and reactivity of iron nanoparticles prepared with added Cu, Pd, and Ni, Environ. Sci. Technol., 2010, 44(13), 5079–5085 CrossRef CAS PubMed.
- C. Lee and D. L. Sedlak, Enhanced formation of oxidants from bimetallic nickel-iron nanoparticles in the presence of oxygen, Environ. Sci. Technol., 2008, 42(22), 8528–8533 CrossRef CAS.
- L.-H. Chen, C.-C. Huang and H.-L. Lien, Bimetallic iron-aluminum particles for dechlorination of carbon tetrachloride, Chemosphere, 2008, 73, 692–697 CrossRef CAS PubMed.
- C.-C. Huang and H.-L. Lien, Trimetallic Pd/Fe/Al particles for catalytic dechlorination of chlorinated organic contaminants, Water Sci. Technol., 2010, 62(1), 202–208 CrossRef CAS PubMed.
- D. E. Meyer and D. Bhattacharyya, Impact of membrane immobilization on particle formation and trichloroethylene dechlorination for bimetallic Fe/Ni nanoparticles in cellulose acetate membranes, J. Phys. Chem. B, 2007, 111(25), 7142–7154 CrossRef CAS PubMed.
- D. E. Meyer, K. Wood, L. G. Bachas and D. Bhattacharyya, Degradation of chlorinated organics by membrane-immobilized nanosized metals, Environ. Prog., 2004, 23(3), 232–242 CrossRef CAS.
-
B. R. Munson, D. F. Young and T. H. Okiishi, Fundamentals of Fluid Mechanics, John Wiley & Sons, Inc., New York, 4th edn, 2002 Search PubMed.
Footnote |
† Commercial equipment, instruments, or materials are identified only in order to adequately specify certain procedures. In no case does such identification imply recommendation or endorsement by the National Institute of Standards and Technology, nor does it imply that the products identified are necessarily the best available for the purpose. |
|
This journal is © The Royal Society of Chemistry 2015 |
Click here to see how this site uses Cookies. View our privacy policy here.