Brönsted acidic ionic liquid-catalyzed conversion of hemicellulose into sugars†
Received
12th August 2014
, Accepted 16th September 2014
First published on 16th September 2014
Abstract
Development of a competent method for the conversion of hemicellulose, a lignocellulosic component, into sugars is essential. In a one-pot method, Brönsted acidic ionic liquid (BAIL)-catalyzed hydrolysis of hardwood hemicellulose at 160 °C in water media gave C5 sugars (xylose + arabinose) in 87% yield. The efficiency of the ILs and their acid strength both followed the similar trend, [C3SO3HMIM][HSO4] > [C3SO3HMIM][PTS] > [C3SO3HMIM][Cl] > [BMIM][Cl]. The ion–dipole-type interaction present between the BAIL and the substrate, which is proposed by the 1H NMR study, is suggested to help in achieving better activity with BAIL than with mineral acid, H2SO4.
1. Introduction
Efficient production of chemicals and fuels from alternative renewable resources, such as biomass, is a major challenge.1–4 The second largest component (20–35%) of plant-derived lignocellulosic biomass, hemicellulose, is usually known as a complex heteropolysaccharide as it is made up of pentoses (xylose, arabinose) and hexoses (glucose, galactose, mannose) along with minor quantities of sugar acids.5,6 Depending on the composition, hemicellulose can be named as xylan (xylose, arabinose), arabinogalactan (arabinose, galactose), glucomannan (glucose, mannose), etc. However, amongst all these hemicelluloses, xylans, which are usually present in hardwood, are available in large amounts. Generally, the degree of polymerization (DP) for hardwood hemicellulose ranges from 70 to 250 and for softwood hemicellulose from 100 to 200.7 Even though hemicellulose is available in an enormous quantity from paper and pulp industries, it is still considered as one of the most under-utilized substrates to produce chemicals. The possibility of hemicellulose undergoing hydrolysis reactions to produce sugar monomers is known from the literature.1–14 These sugars in turn are capable of producing several other industrially important chemicals, such as sugar alcohols (low-calorie sweeteners),8 furans, such as furfural and 5-hydroxymethyl furfural (HMF) as well as acids (gluconic, xylonic, levulinic, formic, etc.).9 Additionally, their conversion into other value-added chemicals is well documented.10–12 Thus, it is vital to synthesize sugars from hemicellulose with high efficiencies.
Conventionally, mineral acid- and enzyme-catalyzed methods for the hydrolysis of hemicellulose into sugars are known.13–15 But to overcome drawbacks associated with these methods, recently conversion of hemicellulose into sugars using solid acid catalysts, such as zeolites (HUSY, Hβ, HMOR) and silicoaluminophosphates (SAPOs) has been shown.16–20 However, in these reactions it was difficult to attain higher yields of sugars in respectable time.16,17 The selective conversion of hemicellulose present in bagasse has also been carried out in biphasic medium using the catalyst HUSY, obtaining 20% C5 sugars and 55% furfural.20 Conversion of hemicellulose using the microwave irradiation technique is also claimed in the literature to yield 23–31% C5 sugars.21 Since ionic liquids (ILs) show tunable properties22,23 and are considered as green solvents, their uses in the biomass-related reactions have increased in the last couple of years. In one of the latest studies, hydrolysis of hemicellulose in the presence of 1-ethyl-3-methylimidazolium chloride ionic liquid, [EMIM][Cl], and mineral acid, H2SO4 (200 