Preparation and characterization of potassium nickel hexacyanoferrate-loaded hydrogel beads for the removal of cesium ions†
Received
24th July 2014
, Accepted 23rd October 2014
First published on 12th November 2014
Abstract
A novel synthetic approach has been used to prepare sorbent hydrogel composite beads of potassium nickel hexacyanoferrate (KNiHCF), using PVA and cross-linked alginate as the binding matrix. The characterization study indicates the high hydrophilicity and high surface area of the synthesized beads. The application potential of these beads for removal of cesium ions from low level liquid nuclear waste has been studied in batch mode using a 137Cs radiotracer. The equilibrium sorption and kinetic data are obtained at different initial cesium ion concentrations. These data are analysed using various sorption isotherm and kinetic models. It is observed that the sorption process can be best described by the Langmuir isotherm model, and the monolayer capacity of the beads is determined to be 7 mg per g of the swollen beads, which corresponds to ~64 mg per g of the dry beads. The sorption of cesium ions onto the sorbent beads is found to follow pseudo second-order kinetics over the entire studied concentration range. The ion-exchange mechanism involves the exchange of cesium ions with potassium ions. The mechanism of the sorption process is also investigated using the intraparticle diffusion model and Boyd's plot, and the results indicate that the sorption of cesium ions onto the beads is a complex process, involving both intraparticle diffusion and film diffusion.
Water impact
As the global trend for nuclear power and reprocessing continues, research is geared towards the removal and recovery of fission products from different nuclear waste streams. 137Cs is the most common fission product and is found in all types of nuclear waste streams. 137Cs is also considered as a major health concern in Fukushima. Many solutions have been proposed over the years for 137Cs removal, but all have their associated disadvantages such as low efficiency, gelatinous nature, secondary waste generation, inactive in alkaline/basic pH, etc. In this manuscript, we have demonstrated the synthesis and application of sorbent hydrogel beads with high sorption efficiency and selectivity towards cesium ions. This sorbent can be used over the entire pH range (1–12) for cesium removal. The characterization of the sorbent as well as the adsorption and kinetics behavior of these adsorbent beads has been also studied in detail.
|
1. Introduction
As the global trend for nuclear power and reprocessing continues, research is geared towards the removal and recovery of fission products from different nuclear waste streams. Most of the combined gamma activity in liquid nuclear waste is a result of the fission products 137Cs and 90Sr. 137Cs is an important radioactive contaminant, and with its long half-life and high mobility in aquatic environments, it poses a serious radiological hazard.1,2 For the past many decades, researchers worldwide have been working on developing efficient solutions towards the removal of radioactive cesium from various nuclear streams. As an outcome of the collective efforts, many methods have emerged for tackling this problem, with precipitation, solvent extraction, volatilization and inorganic ion exchange being the commonly used methods.3–8 Among these ion exchange methods, especially those which make use of various inorganic ion exchangers, such as aluminosilicates, phosphates, ferrocyanides and hydrous oxides of multivalent cations, pillared clays, etc., have been considered as viable options for removal of cesium.6,9–19
Inorganic ion exchangers are characterized by their selectivity, radiation and thermal stabilities and good compatibility with the matrices used for subsequent vitrification. Transition metal hexacyanoferrates are also one of the important inorganic ion exchangers which are currently being used in the nuclear industry for removal of cesium. These are generally used as precipitation agents, either by mixing the solutions of a metal salt and an alkali hexacyanoferrate directly into the waste solution to co-precipitate cesium ions or by first preparing the slurry of the metal hexacyanoferrate, and then mixing it with the waste solution to remove cesium. Both these methods involve a tedious procedure for separation of the co-precipitates from the waste solution. Another approach used is to prepare these sorbents in granular form and use them in column mode. However, the granules of the metal hexacynoferrates are of irregular shape and do not have good mechanical strength. During column operations, these granules wear out, and the fine powder generated causes choking of the column.19,20 Considering these facts, we have developed a new method for preparation of spherical potassium nickel hexacyanoferrate hydrogel beads by loading the pre-formed slurry of KNiHCF particles in a biopolymeric (alginate) matrix. These synthesized (KNiHCF-Gel) spherical beads have been characterized using TGA, XRD, optical microscopy, SEM and BET surface area analysis techniques. The applicability of these beads in the removal of cesium ions has been investigated in detail under various experimental conditions. The effect of pH, amount of cesium ions, and contact time on the sorption by the beads has been studied using a radioanalytical technique, and the results obtained are discussed.
2. Materials and methods
Sodium alginate and polyvinyl alcohol (molecular weight 125
000) were purchased from SD Fine Chemicals, India. Cesium nitrate, potassium hexacyanoferrate (KHCF), nickel sulphate, and nitrate salts of sodium, potassium, calcium and barium were obtained from Merck. All the chemicals used were of analytical grade. Water obtained from a Millipore-Q water purification system, with conductivity <0.3 μS cm−1, was used in all the experiments.
