Mitochondrial energy metabolism in the hepatopancreas of freshwater crabs (Sinopotamon henanense) after cadmium exposure
Received
21st August 2014
, Accepted 9th October 2014
First published on 10th October 2014
Abstract
Energetic homoeostasis is a fundamental requirement in the defense against cadmium (Cd) stress. Using the freshwater crab Sinopotamon henanense as an example, we explored the effects of Cd on submicroscopic structures in hepatopancreatic cells through transmission electron microscopy (TEM) and energy-related parameters such as adenosine triphosphate/adenosine diphosphate (ATP/ADP) ratios, reduced/oxidized nicotinamide adenine dinucleotide (NADH/NAD+) ratios, and the mitochondrial membrane potential (Δψm). The impact of Cd on carbohydrate and protein metabolism, and metallothionein (MT) was also investigated. Experimental 10 day exposure increased NADH and NAD+ and provided a higher NADH/NAD+ ratio and Δψm in hepatopancreatic cells. The corresponding significantly increased levels of ATP and the ATP/ADP ratio in the hepatopancreas supported high energy production. The up-regulation of the MT content in exposed crabs suggests that protein synthesis for detoxification could partially be a major ATP consumer. With increasing exposure time, however, energy production was in decline. Excessive energy consumption was explained by substrate mobilization and mitochondrial impairment. Less carbohydrate and enhanced protein catabolism was observed. Ultrastructurally, there were changes in mitochondria with swelling, membrane disruption, shortening of cristae or the rupture and disappearance of entire mitochondria. The rough endoplasmic reticulum (rER) displayed expansion and membrane rupture, suggesting the destruction of protein-synthesizing structures in hepatopancreatic cells. Our findings suggest that energy-related parameters could be used as biomarkers in the monitoring of metal pollution and quantitative risk assessments of pollutant exposure.
Environmental impact
The intoxication of organisms and a variety of adverse effects from Cd is becoming a global environmental problem that damages aquatic organisms and aquatic ecosystems. It is necessary to develop reasonable biological markers from representative species to indicate environmental pollution in aquatic systems. Therefore, the main objective of the present study was to examine the effects of Cd on energy metabolism, carbohydrates, protein metabolism, and intracellular antioxidants. Another purpose was to provide quantitative risk assessments of potentially deleterious environmental exposure in comparison with routinely used biomarkers in biomonitoring programs by evaluation of these stress parameters.
|
Introduction
Over the last few years, cadmium (Cd) contamination in the aquatic environment has increased, mainly due to human industrial activities such as burning of coal in power stations and metal smelting (Jozef et al., 2009; Pirrone et al., 2010; Ciliberti et al., 2011).1–3 Cd pollution incidents are frequently found in aquatic systems (Bai et al., 2012; Feng et al., 2010; Lin et al., 2002).4–6 Some examples from China are Shaoguan city of Guangdong North River in 2005 and the Longjiang Cd pollution event in Hechi, Guangxi, 2012 (Zhou et al., 2012).7 The Cd level could reach 12.05 ± 1.47 mg l−1 in some valleys near Cd-rich mines (Yuan et al., 2010).8 Cd pollution will have a negative impact on the fitness and survival of aquatic organisms as indicated by a decrease in biodiversity of polluted areas. A decline and extinction of some species has been reported (Han et al., 2008).9 Cd exposure could stimulate the formation of reactive oxygen species (ROS) such as hydroxyl radicals and singlet oxygen. ROS could cause oxidative stress by reacting with macromolecules, leading to membrane lipid peroxidation (Pan and Zhang, 2006).10 The cellular mechanisms of toxicity, such as Cd-induced cell necrosis (Gennari et al., 2003) and apoptosis (Liu et al., 2011),11,12 have been intensively studied. In addition, Cd is also reported to affect lipid and protein metabolism in fish (De and Blust, 2001; Pierron et al., 2007)13,14 and birds (Lucia et al., 2010).15
The acute or chronic intoxication of organisms and a variety of adverse effects from Cd is becoming a global environmental problem that damages aquatic organisms and aquatic ecosystems (Sousa et al., 2008; Moreirinha et al., 2011).16,17 Therefore, it is necessary to develop reasonable biological markers from representative species to indicate environmental pollution in aquatic systems. Biomarkers are useful as indicators of biological states, and as indicators of biological processes, pathogenic processes, responses to stressors, and the monitoring of environmental quality (McCarthy and Shugart, 1990).18 In the monitoring of aquatic systems several different organisms were used as model organisms for environmental pollution studies (Nogueira et al., 2010; Oliveira et al., 2011).19,20 Among them, crustaceans are considered as suitable indicators because of their sediment-associated and less mobile life styles. Some crustacean species, such as crayfish Astacus leptodactylus (Malev et al., 2010)21 and the marine crab Carcinus maenas (Ben-Khedher et al., 2013),22 were chosen for the study of aquatic contamination. In the present study, the freshwater crab Sinopotamon henanense became the subject of our molecular approaches. This sentinel crab species is widely distributed in the Yangtze River drainage, Huaihe River drainage and Yellow River Valley of China. It lives at the interface of sediments and the water column where Cd is deposited (Zhao et al., 2012).23 The hepatopancreas of S. henanense is involved in a variety of metabolic and secretory functions, such as lipid storage and synthesis, and food digestion. It also has the ability to synthesize antioxidants and plays a major role in the detoxification of toxic compounds. Therefore, the hepatopancreas of crabs could be used as a model organ to explore novel molecular mechanisms involved in the reaction to xenobiotics.
Previous laboratory studies focussed on Cd effects on the antioxidant system comprising enzymes, such as the cellular activity of superoxide dismutase (SOD), glutathione peroxidase (GSH-Px), catalase (CAT), malonyldialdehyde (MAD), and metallothionein (MT). These antioxidant enzymes could also be used as routine biomarkers (Ma et al., 2007; Li et al., 2008; Wang et al., 2008).24–26 Recently, more studies have focused on another group of biomarkers derived from energy parameters provided by the mitochondrial energy metabolism (Padmini and Usha, 2011; Sokolova et al., 2012).27,28 They are considered to be more sensitive to contaminant exposure compared to routinely used antioxidant and oxidant stress parameters in biomonitoring programs. For the energy metabolism of eucaryotes, mitochondrial respiration and ATP synthesis represent two most important pathways. Actually, mitochondrial respiration is the oxidation of mitochondrial NADH. Protons coupled with NAD+ are pumped across the mitochondrial inner membrane, generating an electrochemical gradient of protons (Δp) by the electron transport chain which leads to a membrane potential Δψm. Via mitochondrial ATP synthase, these protons are returning to the mitochondrial matrix across the membrane where they also generate ATP. The levels of NADH and Δψm are, therefore, critical for ATP synthesis. Moreover, the main substrate for the respiratory chain is supplied by fatty acids, glucose, and amino acids through interconnected pathways. The products of ATP synthesis are used by a large number of cellular pathways. The most important process may be protein synthesis, which also provides enzymes for the defence of xenobiotics such as antioxidant enzymes, and metallothioneins (Brown, 1992; Rolfe and Brown, 1997).29,30
In the present study, the ratios of ATP/ADP, NADH/NAD+, and Δψm were measured, and mitochondrial submicroscopic structures of hepatopancreatic cells were studied by TEM. The main objective of the present study was to examine the effects of Cd on energy metabolism. Another purpose was to explore the possible effects of Cd on carbohydrates, protein metabolism, and intracellular antioxidants.