mM), has been reported to achieve xylose in 47% yield. However, in the same work, a substantial amount of the ionic liquid (500 μl) was used compared to that of the substrate (27 mg).24 Application of the IL, 1-ethyl-3-methylimidazolium hydrogensulfate, [EMIM][HSO4], in the synthesis of furfural (29%) has also been reported in the literature.25 In yet another report, synthesis of furfural from xylose using dimethylacetamide (DMA)-LiCl, CrCl2 and the IL [EMIM][Cl] has been described.26 Ionic liquids are also widely used in the dehydration reactions of C6 sugars (glucose, fructose) to yield HMF.27,28 In one of the reports, researchers have used 1-butyl-3-methyl imidazolium chloride, [BMIM][Cl], as a solvent and metal chlorides as catalysts to achieve HMF in 71% yield from glucose under microwave heating.29 Conversion of fructose into HMF using choline chloride/citric acid-based ionic liquids in a biphasic system is also known.28 A careful look at the literature reveals that to achieve these conversions, the IL must be used either as a solvent (with high concentrations) or in combination with other catalysts (typically acidic catalysts like H2SO4, metal chlorides, etc.). Besides this, the use of ILs in the pretreatment of cellulose to loosen the crystalline structure and of lignocellulosic materials to separate the components (cellulose, hemicellulose and lignin) is well documented.30–32
Considering the published studies, it is interesting to study the use of stand-alone ILs (without the addition of any extra Lewis or Brönsted acid catalysts during the reactions) in a catalytic amount for the conversion of hemicellulose. This will help in reducing the use of ILs in large quantities as well as in avoiding the use of any mineral acids and metal chlorides. In this paper, for the conversion of hemicellulose into C5 sugars (Scheme 1), we described the use of 3 different Brönsted acidic ionic liquids (BAILs) and compared their activities with mineral acids, solid acids and the neutral IL [BMIM][Cl]. Since xylose is known to form furfural in acidic medium by undergoing cyclodehydration reaction, here we observed the formation of furfural.
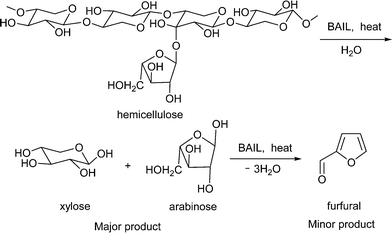 |
| Scheme 1 Conversion of hemicellulose using Brönsted acidic ionic liquids (BAILs). | |
2. Experimental
2.1 Materials
In this study, three different hemicellulose substrates were used: softwood hemicellulose [xylan from oat spelt (Aldrich Chemicals, USA, product no. X0627) has a composition ≥70% xylose, 10% arabinose and 15% glucose], hardwood hemicellulose [xylan from birchwood (Sigma Chemicals, USA, product no. X0502) has a composition ≥90% xylose] and beechwood hemicellulose [TCI Chemicals, India, product no. X0064; composed of 90% C5 sugars]. These substrates were used without any further treatment. Xylose, arabinose, glucose, furfural, 5-hydroxymethyl furfural, etc. were procured from Loba Chemicals, India and Aldrich Chemicals, USA. To quantify the oligosaccharides, a xylooligosaccharide standard was purchased from Cascade Analytical Reagents and Biochemicals, USA (product no. XYLOO).
To synthesize ILs, 1-methylimidazole (Sigma-Aldrich Chemicals, USA, 99%), 1,3-propanesultone (Alfa Aesar, India, 99%) and p-toluenesulfonic acid monohydrate (Sigma-Aldrich Chemicals, USA, 98.5%) were used as received.
The solid acid catalysts [HUSY (Si/Al = 15) and HMOR (Si/Al = 10)] were obtained from Zeolyst International, USA. Prior to use, all of the solid acid catalysts were evacuated at 150 °C for 2 h for the removal of any adsorbed materials.