2.1 Synthesis of the KNiHCF-Gel beads
The KNiHCF-Gel beads used in this study were prepared indigenously, using sodium alginate as a binding matrix. KNiHCF particles were prepared by the reaction of NiSO4 (0.1 M) and KHCF (0.1 M) in 5
:
3 volume ratio, in the presence of 5 mL of 10% PVA solution. PVA acts as a stabilizer and controls the size of the KNiHCF particles formed. To this solution, 8 g of aqueous solution of 4% sodium alginate was added. This solution was used to prepare KNiHCF containing hydrogel composite beads, by dropping it into 4 wt% CaCl2 aqueous solution, through a 0.8 mm diameter syringe needle, using a syringe pump. Then, the formed beads were kept in the same CaCl2 solution for about 3 hours for complete curing by conversion of sodium alginate to calcium alginate. Then, the beads were separated from the solution and washed with water. These beads, in the water-swollen state, were used for the optical microscopy, TGA and extraction experiments, while for SEM, EDX, XRD, and BET surface area analysis, the dried beads were used.
2.2 Techniques
Optical microscope (OM) images of the synthesized KNiHCF-Gel beads were recorded on a Digital Blue QX5 computer microscope. Scanning electron microscopy (SEM) was employed to observe the microscopic morphology of the synthesized beads on a SERON Tech. AIS2100 SEM from South Korea, equipped with an energy-dispersive X-ray (EDX) spectrometer (model: INCA E350 from Oxford, UK). The samples were gold-coated before recording the SEM image and EDX spectra to prevent charging of the polymer. Thermogravimetry analyses (TGA) were performed on a Mettler Toledo TG/DSC STARe system at a heating rate of 10 °C min−1 under N2 atmosphere. The size of the KNiHCF particles was measured by the dynamic light scattering (DLS) method using a VASCOγ particle size analyzer at 25 °C (laser wavelength 658 nm). The Brunauer–Emmett–Teller (BET) surface area of the KNiHCF-Gel beads was determined by N2 adsorption–desorption measurement, using a Sorptomatic 1990 analyzer from CE Instruments, Italy. The samples were out-gassed at 100 °C under vacuum before the measurement. The gamma activity measurements were carried out using a well-type NaI (T1) detector connected to a single-channel analyzer, using the 661 keV photopeak of 137Cs.
2.3 Sorption studies
The 137Cs radiotracer was procured from the Board of Radiation and Isotope Technology (BRIT), Mumbai, India. The batch capacities of the sorbent were determined by equilibrating 0.05 g of the beads with 5 mL of the CsNO3 solution of appropriate concentration, spiked with a known amount of 137Cs (0.5 μCi L−1). The pH of the solution was adjusted by using dilute solutions of hydrochloric acid or sodium hydroxide. The solutions were agitated for a predetermined period at 20 ± 0.5 °C at a fixed speed of 150 rpm. All the sample solutions were equilibrated for 2 hours, maintaining the same sample-volume-to-sorbent-mass ratio for pH and sorption isotherm studies, and for kinetic studies, the samples were agitated at different time intervals (0–120 min). The effect of sorbent dose was examined by using six different masses of the sorbent in the range 0.01–0.2 g, keeping the volume of the sample solution constant (5 mL), with an initial cesium ion concentration of 50 mg L−1 at neutral pH. After equilibration, the sample aliquots were placed in counting vials and counted for gamma activity.
The equilibrium sorption capacity, qe, was calculated using the equation
| 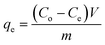 | (1) |
where
Co and
Ce are the initial and the equilibrium concentrations of cesium ions in solution, respectively,
V is the volume of the solution, and
m is the weight of the sorbent.
3. Results and discussion
Gelatinous green precipitates of KNiHCF were obtained by instantaneous reaction of KHCF with NiSO4 salt solution. To control the size of the KNiHCF particles, the reaction was carried out in the presence of PVA, which acts as a stabilizer. This slurry, containing KNiHCF particles stabilized by PVA, was then mixed homogenously with sodium alginate solution, which was then dropped into the aqueous bath containing CaCl2 through a syringe needle pump to form spherical beads. These gel beads contained uniformly distributed fine KNiHCF particles. Calcium ions in the aqueous bath cross-link with the α-1-guluronic acid (G) units of alginate. The binding of Ca2+ ions with the G units takes place in carboxyl groups (primary valence) and hydroxyl groups (secondary valence). The arrangement of the G units around calcium ions appears like an egg box; hence, the model is called an egg box model of the cross-linking of the alginate polymer.21 The beads formed by this procedure were quite dense, and the KNiHCF particles were embedded inside the spherical polymeric gel matrix.