Materials and methods
Ethics statement
The place where crabs were caught is privately owned. Our study was carried out with the permission from the landowner. We also confirm that the current study did not involve endangered or protected species. The work described in this article was performed in accordance with National and Institutional guidelines for the protection of animal welfare.
Treatments and sample preparation
Freshwater crabs, S. henanense, were purchased from Taiyuan Wulongkou fish market. These crabs were known to be collected from the mostly unpolluted Qinghe River near Qingyan, Henan province in China. Before the experiment, mature female crabs of comparable weight were acclimated for 3 weeks in glass aquaria (45 cm × 30 cm × 30 cm) that were filled with dechlorinated tap water that was filtered through charcoal (dissolved oxygen 8.0–8.3 mg l−1, pH 7.5 ± 0.13, and temperature 20 ± 2 °C). The aquaria were shielded using a black plastic to reduce optical disturbance. After acclimation, crabs were randomly divided into a control group and three treatment groups. Crabs in each group were also placed in five different tanks with 20 individuals each.
Crabs were exposed to Cd in the water using a static renewal method. They were exposed to 1.45, 2.9, and 5.8 mg l−1 of CdCl2 (Sailboats Chemical Reagent Co., Ltd., Tianjin, China), corresponding to 1/160, 1/80, 1/40 of the 96 h LC50 according to Wang et al. (2008) for 10, 15 and 20 days. These concentrations were similar to the Cd levels in a few serious pollution incidents. Water was renewed to clean tanks every two days with treatment batches of the same Cd concentration. During the acclimation and exposure period, crabs were fed a commercial feed (Hanye, Beijing, China), containing 30% protein and 15% fat every evening (5% animal wet weight per day). Tissues were sampled after 10, 15 and 20 days. After heparin (400 units per kg body weight) was injected into the abdomen, a haemolymph sample of 0.2 ml was taken 5 min later, centrifuged to collect serum, and frozen temporarily at −20 °C for the subsequent measurement of glucose. After dissection of the cephalothorax, the hepatopancreas was immediately sampled, and was also divided into several parts for the experiments.
Cd concentration assay in water
Because the treatment medium was changed every 48 h, the water samples from each group of only 0 and 48 h of Cd exposure were collected, and the Cd concentration in water samples was determined. The Cd concentration in water was measured by electrothermal atomic absorption spectrophotometry (SHIMADZU AA-6300, Kyoto, Japan). The standard Cd solution (Shanxi Environment Protection Department, China) was used as a control for metal concentration. The Cd concentration was expressed as mg l−1. The same experiment was repeated three times.
Extraction and assay of NADH and NAD+
The procedure for the extraction of NADH/NAD+ was based on the modification described by Zhao et al. (1987).31 Fresh hepatopancreas, 0.1 g, was homogenized in 1 ml of 0.1 mol l−1 HCl for NAD+ or in 1 ml of 0.1 mol l−1 NaOH for NADH determination. The homogenates were then heated in a boiling water bath for 5 min, cooled in an ice bath, and centrifuged at 10
000g for 10 min at 4 °C. Supernatants were neutralized by 0.1 mol l−1 NaOH or HCl, respectively, and then centrifuged at 10
000g for 10 min at 4 °C. Finally, supernatants were kept on ice for NADH/NAD+ assays. The procedure for NADH/NAD+ assays was performed according to the method described by Matsumura and Miyachi (1983).32 Because of the light sensitivity of phenazine ethosulfate (PES) and 3-(4,5-dimethylthiazolyl-2)-2,5-diphenyltetrazolium bromide (MTT), measurements were carried out at low light. Equal volumes of 0.1 mol l−1 Tris–NaOH buffer, 40 mmol l−1 EDTA, 4.2 mmol l−1 MTT, 16.6 mmol l−1 PES, and 5 mol l−1 ethanol were mixed just before the assay, and 500 μl of this mixture was transferred into microtubes. Nicotinamide adenine dinucleotides or biological samples were added to the mixture and the volume was brought to 900 μl with 0.1 mol l−1 NaCl. Tubes containing the assay media were incubated for 5 min in a 37 °C water bath. Enzyme cycling was initiated by adding either 100 μl of 100 U per ml alcohol dehydrogenase solution (ADH, Sigma Chemical Co., St. Louis, MO, U.S.A.) for NAD+ and NADH determination. With each biological sample, a blank measurement was also made by adding 0.1 mol l−1 Tris-NaOH buffer instead of the enzyme. After 40 min of cycling time, the reactions were stopped by adding 500 μl of the 6 mol l−1 NaCl stock solution. The microtubes with NaCl were centrifuged for 5 min at 4 °C and 10
000g. The supernatant was carefully siphoned off and the pellet was solubilized in 1 ml of 96% ethanol. Absorbance at 570 nm and spectra from 400 to 800 nm were determined using a Spectra Max M5 microplate reader (Molecular Devices Corp., San Francisco, CA, U.S.A.).