2.2 Synthesis and characterization of BAILs
Various imidazolium-based BAILs and ILs (Fig. 1), such as 1-methyl-3-(3-sulfopropyl)-imidazolium hydrogensulfate ([C3SO3HMIM][HSO4]), 1-methyl-3-(3-sulfopropyl)-imidazolium para-toluenesulfonate ([C3SO3HMIM][PTS]), 1-methyl-3-(3-sulfopropyl)-imidazolium chloride ([C3SO3HMIM][Cl]), and 1-butyl-3-methylimidazolium chloride ([BMIM][Cl]) were synthesized (ESI†) and their catalytic activities were evaluated in this study. The purity of the synthesized ILs was checked by 13C and 1H NMR spectroscopy. NMR measurements were carried out with a Bruker AV 200 MHz NMR spectrometer equipped with a 4.7 Tesla superconducting magnet. The resonance frequency of proton at this magnetic field is 200 MHz. The proton NMR spectra were obtained by a standard one-pulse experiment using a 30 degree flip angle and 1 s relaxation delay. 32 to 64 scans and 32 K data points were used for data collection. The raw data obtained were Fourier transformed to obtain the frequency domain spectrum without the application of any window function. 13C spectra were obtained with a standard pulse sequence by continuous proton decoupling. A flip angle of ~30 degree, a relaxation delay of 2 s and 32 K data points were used for data collection. Proton decoupling was achieved by a standard ZGPG30 pulse technique. A standard DEPT pulse sequence with a sorting pulse of 135 degree (DEPT135) was employed for 13C spectral editing so that the CH2 peaks appeared as negative and CH and CH3 as positive. The number of scans for the 13C spectral data collection varied from a few hundred to a couple of thousand depending on the concentration. Prior to Fourier transformation, the raw 13C data (FIDs) were multiplied by an exponential window function with a line broadening (LB) of 2 Hz for sensitivity enhancement. The chemical shifts of the solvent peak (HOD) were set at 4.72–4.73 ppm in the 1H NMR of ionic liquids. Element percentage was calculated using C, H, N, S analysis (elemental microanalysis was performed using a Thermo Finnigan Flash EA 1112 series instrument) (ESI†). The thermal stability of the synthesized ILs was studied by thermogravimetric analysis (TGA); characterization (performed under air atmosphere) was performed using Mettler Toledo Instrument TGA/SDTA851e (Fig. S1†). Hammett acidity functions (HO) of catalysts were determined by means of a UV-vis spectrophotometer (Jasco V-570 spectrophotometer, Jasco Corporation, Japan) using 4-nitroaniline as a basic indicator.
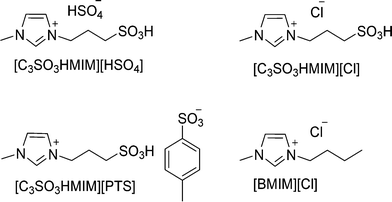 |
| Fig. 1 Ionic liquids used in the hemicellulose conversion reaction. | |
2.3 Catalytic runs
All of the catalytic reactions were performed in batch mode using a high-temperature and high-pressure reactor (Parr autoclaves, USA) equipped with a Teflon liner. In a typical reaction, 0.6 g (4.54 mmol) of solid hemicellulose was charged in the reactor and then 60 mL of water and 0.24 g of catalyst were added to it (substrate/catalyst = 2.5 (wt/wt)). The reactions were performed at a desired reaction temperature for a definite time under mechanical stirring (800 rpm). After the reaction, the reactor was cooled to room temperature under flowing water.
2.4 Analysis and calculations
The reaction mixture analysis was performed using a HPLC instrument (Agilent Infinity 1200 series) equipped with a Pb2+ column (Supelco, USA; 300 mm × 7.8 mm ID) maintained at 80 °C. Millipore water was used as an eluent with a flow rate of 0.6 mL min−1. The refractive index detector (RID) with a cell temperature of 40 °C was used to detect the products. The reaction mixture was also analyzed using a HPLC instrument (Shimadzu, Japan) equipped with a H+ column and a RID (40 °C). 0.1% orthophosphoric acid was used as the mobile phase with a flow rate 0.6 mL min−1. The calibration curve was drawn with the standard compounds. In the case of [BMIM][Cl]-, [C3SO3HMIM][HSO4]- and H2SO4-catalyzed reactions, the peaks of oligomers in HPLC overlapped with the peak due to the catalyst (IL) and H2SO4, hence quantification of the oligomers was not possible. Fig. S2 (ESI†) presents a typical HPLC profile of the reaction mixture.
For hemicellulose conversion reaction, the considered molecular weight of hemicellulose is 132 since hemicellulose is made up of C5 sugars xylose and arabinose (the molecular weight of xylose and arabinose is 150). When forming hemicellulose from C5 sugars, loss of water molecules takes place (molecular weight of H2O = 18) and the C6 sugars glucose and galactose are present in very small amounts, less than 5%. Therefore, for simplicity of calculations, we have considered the molecular weight of hemicellulose as 132 g mol−1.