3.1 Effect of PVA concentration
The stabilizing effect of PVA on the size of KNiHCF particles was studied by varying the concentration of PVA from 0 to 10 wt%, keeping the concentrations of KHCF and NiSO4 constant. The mean sizes of the KNiHCF particles, obtained by carrying out the reaction in the presence of different concentrations of PVA, were smaller than that obtained in the absence of PVA. It was observed that KNiHCF particles of mean size 1.4 μm were formed in the absence of PVA, whereas in the presence of 3% PVA, the mean size of the particle was reduced to 0.41 μm. Further increase in the concentration of PVA did not cause any significant reduction in the size of the KNiHCF particles in the studied range. However, 10 wt% solution of PVA was used in the synthesis of KNiHCF particles to maintain the optimum viscosity of the polymer slurry for efficient formation of the sorbent gel beads. The average size of the KNiHCF particles, under the conditions of the bead formation, was determined to be 0.4 μm. The hydration sphere associated with particles is generally responsible for the observed higher particle size by the dynamic light scattering technique as compared to that obtained by other techniques, such as AFM, SEM, TEM, etc. Thus, the actual size of the particles may be much smaller than 0.4 μm.
3.2 Characterization of the beads
The synthesized KNiHCF-Gel beads were characterized for their thermal stability, moisture content, surface morphology and surface area by using various techniques described subsequently.
3.2.1 SEM and EDX study.
The optical microscope (OM) image of the swollen KNiHCF-Gel beads is shown in Fig. 1(a). The OM image reveals that the beads are of spherical shape, with a relatively uniform size of ~2 mm. Fig. 1(b) and (c) show the SEM images of the outer surface and the cross-section of a KNiHCF-Gel bead, respectively. The outer surface of the dried bead is quite rough, and some large pores are visible in the SEM image. The cross-sectional image of the bead shows the presence of pores of even smaller size. The presence of KNiHCF particles in the synthesized beads was further confirmed by the EDX measurement.
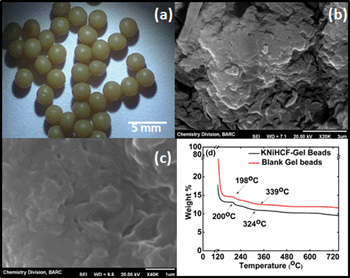 |
| Fig. 1 (a) OM image and SEM images of (b) the outer surface and (c) cross section of the KNiHCF-Gel beads and (d) TGA profiles of the blank alginate beads and the KNiHCF-Gel beads. | |
3.2.2 Thermogravimetry.
The thermal stability of the beads and their water content were determined by thermogravimetry. A known amount of the surface-dried swollen beads was heated in an alumina crucible at a heating rate of 10 °C min−1 under nitrogen atmosphere, from ambient conditions up to 750 °C. TGA profiles of the blank PVA–alginate gel beads and the KNiHCF-Gel beads are shown in Fig. 1(d). In the TGA profile of blank alginate beads, a huge weight loss is observed at 110 °C, which continues up to 150 °C, with a total weight loss of ~85%, due to the loss of the absorbed water molecules (Fig. S1(a), ESI†). A degradation step is observed from 198–339 °C, corresponding to a very small weight loss of 2%. After 339 °C, continuous degradation of the blank alginate beads takes place, and at the end of 750 °C, ~11.5% charred mass is left. The TG profile of KNiHCF-Gel beads is similar to that of the blank alginate beads. The first weight loss step, corresponding to the loss of the absorbed and structural water (~87%), is observed up to 150 °C, with maximum loss at 100 °C (Fig. S1(b), ESI†). Then, the second degradation step is observed from 200 to 324 °C. A shift of about +2 °C in the onset temperature and −15 °C in the offset temperature are observed in the TG profile of the sorbent beads, as compared to those of the blank beads. These shifts could be due to the presence of the KNiHCF particles in the polymeric matrix. At the end of 750 °C, ~9.5 % of the mass is left as a residue. From these results, it can be inferred that the sorbent beads are stable up to ~200 °C, and the moisture content (87%) of these beads is much higher than those of the sorbents reported in the literature, which are prepared by using conventional supports.22 The sorbent hydrogel beads synthesized in the present are expected to have faster kinetics for sorption of metal ions from aqueous waste solutions.
3.2.3 XRD and BET surface area analysis.
The X-ray powder diffraction pattern of the synthesized KNiHCF-Gel beads was recorded using CuKα radiation. The diffraction pattern obtained had broad diffraction peaks, indicating the amorphous nature of the KNiHCF particles (figure not shown here). The main diffraction peaks were observed at 2θ values of 15.19°, 17.56°, 25.05° and 35.49°, which match well with those reported for K2Ni[Fe(CN)6] (JCPDS card no. 20-0915). Thus, the results of the XRD study confirmed the presence of amorphous KNiHCF in the synthesized beads. The specific surface area and pore volume of the beads were determined by the BET N2 adsorption method. The nitrogen adsorption–desorption isotherms were measured at 77 K, at a relative partial pressure (i.e. P/Po) of N2 of 0.98. The surface area was determined to be 178.97 m2 g−1, and the pore volume was found to be 0.564 cc g−1. The surface area and pore volume measured are for the dried beads, but the actual extraction experiments were carried out using the swollen beads. Therefore, the effective surface area and pore volume of the swollen beads area expected to be much higher.