Assessment of the mitochondrial membrane potential (Δψm) using JC-1
The method of mitochondrial isolation was a modification of the method described by Kun et al. (1979).33 Fresh hepatopancreas was chilled on ice and washed in a separation medium containing 0.4% bovine serum albumin (BSA) (0.22 M mannitol, 0.07 M sucrose, 0.02 M 4-(2-hydroxyethyl)-1-piperazine ethanesulphonic acid (HEPES), 2 mM Tris–HCl, pH 7.2, 1 mM ethylenediaminetetraacetic acid (EDTA)) in a prechilled glass Petri dish. Tissues were suspended in a separation medium (1
:
20, w/v) containing 0.4% BSA and then homogenized with a prechilled glass homogenizer. The homogenate was centrifuged at 3000g for 1.5 min at 4 °C, and the supernatant was collected and centrifuged at 17
500g for 2.5 min at 4 °C. The resulting pellet was washed with a separation medium and then centrifuged at 17
500g for 4.5 min. The pellet was resuspended in a suspension medium (0.22 M mannitol, 0.07 M sucrose, 0.01 M Tris–HCl, pH 7.2, 1 mM EDTA) and was centrifuged at 17
500g for 4.5 min. The pellet was finally resuspended in the suspension medium at a ratio of 1.0 ml suspension medium per 1.0 g of the starting material. JC-1 is an important fluorescent carbocyanine dye for the assessment of the mitochondrial membrane potential (Δψm). Depending on the mitochondrial membrane potential, it accumulates in the mitochondrial membrane in two forms (monomers or dimers). The negative potential of the inner mitochondrial membrane facilitates the formation of JC-1 aggregates, which results in a shift of JC-1 aggregate fluorescence. The measurement of the JC-1 aggregate fluorescence is a convenient and reliable method for the estimation of the mitochondrial membrane potential. According to the described requirements of the mitochondrial membrane potential assay kit with JC-1 (Beyotime Institute of Biotechnology, Jiangsu, China), 20 μl purified mitochondria sample were added to 180 μl JC-1 (1 μg ml−1), and were then placed in black 96-well flat-bottom microtiter plates. The JC-1 aggregate fluorescence values were measured using a spectrophotometer. The excitation and emission wavelengths of the spectrophotometer were 490 nm and 590 nm, respectively. The membrane potential of the sample was expressed in the value of fluorescence emissions.
Determination of ATP/ADP ratios
According to the modified method of Anderson and Murphy (1976),34 the ratio of ATP to ADP was determined by high performance liquid chromatography (HPLC). Hepatopancreases (0.1 g) were homogenized with 1.5 ml ice-cold 0.6 mol l−1 perchloric acid in an ice bath for 20 min. The homogenate was centrifuged using a refrigerated centrifuge (Eppendorf 5415R, Hamburg, Germany) for 30 min at 16
000g at 0 °C. The clear supernatant was removed and neutralized to pH 7 using 6 mol l−1 KOH. After storage for 2 hours at 4 °C, the neutralized supernatant was centrifuged for 10 min at 1468g at 0 °C to precipitate potassium perchlorate. Potassium perchlorate was removed by paper filtration, and the clear supernatant was stored at −25 °C. Before the measurement by HPLC, the supernatant was filtered through a 0.5 μm filter and diluted to 0.5 ml. Calibration curves were constructed using ATP and ADP standards (Sigma Chemical Co., Poole, Dorset, UK). The presence of ATP and ADP in the samples was analyzed using a HPLC system (Shimadzu, Kyoto, Japan) including a SCL-10Avp controller, a LC-10Avp pump, and a SPD-10Avp UV-visible spectrophotometric detector with the peaks being detected and analyzed at 254 nm. Chromatographic separation was achieved on a Diamonsil 5U C18 column (250 mm × 4.6 mm, Dikma technologies Inc., USA). The mobile phase consisted of 0.5% methanol of 0.05 M ammonium dihydrogen phosphate pH 6.0 (adjusted using 1 mol l−1 KOH). The flow rate of the mobile phase was 1.0 ml min−1, and the injection volume was 10 μl. The retention times for ATP and ADP were 9.3 and 11.5 min, respectively. ATP and ADP in the samples were identified by comparison with the retention time of standards, while their concentrations were determined using an external standard method. The results were expressed in terms of micrograms of ATP or ADP nmol per mg protein from which the ratio of ATP to ADP was calculated. The protein concentration in the tissue was determined using the method of Bradford (1976)35 where bovine serum albumin was used as a standard.
Transmission electron microscopy
Following the method of Liu et al. (2013),36 fresh hepatopancreases were immediately fixed in 2% (v/v) glutaraldehyde for 2 h and post-fixed in 1% (v/v) osmic acid. After dehydration in a graded series of acetone, the samples were rinsed in propylene oxide and infiltrated with epoxy resin. Ultrathin sections were stained with uranyl acetate and lead citrate and electron micrographs were prepared with a transmission electron microscope (JEM-1011, JEOL, Tokyo, Japan).
Biochemical analysis
The glycogen concentration in the hepatopancreas was measured using the method of Seifter et al. (1950).37 Tissue digestion was performed by the addition of a concentrated sodium hydroxide solution (3 μl per 1 mg wet tissue) and heating the mixture for 20 min at 100 °C. After cooling, the glycogen hydrolysed was colored with 0.2% anthrone sulfuric acid in a boiling water bath. The colored mixture was determined spectrophotometrically by absorption at 620 nm using glucose as standard. The glycogen level was expressed as mg g−1 tissue. The alanine aminotransferase (ALT; EC 2.6.1.2) and aspartate aminotransferase (AST; EC 2.6.1.1) activities of homogenates were measured using commercially available kits (Jiancheng Nanjing Institute of Bio-engineering, Nanjing, China). Alanine and aspartate were used as the substrates of ALT and AST, respectively. For the measurement of each enzyme activity, a relevant substrate, ketoglutarate and assayed sample were incubated at 37 °C for 30 min. The reaction was stopped with 2,4-dinitrophenyl hydrazine. The substance was colored with sodium hydroxide and spectrophotometrically assayed at 505 nm. ALT and AST enzyme activities were expressed as U per mg protein.
MT measurement by enzyme linked immunosorbent assay (ELISA)
Hepatopancreases were homogenated in precooled homogenization buffer (1
:
9, w/v) including 0.5 mol l−1 NaCl, 0.26 mol l−1 EDTA, 0.1 mmol l−1 phenylmethylsulfonyl fluoride (PMSF), and 0.02 mol l−1 Tris-HCl pH 7.6. The homogenate was centrifuged at 12
000g for 15 min at 4 °C. The supernatant was collected and stored at −20 °C for MT measurements. A polystyrene microtiter plate (Corning, NY, USA) was coated with different concentrations of the MT standard ranging from 3.90 and 500.00 ng ml−1 or samples diluted by coating buffer (50 mM sodium carbonate, pH 9.6). After 12 hours at 4 °C, the coated liquid was discarded, and the plate was washed 5× with PBST (10 mM PBS in 0.05% Tween-20, pH 7.2). The nonspecific binding sites were blocked by incubating the plate with PBST containing 1% bovine serum albumin (BSA) for 2 h at 37 °C. After 5× washing with PBST, anti-MT polyclonal antiserum raised in mouse and diluted to 1
:
160
000 with 1% BSA was added, and the plate was incubated for 2 h at 37 °C. Plates were then washed 5× with PBST, and incubated for 1 h with goat antimouse IgG-HRP (Sigma, St. Louis, MO, USA) diluted to 1
:
2000 with 1% BSA. 3,3′,5,5′-Tetramethylbenzidine (TMB) chromogenic reagent (Solarbio, Beijing, China) was added to the plate after washing 5× with PBST. After incubation for 30 min, 50 μl of 2 M H2SO4 was added to plots to stop the reaction. The absorbance was read at 450 nm on a spectrophotometer.