%yield calculation for xylose + arabinose (C5 sugars) |
From 132 g of hemicellulose, 150 g of C5 sugars can be formed (considering 100% conversion and selectivity).
%yield of xylose + arabinose = mol of xylose + arabinose(HPLC)/mol of xylose + arabinose(theoretical, based on conversion) × 100 |
%Hemicellulose conversion = [(weight of hemicellulose charged in the reactor − weight of hemicellulose recovered after the reaction)/(weight of initial hemicellulose charged in the reactor)] × 100
3. Results and discussion
3.1 Evaluation of catalytic activities in hemicellulose conversion
As seen from Fig. 2, when hardwood hemicellulose (xylan from birchwood) hydrolysis reactions were conducted in the presence of water at 160 °C, the BAIL [C3SO3HMIM][HSO4] was very selective in forming C5 sugars (xylose + arabinose), with 87% yield within 1 h of reaction time. Similarly, other BAILs evaluated in this study, such as [C3SO3HMIM][PTS] (76%) and [C3SO3HMIM][Cl] (75%) also showed good activity for C5 sugar (xylose + arabinose) formation. Among all of the catalysts evaluated in this study, >99% conversion was observed for xylan (hemicellulose).
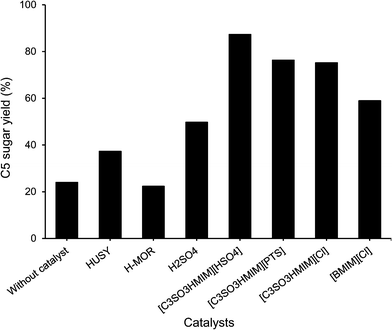 |
| Fig. 2 Conversion of hardwood hemicellulose using different catalysts. Reaction conditions: 0.6 g of hardwood hemicellulose (xylan from birchwood), 0.24 g of catalyst, 60 mL of water, 1 h, 160 °C. | |
3.2 Comparison of BAILs with mineral acid and solid acid catalysts
To compare the results of BAILs with those of a conventional mineral acid catalyst, reaction with sulphuric acid was carried out. When 0.24 g of sulphuric acid was used (4.9 mmol of H+) in the reaction, only 50% yield of C5 sugars (xylose + arabinose) was achieved (Fig. 2). The lower yield of C5 sugars is attributed to the formation of insoluble degradation products (obtained dark color reaction mixture), which we could not detect by HPLC. The formation of degradation products in this reaction was predominant because when 0.24 g of H2SO4 was used, very high concentrations of H+ ions (4.9 mmol) were present, which may initiate many side reactions. Compared to this, when 0.24 g of BAILs {[C3SO3HMIM][HSO4] (1.59 mmol), [C3SO3HMIM][PTS] (0.63 mmol), and [C3SO3HMIM][Cl] (1.0 mmol)} were used in the reaction solution, very low concentrations of H+ ions are present. To nullify the effect of the higher concentration of H+ in H2SO4 on the product formation, we carried out the reaction with sulphuric acid using the same H+ concentration (0.63 mmol) in the solution as that when [C3SO3HMIM][PTS] was used as a catalyst. In this reaction, 65% yield of C5 sugars was observed, which is lower than the yield of the [C3SO3HMIM][PTS] catalyst (76%). We also performed reactions with varying molar concentrations of H+ by charging different concentrations of H2SO4; the results for C5 sugar formation (yield) are summarized below.
0.0324 mmol of H+ (33% yield) < 0.64 mmol of H+ (65%) < 1.26 mmol of H+ (68%) < 2.4 mmol of H+ (76%) < 4.8 mmol of H+ (50%) |
As seen, there is an optimum concentration of H2SO4 (2.4 mmol of H+) at which the highest yield (76%) is observed. However, compared to BAILs 0.63 mmol, the higher concentration of H2SO4 is required to achieve an almost similar activity to that observed with the BAIL. This difference in the activity can be explained on the basis of the ion–dipole interaction (for more details, please refer to section 3.7).