3.3 Sorption studies
KNiHCF has a cubic structure (FCC), in which iron and Ni ions are located at the corners of the elementary cubes and cyano groups are present on the edges. The exchangeable potassium ions are located in the body center. The lattice parameter ‘a’ of the unit cell is 1.012 nm for KNiHCF, which gives it ion sieving ability towards larger hydrated ions and is responsible for its selectivity for cesium ions.23 Therefore, the sorption of cesium ions onto the KNiHCF particles takes place by the ion-exchange mechanism, in which, generally, the potassium ion of KNiHCF is exchanged with a cesium ion. The synthesized sorbent beads being of hydrogel nature with high water content can freely exchange their water with that in the aqueous waste solution outside. Thus, it is expected that all the KNiHCF particles, even those present deep inside the beads, are accessible and contributing to the removal of cesium ions by the ion-exchange process. The sorption capacity of the blank polymer beads was determined at a cesium ion concentration of 5 mg L−1, while keeping the other conditions, such as the sorbent mass and sample volume, the same as those for the sorbent beads. The qe value for the blank polymer beads was determined to be 0.002 mg per g of the swollen beads. This capacity value is very low; hence, the sorption due to the polymeric binding matrix was considered to be negligible in all the sorption experiments. The synthesized beads were used to remove the cesium ions from the aqueous solution, and the effect of pH, sorbent dose, interfering ions and cesium ion concentrations on the sorption efficiency of the beads was investigated.
3.3.1 Effect of sorbent dose.
The effect of the sorbent dose on the percentage removal of cesium ions from the solution is shown in Fig. 2(a). A gradual increase in the percentage removal values is observed with increasing mass of the sorbent beads. Along with the increase of sorbent dosage, from 2 to 40 g L−1, the percentage of sorbed cesium ions increases from 30 to 99.9%. The increase in the percentage removal can be due to the fact that the high sorbent dosage offers more number of active sites and larger surface area for sorption of cesium ions. However, the determined sorption capacity (mg g−1) values for the various sorbents decreases with increasing sorbent dose (inset, Fig. 2(b)). The main reason for the decrease in the sorption capacity is the decrease in the fraction of occupied sites in the sorbent. The additional effect could be due to the interference between the binding sites at higher sorbent doses.24,25 Therefore, an optimum sorbent dose of 0.05 g per 5 ml sorbate solution, i.e. 10 g L−1, was chosen for the subsequent experiments.
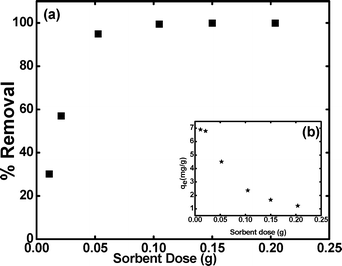 |
| Fig. 2 Effect of sorbent dose on the (a) %removal and (inset, (b)) sorption capacity of the KNiHCF-Gel beads for cesium ions. | |
3.3.2 Effect of pH.
The effect of pH on the equilibrium uptake capacity was studied by varying the pH of the sorbate solution using dilute NaOH and HNO3. It is observed that there is no significant change in the qe values in the pH range 1–12 (Fig. S2, ESI†). These results suggest that these beads can be used effectively over a wide pH range.
3.3.3 Effect of cesium ion concentration.
The effect of cesium ion concentration on the sorption capacity of the beads was studied by varying the initial concentration of cesium ions from 1 to 150 mg L−1 in neutral solution. Fig. 3(a) shows the effect of initial cesium ion concentration on the sorption capacity (qe). A gradual increase in the sorption capacity of the beads is observed up to cesium ion concentration of about 80 mg L−1, and thereafter, the qe value starts saturating. The qe value of 7.19 mg per g of the swollen beads is observed at Co of 150 mg L−1. However, the percentage uptake shows a decreasing trend, as the initial concentration of the cesium is increased (Fig. S3, ESI†). The maximum capacity, in terms of the dry weight of the sorbent, will be almost ten times more than the value obtained for the swollen beads (7.19 mg g−1), as these contain ~87% moisture. The initial cesium ion concentration plays an important role in the uptake of cesium by the beads by providing the necessary driving force to overcome all the mass resistances from the liquid to the solid phase. But when the concentration of cesium ions becomes very high, the competition between the cesium ions, for interacting with the active sites, also intensifies, and further uptake of cesium ions decreases due to the lower number of the vacant sites available on the sorbent beads.