Data analysis
Statistical computations were performed using SPSS 17.0 (SPSS Inc., Chicago, USA). Data distributions and the homogeneity of variance were tested using the Kolmogorov–Smirnov and Levene tests. Significant differences between treated and control groups were calculated by one-way ANOVA analysis with a Dunnett's post hoc test. If the prerequisites for parametric tests could not be satisfied, differences were tested using non-parametric ANOVA analyses with a Kruskal–Wallis test. All the data representing mean values of five independent sets of experiments are given as mean ± standard deviation (S.D.). Probability values less than 0.05 were considered as significant.
Results
Cd concentration assay in water
Cd concentrations in the water from each treatment group after 0 and 48 h of subchronic Cd exposure are presented in Table 1. At 0 h, no significant changes in the Cd concentration from each treatment group were observed compared to the nominal exposure concentration. However, the Cd concentrations in water of all treatment groups declined significantly (p < 0.05) after 48 h of Cd exposure compared with the nominal exposure concentration. The experiment was repeated three times with similar results.
Table 1 Cd concentration analysis in water of freshwater crab (Sinopotamon henanense) exposed to subchronic Cda
Nominal exposure concentration (mg l−1) |
Measured exposure concentration in water (mg l−1) |
1–0 h |
1–48 h |
2–0 h |
2–48 h |
3–0 h |
3–48 h |
Data are expressed as mean ± standard deviation (n = 5). Statistical significance is denoted by * p < 0.05 compared to the respective nominal exposure concentrations.
|
Control |
0 |
0 |
0 |
0 |
0 |
0 |
1.45 |
1.39 ± 0.04 |
1.02 ± 0.02* |
1.42 ± 0.01 |
0.98 ± 0.03* |
1.44 ± 0.05 |
0.91 ± 0.02* |
2.9 |
2.83 ± 0.03 |
2.01 ± 0.05* |
2.78 ± 0.07 |
1.95 ± 0.08* |
2.84 ± 0.05 |
2.12 ± 0.10* |
5.8 |
5.72 ± 0.08 |
4.56 ± 0.11* |
5.83 ± 0.10 |
4.63 ± 0.09* |
5.78 ± 0.13 |
4.58 ± 0.07* |
Impact of Cd stress on NADH/NAD+ and Δψm
The NADH, NAD+, and NADH/NAD+ ratios were measured as an important H+ carrier during mitochondrial energy metabolism. As shown in Fig. 1A–C, all three indices were higher than those of the controls after 10 day Cd exposure. With increasing exposure time, the levels decreased, while no significant difference was observed at 15 day exposure. The levels in 20 day exposed crabs showed a significant decrease compared to those of the control, 10 and 15 days. The mitochondrial membrane potential was determined by evaluating the changes of JC-1 aggregate fluorescence. As shown in Fig. 1D, the value of JC-1 aggregate fluorescence in the hepatopancreas showed a close trend with the NADH/NAD+ ratio. Compared to the control at 10 day exposure, a significantly increased value suggested an increase of Δψm. With time, Δψm in exposed crabs decreased, and a significantly lower value of JC-1 aggregate fluorescence was observed at 20 day exposure.
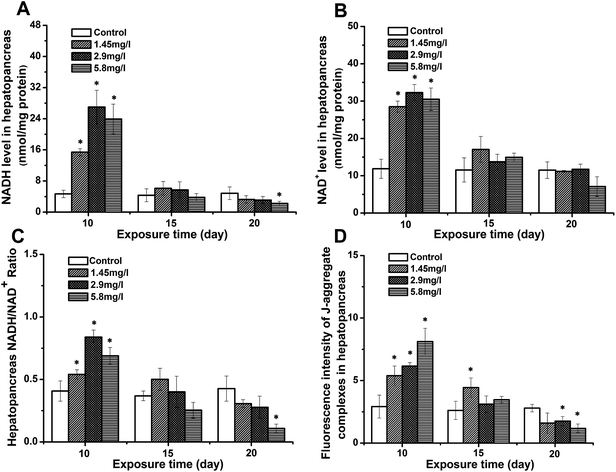 |
| Fig. 1 The level of NADH (A), NAD (B), NADH/NAD ratio (C), and fluorescence intensity of J-aggregate complexes (D) in the hepatopancreas of control and Cd-exposed crabs after 10, 15 and 20 days of experimentation. Data are expressed as mean ± standard deviation (n = 5). Comparison between the control and treatment groups is notified by * p < 0.05. | |
Bioenergetic status in response to Cd stress
To evaluate the impact of Cd stress on the crab energy status, the levels of ATP, ADP and the ratio of ATP to ADP in hepatopancreases were measured by HPLC (Fig. 2). After 10 day exposure, the levels of ATP and ADP of the hepatopancreas (Fig. 2A and B) in exposed crabs increased significantly, and the ATP/ADP ratio of hepatopancreases (Fig. 2C) was higher than that of the control. As the exposure time elapsed, the ATP and ADP levels decreased compared to 10 day exposure. However, the ADP level at 15 day exposure was still higher than that of the control, leading to a decreased ATP/ADP ratio. A significant decrease was also observed to the lowest levels at 20 day exposure.