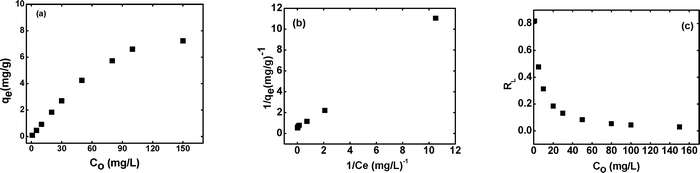 |
| Fig. 3 (a) Effect of initial cesium ion concentration (Co) on qe, (b) Langmuir sorption isotherm plot and (c) RLvs. Co plot for the sorption of cesium ions onto KNiHCF-Gel beads at 293 K. | |
3.4 Sorption isotherm studies
Application of the sorption isotherm models to the sorption data is helpful in describing how the solute interacts with the sorbent and hence helps in optimizing the conditions for the use of the sorbent. The experimental isotherm data were analyzed by Langmuir and Freundlich isotherms.26,27
3.4.1 Langmuir sorption isotherm.
The Langmuir sorption isotherm is the simplest isotherm model for sorption of a solute from a liquid solution onto a surface containing a finite number of identical active sites and assumes monolayer sorption.26 It is described by the following equation: | 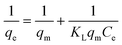 | (2) |
where qm (mg g−1) is the maximum capacity at complete monolayer coverage of the swollen beads, and KL is the Langmuir constant also known as the sorption coefficient. The plot of 1/qevs. 1/Ce is a straight line (Fig. 3(b)) with a correlation coefficient R2 of 0.999, which indicates a good correlation between the experimental data and the Langmuir sorption model. The values of qm and KL can be calculated from the intercept and the slope of the plot, respectively. The maximum monolayer sorption capacity of the beads for Cs+ ions is determined to be 6.86 mg per g of the swollen beads.
The favorability of sorption of Cs+ ions onto KNiHCF-Gel beads can also be expressed in terms of a dimensionless constant, (RL), called the separation factor, which is given below
The parameter RL indicates the nature of the shape of the isotherm. Depending on the value of RL, the sorption process is considered as unfavorable (RL > 1), favorable (0 < RL < 1), irreversible (RL = 0) or linear (RL = 1) sorption.
Fig. 3(c) depicts the plot of the calculated RL values at different initial cesium ion concentrations. All the RL values for the synthesized beads lie between 0 and 1, indicating that the sorption of cesium ions onto the beads is favorable for efficient removal of Cs+ ions from aqueous waste solutions under the studied conditions.28 However, a steep decrease in the RL values is observed with increasing initial concentration from 1 to 50 mg L−1. This indicates that the sorption of cesium ions onto the beads is more favourable at higher initial concentrations.
3.4.2 Freundlich isotherm.
The Freundlich isotherm is well known and is one of the oldest empirical sorption models. It assumes that all the active sites on the sorbent are not equal and the stronger binding sites are occupied first, and that the binding strength decreases with the increase in the degree of site occupation. The data were analyzed using the linearized form of the Freundlich isotherm given below:29 | 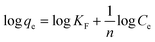 | (4) |
where KF is the Freundlich constant, which is related to the sorption capacity, and n is the Freundlich parameter, which is indicative of heterogeneity of the sorbent surface. The plot of log(qe) vs. log(Ce) is a straight line, and from the slope and the intercept of this straight line, the values of n and KF are determined (Fig. S4, ESI†). The correlation function value R2 for this plot is 0.918, suggesting a poor correlation between the experimental data and the Freundlich isotherm model, as compared to that with the Langmuir sorption model. The value of KF and n are determined to be 0.89 mg g−1 and 1.66, respectively.
3.5 Sorption kinetics
The sorption of metal ions onto the polymeric composites is a complex process, and the rate of uptake of the metal ions depends upon the physical and chemical properties of the sorbent. Therefore, it is necessary to understand the kinetics of sorption for selecting the optimum operating conditions for the full-scale batch process.30Fig. 4(a) shows the effect of contact time on the sorption of the cesium ions onto the synthesized beads at different initial cesium ion concentrations. It is observed that the equilibrium amount of cesium ions sorbed onto the beads increases with increasing initial cesium ion concentration. Most of the cesium ions are sorbed within the first 40 min. This could be due to the fact that after the initial fast sorption, the competition for the available active sites intensifies greatly, and the rate of sorption starts to decrease until equilibrium is reached. An increase in the rate of the sorption with increasing initial cesium ion concentration is observed, which could be due to driving force, provided by the higher concentration of cesium, to overcome the mass resistances, as explained earlier. The kinetic sorption data were analyzed using different kinetic models to investigate the rate law for sorption of cesium onto the beads.
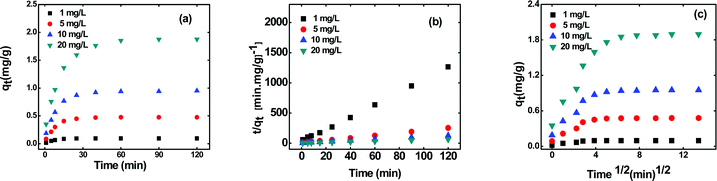 |
| Fig. 4 (a) Effect of contact time, (b) pseudo second-order kinetic and (c) intraparticle diffusion plots for sorption of cesium ions onto the KNiHCF-Gel beads at different initial cesium ion concentrations. | |
The pseudo first-order model and pseudo second-order kinetic model can be described by the following equations:31
|  | (5) |
|  | (6) |
where
qt is the amount of the cesium ions adsorbed at time
t, and
k1 and
k2 are the pseudo first-order and pseudo second-order rate constants, respectively. After integration and applying the boundary conditions, at
t = 0,
qt = 0, and the linearized forms of the two models can be written as follows:
|  | (7) |
|  | (8) |
The values of k1 and qe for pseudo first-order rate kinetics can be determined from the slope and intercept, respectively, of the straight line plot of log(qe − qt) versus t (Fig. S5 and Table S1, ESI†). Similarly, the pseudo second-order rate constants k2 and qe can be determined from the t/qtvs. t plot (Fig. 4b). The values of k2 and the corresponding correlation coefficients (R2), along with the theoretically determined values of qe, from the pseudo second-order rate model, are given in Table 1. The R2 values for the pseudo first-order model are quite small as compared to those for the pseudo second-order rate model. Moreover, the equilibrium capacities, qe, obtained from the pseudo second-order rate model closely match with the experimental values (Table 1). Therefore, it can be concluded that the sorption of cesium ions onto the sorbent beads follows pseudo second-order kinetics for the entire time period and for all the studied initial concentrations.
Table 1 The pseudo second-order rate constants and the theoretical and experimental qe values determined for the removal of cesium by the KNiHCF-Gel beads at different initial concentrations of cesium
Pseudo-2nd order kinetic model |
C
o (mg L−1) |
k
2 (mg min−1) |
R
2
|
q
e (theoretical) (mg g−1) |
q
e (experimental) (mg g−1) |
1 |
3.572 |
0.999 |
0.097 |
0.095 |
5 |
0.539 |
0.997 |
0.490 |
0.477 |
10 |
0.238 |
0.997 |
0.987 |
0.950 |
20 |
0.083 |
0.998 |
1.974 |
1.896 |
3.6 Sorption mechanism
The transport of the sorbate from the solution phase to the sorbent particles involves many steps.32 These steps include external or film diffusion and intraparticle diffusion. To determine the mechanistic steps involved in the present sorption system, the kinetic data were analyzed using the intraparticle diffusion model given by Weber–Morris, which is expressed aswhere Kid (mg g−1 min−1/2) is the intraparticle diffusion parameter, and I gives an idea about the boundary layer thickness.33
According to this model, the plot of qtversus the square root of time (t1/2) should be linear if intraparticle diffusion is involved in the sorption process, and if this line passes through the origin, then the intraparticle diffusion is the only rate-limiting step. From Fig. 4(c), it can be seen that the plot of qtvs. t1/2 is multilinear, with a sharp slope for the initial fast sorption stage and a gentle slope for the subsequent slower sorption stage at later time. The values of Kid and I can be determined from the slope and intercept, respectively, of the second stage (Table S1, ESI†). The results from this model imply that the intraparticle diffusion is also involved in the sorption of cesium ions onto the beads, but it is not the only rate-governing step. Therefore, in order to further investigate the steps involved in the sorption process, the sorption data were also analyzed by the kinetic expression given by Boyd,34
| 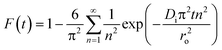 | (10) |
| 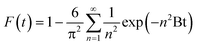 | (11) |
| 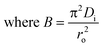 | (12) |
and
F is the fractional attainment of equilibrium,
i.e., the ratio of the solute sorbed at time t to that at equilibrium (
F =
qt/
qe),
Di is the effective diffusion coefficient of the metal ion,
ro is the radius of the sorbent and
n is an integer.
Solutions to eqn (11), depending on the value of F, are given as eqn (13) and eqn (14).35
| 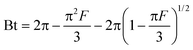 | (13) |
| Bt = −0.4977 − ln(1 − F) | (14) |
Thus, the value of Bt can be calculated for each value of F, using eqn (13) for F values up to 0.85 and eqn (14) for higher F values.35 The calculated Bt values were plotted against time (Fig. S6, ESI†). The linearity of this plot provides useful information to distinguish between the film diffusion and the intraparticle diffusion rates of sorption.32 A straight line passing through the origin is indicative of sorption processes governed only by the intraparticle diffusion mechanism; otherwise, it is governed by film diffusion.36 In the present case, the plots are scattered and do not pass through the origin, indicating the complex nature of the sorption process and film diffusion as the rate-determining step.