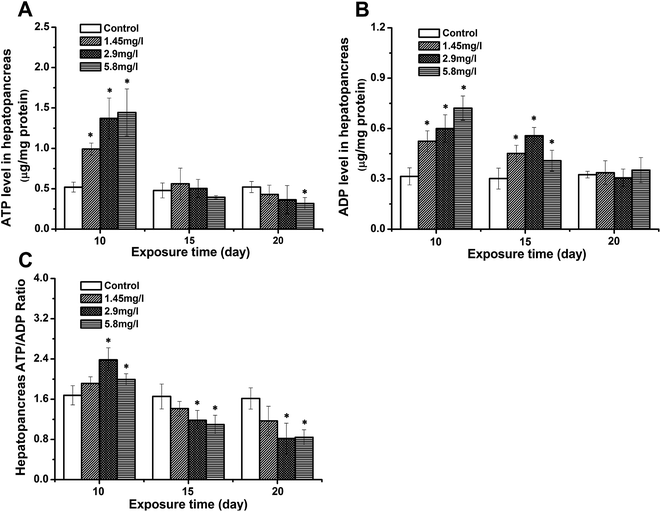 |
| Fig. 2 The levels of ATP (A), ADP (B) and the ATP/ADP ratio (C) in the hepatopancreas after 10, 15 and 20 days for control and Cd-exposed crabs. Data are expressed as mean ± standard deviation (n = 5). Statistical significance is denoted by * p < 0.05 compared to the respective control. | |
Ultrastructural observations
Fig. 3 and 4 show the effects of Cd on submicroscopic structures of hepatopancreatic cells in the control and 5.8 mg l−1 Cd exposure group. In mitochondria of normal hepatopancreatic cells discernible cristae and moderate electron dense matrices were observed (Fig. 3A). After 10 day Cd exposure, numerous mitochondria and mitochondrial cristae were found to be well-preserved in the cytoplasm of hepatopancreatic cells at 5.8 mg l−1 Cd concentration (Fig. 3B), and no apparent changes appeared. However, mitochondrial injury in hepatopancreatic cells appeared gradually with increasing Cd exposure time. Volume expansion and swollen matrices were observed in some mitochondria of the 15 day Cd exposed crab (Fig. 3C). Following exposure for 20 days, volume expansion and swollen matrices in more mitochondria could lead to disturbance in mitochondrial morphology, such as mitochondrial membrane disintegration and the disappearance of cristae (Fig. 3D). As shown in Fig. 4A, the normal rER present as single short cisternae is distributed throughout the cell, and is generally associated with mitochondria. In 10 and 15 day Cd exposed crabs, normal rER and large numbers of ribosomes on the surface of the rER could be found (Fig. 4B and C). However, dilation and vesiculation of rER cisternae were frequently observed after 20 day Cd exposure. Membrane degranulation and breaks of rER became visible, and some ribosomes were detached from the surface of the rER (Fig. 4D).
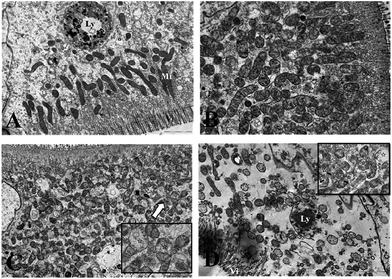 |
| Fig. 3 Effects of Cd on the ultrastructure of the hepatopancreatic mitochondria of S. henanense. (A) Normal mitochondria. Bar = 2 μm. (B) Mitochondria in Cd 5.8 mg l−1 of the 10 day exposure group. Bar = 2 μm. (C) Minor mitochondrial injury in Cd 5.8 mg l−1 of the 15 day exposure group. Bar = 2 μm; mitochondria at the upper left corner shows an edematous mitochondrial matrix. Bar = 1 μm. (D) Mitochondria in Cd 5.8 mg l−1 of the 20 day exposure group. Bar = 2 μm. Mitochondria at the upper right corner show a serious edematous mitochondrial matrix and a fragmented outer membrane. Bar = 1 μm (Vi: microvillus; Mi: mitochondria; Ly: lysosome; : edematous mitochondrial matrix; : mitochondrial outer membrane damage). | |
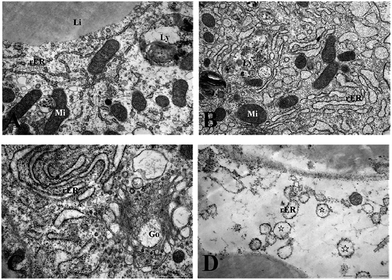 |
| Fig. 4 Effects of Cd on the rough endoplasmic reticulum and ribosomes of S. henanense. (A) Rough endoplasmic reticulum, ribosome and mitochondria in the normal group. Bar = 1 μm. (B) Rough endoplasmic reticulum and ribosome in Cd 5.8 mg l−1 of the 10 day exposure group. Bar = 1 μm. (C) Slightly damaged Golgi complex in Cd 5.8 mg l−1 of the 15 day exposure group. Bar = 1 μm. (D) Damaged rER in Cd 5.8 mg l−1 of the 20 day exposure group. Detached ribosomes from rER were observed. Bar = 2 μm (rER: rough endoplasmic reticulum; Mi: mitochondria; Li: lipid; Ly: lysosome; Go: Golgi complex; ☆: rER with few ribosomes). | |
Effect of Cd on carbohydrates, protein metabolism and MT synthesis of S. henanense
Significant decreases in hepatopancreatic glycogen were observed on 15 and 20 days, and showed a time- and dose-dependent pattern (Fig. 5A). Protein levels in the hepatopancreas in the 5.8 mg l−1 Cd concentration group at 10 day exposure were higher than those of the control, and also showed decreasing levels with time (Fig. 5B). Correspondingly, increasing activities of the ALT and AST were observed at 10- and 15 day exposure. However, both enzyme activities were significantly down-regulated at 20 day exposure compared to the control (Fig. 5C and D). Fig. 5E shows changes of hepatopancreatic MT levels in Cd exposed crabs. The MT levels in the hepatopancreas of 10 day exposed crabs were higher than the control, and showed a dose-dependent pattern. A significant up-regulation of MT levels was observed at 15 day exposure, while these levels were lower than those at 10 day exposure. Though no significant difference was observed at 20 day exposure to that of the control, the down-regulation of MT levels at 20 day exposure was still lower compared to that at 10- and 15 day exposure.