3.7 Effect of interfering ions
The synthesized beads can be used for the removal of cesium ions from low level liquid waste originating at different points during the nuclear fuel cycle. One such source is the cooling pond water, which is among the largest waste streams in terms of the volume of liquid. Cooling pond water is used to cool and shield irradiated uranium fuel rods prior to their disposal or reprocessing. During their storage, small amounts of 137Cs and 90Sr are released from the fuel rods into the cooling water. For safe discharge of the pond water in the environment, removal of radioactivity is essential. The contaminated fuel pond water typically has a pH of about 8.2 and contains cesium (~6 × 10−5 ppm) along with interfering ions such as calcium (~33 ppm), magnesium (~0.7 ppm), sodium (~37 ppm), potassium (~2.5 ppm) and barium (~3 ppm).37 The sorption of cesium by the KNiHCF sorbent mainly proceeds through exchange of potassium ions with cesium ions.38 Therefore, it is necessary to study the effect of the presence of other ions on the efficiency of the synthesized beads for removal of cesium ions. The influence of the interfering cations (Na+, Ca2+ and Ba2+) on the sorption of cesium ions onto the KNiHCF-Gel beads was investigated, keeping the concentration of cesium ions constant (5 mg L−1) and varying the concentration of Na+, Ca2+ and Ba2+ ions under neutral pH at 298 K. On increasing concentration of the metal ions from 0 to 32 mg L−1, there is almost insignificant change in the percentage removal value and sorption capacity of the beads for cesium ions in the presence of Na+, Ca2+ and Ba2+ ions (Table S2, ESI†). This suggests that the synthesized beads have high selectivity for cesium ions in the presence of the interfering ions. The high selectivity of the sorbent could be due to the smaller hydration radius of the cesium ions as compared to that of the other interfering ions studied. These results indicate that the synthesized sorbent beads can be used effectively for removal of cesium ions in the presence of the interfering metal ions.
Conclusions
Potassium nickel hexacyanoferrate (KNiHCF) was loaded on a composite matrix of PVA and sodium alginate to form spherical sorbent beads. The equilibrium adsorption studies show that the synthesized beads have good potential as a sorbent for the removal of radioactive cesium ions from low-level liquid nuclear waste in the pH range 1–12. The monolayer capacity determined by applying the Langmuir adsorption isotherm is ~64 mg per g of the dry beads. The kinetics of sorption of the cesium ions onto the synthesized beads is quite fast, and the sorption process reaches equilibration in the first 60 minutes. Among the different kinetic equations applied, the kinetics data are represented well by the pseudo second-order rate model. Analysis of the mechanistic steps involved in the sorption process reveals the complex nature of the sorption process, which consists of both film diffusion and surface adsorption. The sorbent beads have shown good selectivity for cesium ions even in the presence of high concentration of interfering metal ions. These results show that the synthesized beads have good potential for use in cesium removal from low level radioactive waste.
Acknowledgements
The author, Charu Dwivedi, is grateful to BRNS, Department of Atomic Energy, for awarding a research fellowship. The authors are thankful to Mr. J. Nuwad and Dr. C.G.S. Pillai for SEM experiments and MR. T. V. V. Rao, Dr. K. T. Pillai and Dr. S. K. Mukerjee for BET surface area measurements. The authors also wish to acknowledge Dr. T. Mukherjee and Dr. S.K. Sarkar for their encouragement during the course of the study.
References
- B. J. Howard, N. A. Beresford and K. Hove, Health Phys., 1991, 61, 715–725 CrossRef CAS.
- W. L. Robison and E. L. Stone, Health Phys., 1992, 62, 496–511 CrossRef CAS.
- J. D. Law, R. S. Herbst and T. A. Todd, Sep. Sci. Technol., 2002, 37, 1353–1373 CrossRef CAS PubMed.
- M. Adabbo, D. Caputo, B. De Gennaro, M. Pansini and C. Colella, Microporous Mesoporous Mater., 1999, 28, 315–324 CrossRef CAS.
- S. Komarneni and R. Roy, Nature, 1982, 299, 707–708 CrossRef CAS.
- S. Komarneni and R. Roy, Science, 1988, 239, 1286–1288 CAS.
- T. Tsuda, C. L. Hussey, H. Luo and S. Dai, J. Electrochem. Soc., 2006, 153, D171–D176 CrossRef CAS PubMed.
- C. Xu, L. Yuan, X. Shen and M. Zhai, Dalton Trans., 2010, 39, 3897–3902 RSC.
- M. Panagiotis, Microporous Mesoporous Mater., 2011, 144, 15–18 CrossRef PubMed.
- K. Shakir, M. Sohsah and M. Soliman, Sep. Purif. Technol., 2007, 54, 373–381 CrossRef CAS PubMed.
- J. B. Ayers and W. H. Waggoner, J. Inorg. Nucl. Chem., 1971, 33, 721–733 CrossRef CAS.
- H. Buchwald and W. P. Thistlethwaite, J. Inorg. Nucl. Chem., 1958, 5, 341–343 CrossRef CAS.