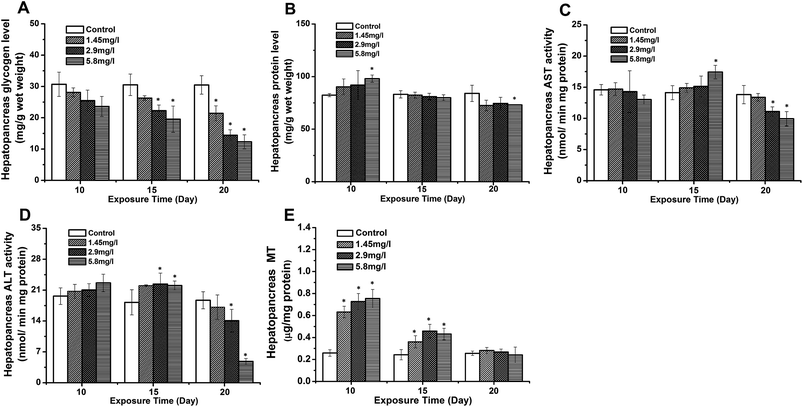 |
| Fig. 5 Effect of Cd on carbohydrates, protein metabolism, and S. henanense. Hepatopancreatic glycogen (A), protein levels (B), AST activity (C), ALT activity (D) and MT levels (E) after 10, 15, and 20 days of experimentation. Data are expressed as mean ± standard deviation (n = 5). Comparison between the control and treatment groups is notified by *p < 0.05. | |
Discussion and conclusion
The reduction of the Cd concentration in water from treatment groups after subchronic Cd exposure indicated that Cd from the ambient water could be transferred to the body of the crabs. These observations were supported by our previous study of Yang et al. (2013)38 and Ma et al. (2007),24 which demonstrated the high Cd accumulation in tissues of exposed crabs. Moreover, hepatopancreases were found to be the major targets for Cd distribution. In the present experiments, we found a variation of the bioenergetic status as a response to Cd stress, suggesting the interference of metabolic functions and energy balance in organisms. After 10 day exposure, higher levels of ATP, ADP, and the ratios of ATP/ADP in the hepatopancreas of exposed crabs suggested an enhanced energy metabolism. However, with increasing exposure time, decreased energy production was observed. Failure of the cellular energy metabolism could be one of the factors of early cell damage upon xenobiotic exposure (Sims and Muyderman, 2010).39 A severe depletion of ATP might result in the dysfunction, destabilization of several regulatory pathways mediated by enzymes and the impairment of lipids (Lucia et al., 2010; Pike et al., 2011).15,40 A longer exposure time or an increase in reactive species induced by pollutants and various other sources caused a transient drop in cellular ATP (Kabakov et al., 2002).41 The latter report confirmed the observed results of decreased ATP and increased ADP and hence altered ATP/ADP ratios in response to 15- and 20 day Cd stress in the hepatopancreas of exposed crabs. Mitochondria generate ATP and hence they are the major supplier of energy. By TEM studies, a specific cellular ultrastructural finding and difference between exposed and control crabs was revealed. With the time of Cd exposure, serious mitochondrial injury in hepatopancreatic cells appeared gradually, such as volume expansion and swollen matrices of mitochondrial membrane disintegration. A reduction and disappearance of the cristae together with mitochondrial membrane disintegration could contribute to less ATP production. Mitochondrial impairment could lead to significant disturbance in mitochondrial morphology. In accordance with our results, Martin and Forkert (2004)42 and Padmini and Usha (2011)27 also found that the dysfunction of hepatic mitochondria resulted from the impairment of mitochondria caused by environmental contaminants.
Δψm, reflecting the pumping of hydrogen ions across the inner membrane during the process of electron transport and oxidative phosphorylation, is the driving force behind mitochondrial ATP production and also a key indicator of cellular viability. Hence, a mitochondrial dysfunction associated with the impairment of mitochondrial membranes and disturbance in the membrane potential causes abatement in ATP production. Accordingly, changes of hepatopancreatic mitochondria in Cd exposed crabs could affect the level of Δψm and the NADH/NAD+ ratio. Moreover, the level of Δψm and the NADH/NAD+ ratio in the present study provided a positive correlation with ATP levels in both control and exposed crabs, reflecting disturbance in ATP production during alterations in Δψm and NADH. This view has also been supported in a study on human leukemic cell lines (Bradbury et al., 2000).43
The main substrate for the respiratory chain was supplied by glucose and amino acids by two interconnected pathways. It was necessary, therefore, to study the relationship between substance metabolism and mitochondrial energy metabolism. For most organisms, carbohydrates provided the immediate source of energy (Batatinha et al., 2013).44 Under Cd stress conditions, carbohydrate reserves are mobilized to meet the energy demands (Pretto et al., 2014).45 In accordance with this, Cd-exposed crabs typically contain fewer carbohydrates in the hepatopancreas compared to the control. This indicates increased energy demands triggered by Cd. Elevated cellular energy demands for the basal maintenance often lead to changes in energy allocation in order to cover maintenance needs in the biochemical defence of Cd stress.
Cd could also result in the upregulation of cellular protective mechanisms, such as antioxidant defense systems and cellular repair pathways (Cherkasov et al., 2006; Ivanina and Sokolova, 2008).46,47 Cd-induced expression of various proteins of functional importance, such as SOD, GSH-Px, CAT, and MT, could be observed in tissues of the freshwater crab S. henanense (Li et al., 2008; Wang et al., 2008).25,26 Among them, MT is one of the important metal-binding proteins and also plays a key role in the defense against Cd-stress. In the present study we found an up-regulation in the expression of MT in the hepatopancreas following exposure to Cd stress. Hand and Hardewig (1996)48 and Cherkasov et al. (2006)46 showed that protein synthesis is a major ATP sink in the total ATP consumption of organisms, especially under stress. Thus, the above mentioned elevated basal metabolism, specifically the up-regulation of protein synthesis involved in the defense against Cd-stress during 10 day Cd exposure, could be an important energy consumption mechanism during exposure to pollutants (Larade and Storey, 2007).49 However, the protein in the hepatopancreas at 15 day exposure showed decreasing levels compared to that at 10 day exposure. Accordingly, the activity of ALT and AST, two main aminotransferases, was up-regulated after 15 day Cd exposure. The transamination being an important pathway for the amino acid metabolism may be suggestive of increasing utilization of amino acids during Cd exposure. Prolonged 20 day Cd exposure resulted in the destruction of protein-synthesizing structures, such as membrane degranulation of rER and a loss of ribosomes detached from the surface of the rER, which may further affect the protein synthesis and the ability to metabolize amino acids within the hepatopancreas.
In the present study, significant disturbance in hepatopancreatic morphological structures was observed in the hepatopancreas of exposed crabs compared to the control. The analysis of three stress parameters namely the ATP/ADP ratio, NADH/NAD+ ratio and Δψm under the same experimental conditions revealed a significant alteration in the bioenergetic status, and enhanced induction of MT in exposed crabs in response to Cd stress. Hence, evaluation of these stress parameters as biological markers could provide quantitative risk assessments of potentially deleterious environmental exposure in comparison with routinely used biomarkers in biomonitoring programs.
Acknowledgements
This research was supported by a grant from the National Nature Science Foundation (31272319) and Research Fund for the Doctoral Program of Higher Education (20111401110010). We would like to thank Fei Wang and Jinping Liu for their technical help. We would also like to thank Dr Hans-Uwe Dahms and Dr Enmin Zou for their assistance in manuscript preparation and language editing.
References
- J. M. Pacyna, E. G. Pacyna and W. Aas, Changes of emissions and atmospheric deposition of mercury, lead, and cadmium, Atmos. Environ., 2009, 43, 117–127 CrossRef CAS PubMed.
- N. Pirrone, S. Cinnirella, X. Feng, R. B. Finkelman, H. R. Friedll and J. Leaner, Global mercury emissions to the atmosphere from anthropogenic and natural sources, Atmos. Chem. Phys. Discuss., 2010, 10, 4719–4752 CrossRef.
- A. Ciliberti, P. Berny, M. L. Delignette-Muller and V. de Buffrénil, The Nile monitor (Varanus niloticus; Squamata: Varanidae) as a sentinel species for lead and cadmium contamination in sub-Saharan wetlands, Sci. Total Environ., 2011, 409, 4735–4745 CrossRef CAS PubMed.
- J. Bai, R. Xiao, K. Zhang and H. Gao, Arsenic and heavy metal pollution in wetland soils from tidal freshwater and salt marshes before and after the flow-sediment regulation regime in the Yellow River Delta, China, J. Hydrol., 2012, 450–451, 244–253 CrossRef CAS PubMed.
- J. Feng, G. Wang, J. Sun, S. Sun and X. Liu, Metals in water and surface sediments from Henan reach of the Yellow River, China, Sci. China: Chem., 2010, 53, 1217–1224 CrossRef CAS PubMed.
- S. Lin, I. Hsieh, K. Huang and C. Wang, Influence of the Yangtze River and grain size on spatial variations of heavy metals and organic carbon in the East China Sea continental shelf sediments, Chem. Geol., 2002, 182, 377–394 CrossRef CAS.
- L. Zhou, Q. Wu and G. Gao, Remediation of Lead-Zinc Contaminated Soil in China, Appl. Mech. Mater., 2012, 209–211, 1116–1119 CrossRef.
- Q. H. Yuan, Y. L. Ye and Z. S. He, Research on heavy metal pollution of water in Lanping mining area, Occup. Health, 2010, 26, 2235–2236 CAS.
- T. Han, Q. Wang and L. Wang, Ecological investigation of freshwater crab and river pollution in the basin of Qinhe River, Sichuan J. Zool., 2008, 27, 804–806 Search PubMed.
- L. Pan and H. Zhang, Metallothionein, antioxidant enzymes and DNA strand breaks as biomarkers of Cd exposure in a marine crab, Charybdis japonica, Comp. Biochem. Physiol., Part C: Toxicol. Pharmacol., 2006, 144, 67–75 CrossRef PubMed.
- A. Gennari, E. Cortese, M. Boveri, J. Casado and P. Prieto, Sensitive endpoints for evaluating cadmium-induced acute toxicity in LLC-PK1 cells, Toxicology, 2003, 183, 211–220 CrossRef CAS.
- D. Liu, B. Yan, J. Yang, W. Lei and L. Wang, Mitochondrial pathway of apoptosis in the hepatopancreas of the freshwater crab Sinopotamon yangtsekiense exposed to cadmium, Aquat. Toxicol., 2011, 105, 394–402 CrossRef CAS PubMed.
- H. De Smet and R. Blust, Stress responses and changes in protein metabolism in carp Cyprinus carpio during cadmium exposure, Ecotoxicol. Environ. Saf., Mar., 2001, 48, 255–262 Search PubMed.
- F. Pierron, M. Baudrimont, A. Bossy, J. P. Bourdineaud, D. Brethes and P. Eile, Impairment of lipid storage by cadmium in the European eel (Anguilla anguilla), Aquat. Toxicol., 2007, 81, 304–311 CrossRef CAS PubMed.
- M. Lucia, J. M. André, P. Gonzalez, M. Baudrimont, M. D. Bernadet and K. Gontier, Effect of dietary cadmium on lipid metabolism and storage of aquatic bird Cairina moschata, Ecotoxicology, 2010, 19, 163–170 CrossRef CAS PubMed.
- R. Sousa, C. Antunes and L. Guihermino, Ecology of the invasive asian clam Corbicula fluminea (Müller, 1774) in aquatic ecosystems: an overview, Ann. Limnol., 2008, 44, 85–94 CrossRef.
- C. Moreirinha, S. Duarte, C. Pascoal and F. Cássio, Effects of cadmium and phenanthrene mixtures on aquatic fungi and microbially mediated leaf litter decomposition, Arch. Environ. Contam. Toxicol., 2011, 61, 211–219 CrossRef CAS PubMed.
-
J. F. McCarthy and L. R. Shugart, Biological markers of environmental contamination, in Biomarkers of Environmental Contamination, ed. J. F. McCarthy and L. R. Shugart, Lewis Publishers, Boca Raton, FL, USA, 1990, pp. 3–14 Search PubMed.
- P. Nogueira, M. Pacheco, M. Lourdes Pereira, S. Mendo and J. M. Rotchell, Anchoring novel molecular biomarker responses to traditional responses in fish exposed to environmental contamination, Environ. Pollut., 2010, 58, 1783–1790 CrossRef PubMed.
- M. Oliveira, M. Pacheco and M. A. Santos, Fish thyroidal and stress responses in contamination monitoring – an integrated biomarker approach, Ecotoxicol. Environ. Saf., 2011, 74, 1265–1270 CrossRef CAS PubMed.
- O. Malev, M. Srut, I. Maguire, A. Stambuk, E. A. Ferrero and S. Lorenzon, Genotoxic, physiological and immunological effects caused by temperature increase, air exposure or food deprivation in freshwater crayfish Astacus leptodactylus, Comp. Biochem. Physiol., Part C: Toxicol. Pharmacol., 2010, 152, 433–443 CrossRef PubMed.
- S. Ben-Khedher, J. Jebali, N. Kamel, M. Banni, M. Rameh and A. Jrad, Biochemical effects in crabs (Carcinus maenas) and contamination levels in the Bizerta Lagoon: an integrated approach in biomonitoring of marine complex pollution, Environ. Sci. Pollut. Res., 2013, 20, 2616–2631 CrossRef CAS PubMed.
- S. Zhao, C. Feng, W. Quan, X. Chen, J. Niu and Z. Shen, Role of living environments in the accumulation characteristics of heavy metals in fishes and crabs in the Yangtze River Estuary, China, Mar. Pollut. Bull., 2012, 64, 1163–1171 CrossRef CAS PubMed.
- W. Ma, L. Wang, Y. He and Y. Yan, Tissue-specific cadmium and metallothionein levels in freshwater crab Sinopotamon henanense during acute exposure to waterborne cadmium, Environ. Toxicol., 2007, 23, 393–400 CrossRef PubMed.