- J. Doležal, J. Stejskal, M. Tympl and V. Kouřím, J. Radioanal. Chem., 1974, 21, 381–387 CrossRef.
- J. Krtil, J. Inorg. Nucl. Chem., 1962, 24, 1139–1144 CrossRef CAS.
- V. Pekárek and V. Veselý, Talanta, 1972, 19, 1245–1283 CrossRef.
- P. Sylvester and A. Clearfield, Solvent Extr. Ion Exch., 1998, 16, 1527–1539 CrossRef CAS.
- M. M. Abd El-Latif and M. F. Elkady, Desalination, 2010, 255, 21–43 CrossRef CAS PubMed.
- T. Kodama, T. Higuchi, T. Shimizu, K. I. Shimizu, S. Komarneni, W. Hoffbauer and H. Schneider, J. Mater. Chem., 2001, 11, 2072–2077 RSC.
- S. Taj, D. Muhammad, M. A. Chaudhry and M. Mazhar, J. Radioanal. Nucl. Chem., 2011, 288, 79–88 CrossRef CAS.
- N. M. Hassan and K. Aduvu Wusu, Solvent Extr. Ion Exch., 2005, 23, 375–389 CrossRef CAS PubMed.
- M. Alnaief, M. A. Alzaitoun, C. A. García-González and I. Smirnova, Carbohydr. Polym., 2011, 84, 1011–1018 CrossRef CAS PubMed.
- A. Nilchi, R. Saberi, M. Moradi, H. Azizpour and R. Zarghami, Chem. Eng. J., 2011, 172, 572–580 CrossRef CAS PubMed.
- H. Mimura, J. Lehto and R. Harjula, J. Nucl. Sci. Technol., 1997, 34, 484–489 CrossRef CAS.
- B. Benguella and H. Benaissa, Water Res., 2002, 36, 2463–2474 CrossRef CAS.
- M. N. Zafar, R. Nadeem and M. A. Hanif, J. Hazard. Mater., 2007, 143, 478–485 CrossRef CAS PubMed.
- K. R. Hall, L. C. Eagleton, A. Acrivos and T. Vermeulen, Ind. Eng. Chem. Fundam., 1966, 5, 212–223 CAS.
- P. Saha, S. Chowdhury, S. Gupta and I. Kumar, Chem. Eng. J., 2010, 165, 874–882 CrossRef CAS PubMed.
- I. A. W. Tan, A. L. Ahmad and B. H. Hameed, J. Hazard. Mater., 2009, 164, 473–482 CrossRef CAS PubMed.
- D. Das, N. Das and L. Mathew, J. Hazard. Mater., 2010, 184, 765–774 CrossRef CAS PubMed.
- V. K. Gupta and S. Sharma, Ind. Eng. Chem. Res., 2003, 42, 6619–6624 CrossRef CAS.
- A. Saeed, M. Sharif and M. Iqbal, J. Hazard. Mater., 2010, 179, 564–572 CrossRef CAS PubMed.
- B. K. Singh and N. S. Rawat, J. Chem. Technol. Biotechnol., 1994, 61, 57–65 CrossRef CAS.
- F.-C. Wu, R.-L. Tseng and R.-S. Juang, Chem. Eng. J., 2009, 150, 366–373 CrossRef CAS PubMed.
- G. E. Boyd, A. W. Adamson and L. S. Myers Jr, J. Am. Chem. Soc., 1947, 69, 2836–2848 CrossRef CAS.
- D. Reichenberg, J. Am. Chem. Soc., 1953, 75, 589–597 CrossRef CAS.
- M. Sankar, G. Sekaran, S. Sadulla and T. Ramasami, J. Chem. Technol. Biotechnol., 1999, 74, 337–344 CrossRef CAS.
-
P. Sylvester, Encyclopedia of Separation Science, 2000, vol. 9, pp. 4261–4267 Search PubMed.
- L. Vrtoch, M. Pipíška, M. Horník, J. Augustín and J. Lesný, J. Radioanal. Nucl. Chem., 2011, 287, 853–862 CrossRef CAS PubMed.
Footnote |
† Electronic supplementary information (ESI) available: TGA-DTG curves of blank and KNiHCF-Gel beads; effect of pH on the sorption capacity of the beads; effect of initial cesium ion concentration on its sorption onto the KNiHCF-Gel beads; Freundlich sorption isotherm plot; pseudo first-order kinetic plots and Boyd's plots for sorption of cesium ions onto the KNiHCF-Gel beads at different initial cesium ions; pseudo first-order and intraparticle diffusion model parameters for sorption of cesium ions onto the KNiHCF-Gel beads at different initial concentrations; and effect of the interfering ion concentration on the sorption of cesium ions onto the KNiHCF-Gel beads. See DOI: 10.1039/c4ew00021h |
|
This journal is © The Royal Society of Chemistry 2015 |