- Y. Li, L. Wang, N. Liu, Q. Wang, Y. He and F. Men, Effects of cadmium on enzyme activities and lipid peroxidation in freshwater crab Sinopotamon yangtsekiense, Acta Hydrobiol. Sin., 2008, 32, 373–379 CrossRef CAS.
- L. Wang, B. Yan, N. Liu and Y. Li, Effects of cadmium on glutathione synthesis in hepatopancreas of freshwater crab, Sinopotamon yangtsekiense, Chemosphere, 2008, 74, 51–56 CrossRef CAS PubMed.
- E. Padmini and M. Usha Rani, Mitochondrial membrane potential is a suitable candidate for assessing pollution toxicity in fish, Sci. Total Environ., 2011, 409, 3687–3700 CrossRef CAS PubMed.
- I. M. Sokolova, M. Frederich, R. Bagwe, G. Lannig and A. A. Sukhotin, Energy homeostasis as an integrative tool for assessing limits of environmental stress tolerance in aquatic invertebrates, Mar. Environ. Res., 2012, 79, 1–15 CrossRef CAS PubMed.
- G. C. Brown, Control of respiration and ATP synthesis in mammalian mitochondria and cells, Biochem. J., 1992, 284, 1–13 CAS.
- D. F. Rolfe and G. C. Brown, Cellular energy utilization and molecular origin of standard metabolic rate in mammals, Physiol. Rev., 1997, 77, 731–758 CAS.
- Z. Zhao, X. Hu and C. W. Ross, Comparison of tissue preparation methods for assay of nicotinamide coenzymes, Plant Physiol., 1987, 84, 987–988 CrossRef CAS PubMed.
-
H. Matsumura and S. Miyachi, Methods in Enzymology, ed. (A. San Pietro), Academic Press, New York, 1983, pp. 465–470 Search PubMed.
-
E. Kun, E. Kirsten and W. N. Piper, Stabilization of mitochondrial functions with digitonin, in Methods in Enzymology, ed. S. Fleischer and L. Packer, Academic Press, San Diego, 1979, pp. 115–118 Search PubMed.
- F. S. Anderson and R. C. Murphy, Isocratic separation of some purine nucleotide, nucleoside, and base metabolites from biological extracts by high-performance liquid chromatography, J. Chromatogr., 1976, 121, 251–262 CrossRef CAS.
- M. M. Bradford, A rapid and sensitive method for the quantization of microgram quantities of protein utilizing the principle of protein-dye binding, Anal. Biochem., 1976, 72, 248–254 CrossRef CAS.
- D. Liu, J. Yang and L. Wang, Cadmium induces ultrastructural changes in the hepatopancreas of the freshwater crab Sinopotamon henanense, Micron, 2013, 47, 24–32 CrossRef CAS PubMed.
- S. Seifter, S. Dayton, B. Novic and E. Muntwyler, The estimation of glycogen with the anthrone reagent, Arch. Biochem., 1950, 25, 191–200 CAS.
- J. Yang, D. Liu, W. Jing, H. U. Dahms and L. Wang, Effects of Cadmium on Lipid Storage and Metabolism in the Freshwater Crab Sinopotamon henanense, PLoS One, 2013, 8(10), e77569 CAS.
- N. R. Sims and H. Muyderman, Mitochondria, oxidative metabolism and cell death in stroke, Biochim. Biophys. Acta, 2010, 1802, 80–91 CrossRef CAS PubMed.
- L. S. Pike, A. L. Smift, N. J. Croteau, D. A. Ferrick and M. Wu, Inhibition of fatty acid oxidation by etomoxir impairs NADPH production and increases reactive oxygen species resulting in ATP depletion and cell death in human glioblastoma cells, Biochim. Biophys. Acta, 2011, 1807, 726–734 CrossRef CAS PubMed.
- A. E. Kabakov, K. R. Budagova, D. S. Latchman and H. H. Kampinga, Stressful preconditioning and HSP70 overexpression attenuate proteotoxicity of cellular ATP depletion, Am. J. Physiol., 2002, 283, C521–C534 CrossRef CAS PubMed.
- E. J. Martin and P. G. Forkert, Evidence that 1,1-dichloroethylene induces apoptotic cell death in murine liver, J. Pharmacol. Exp. Ther., 2004, 310, 33–42 CrossRef CAS PubMed.
- D. A. Bradbury, T. D. Simmons, K. J. Slater and S. P. Crouch, Measurement of the ADP: ATP ratio in human leukaemic cell lines can be used as an indicator of cell viability, necrosis and apoptosis, J. Immunol. Methods, 2000, 240, 79–92 CrossRef CAS.
- H. A. Batatinha, C. E. da Costa, E. de França, I. R. Dias, A. P. Ladeira, B. Rodrigues, F. S. de Lira, S. C. Correia and E. C. Caperuto, Carbohydrate use and reduction in number of balance beam falls: implications for mental and physical fatigue, J. Int. Soc. Sports Nutr., 2013, 10(1), 32 CrossRef CAS PubMed.
- A. Pretto, V. L. Loro, V. M. Morsch, B. S. Moraes, C. Menezes, A. Santi and C. Toni, Alterations in carbohydrate and protein metabolism in silver catfish (Rhamdia quelen) exposed to cadmium, Ecotoxicol. Environ. Saf., 2014, 100, 188–192 CrossRef CAS PubMed.
- A. S. Cherkasov, P. K. Biswas, D. M. Ridings, A. H. Ringwood and I. M. Sokolova, Effects of acclimation temperature and cadmium exposure on cellular energy budgets in a marine mollusk Crassostrea virginica: linking cellular and mitochondrial responses, J. Exp. Biol., 2006, 209, 1274–1284 CrossRef CAS PubMed.
- A. V. Ivanina and I. M. Sokolova, Effects of cadmium exposure on expression and activity of P-glycoprotein in eastern oysters, Crassostrea virginica Gmelin, Aquat. Toxicol., 2008, 88, 19–28 CrossRef CAS PubMed.
- S. C. Hand and I. Hardewig, Downregulation of cellular metabolism during environmental stress: mechanisms and implications, Annu. Rev. Physiol., 1996, 58, 539–563 CrossRef CAS PubMed.
- K. Larade and K. B. Storey, Arrest of transcription following anoxic exposure in a marine mollusc, Mol. Cell. Biochem., 2007, 303, 243–249 CrossRef CAS PubMed.
|
This journal is © The Royal Society of Chemistry 2015 |
Click here to see how this site uses Cookies. View our privacy policy here.