The results obtained for BAILs are also compared with those for the solid acid catalysts HUSY (Si/Al = 15) and HMOR (Si/Al = 10) using similar catalyst loading (0.24 g). The results (Fig. 2) indicate the formation of 37% and 22% C5 sugars (xylose + arabinose). Reactions with solid acid catalysts were also carried out by maintaining similar H+ concentrations (0.63 mmol, same as when the IL [C3SO3HMIM][PTS] was used in the reaction). To achieve this, we altered the catalyst loading in the reaction [HUSY, 1.16 g (total acidity 0.55 mmol g−1); HMOR, 0.53 g (total acidity 1.20 mmol g−1), ESI,† Fig. S3]. In these reactions, 61% yield of C5 sugars was observed for HUSY and 48% yield for HMOR. This shows that even when very high (weight basis, 1.16 g and 0.53 g) loading of solid acid catalysts was used compared to those of BAILs (0.24 g) to maintain the same H+ concentration in the solution, lower yields were obtained with solid acid catalysts. We believe that the difference in the activity between BAILs and solid acid catalysts arises due to the difference in the catalytic system, as BAILs are soluble in water, whereas solid acid catalysts are not. This gives rise to a difference in the interaction between the catalyst and the substrate.
Reactions with hardwood hemicellulose (xylan from birchwood) were also carried out using p-toluenesulfonic acid monohydrate (PTSA) and HCl catalysts by maintaining the similar H+ concentration, similar to that of the [C3SO3HMIM][PTS] BAIL catalyst (0.63 mmol of H+). In these reactions at 170 °C within 1 h of reaction time, 44% and 39% yield of C5 sugars for PTSA and HCl catalysts were observed, respectively. Compared to the [C3SO3HMIM][PTS] catalyst (76% yield of C5 sugars), PTSA and HCl showed lower yields, which again confirmed the fact that BAILs are better catalysts than mineral acids.
3.3 Effect of time and temperature on conversion of hemicellulose
Further, to improve the C5 sugar yield obtained using BAILs, we carried out the reactions for a longer time using [C3SO3HMIM][PTS] and [C3SO3HMIM][HSO4] catalysts at 160 °C. As observed from Fig. 2, when the reaction was conducted for 1 h with the [C3SO3HMIM][HSO4] catalyst, 87% yield of C5 sugars was obtained. Further, with the increase in time, we could not see any increase in the C5 sugar yield compared to the result for 1 h of reaction time (87%), but the concentration of furfural started to build up in the reaction solution. This is because in the presence of an acid, xylose is known to undergo dehydrocyclization reactions to form furfural. We could detect a sharp decrease in the C5 sugar yield (33%) and a decrease in carbon balance when reactions were done for a longer time. This is because of the formation of degradation products, which could not be detected by HPLC analysis (since they are not completely soluble and not eluting). With the [C3SO3HMIM][PTS] catalyst, we also observed a similar trend of decrease in C5 sugar (57%, 5 h) yield. With this catalyst, we performed the reaction for 30 min since at 1 h, 76% yield of C5 sugars was observed; hence there is a possibility that the optimum time might be shorter. Yet, within a 30 min reaction time under similar reaction conditions, we observed 74% C5 sugar yield.
To check the effect of reaction temperature on the conversion of hemicelluose into C5 sugars, the reactions were performed using beechwood hemicellulose and the [C3SO3HMIM][HSO4] catalyst for 1 h. When reaction was performed at 150 °C, 33% C5 sugar yield was achieved. By increasing the temperature to 170 °C, enhancement in the C5 sugar yield (48%) was observed, although the best result was obtained when the reaction was performed at 160 °C (87% C5 sugar yield). Temperature studies were also carried out with the [C3SO3HMIM][PTS] catalyst for 1 h reaction time. Using this catalyst, 20% C5 sugar yield was observed at 150 °C. By increasing the reaction temperature to 170 °C, 28% C5 sugars was obtained. To check the possibility of whether at lower temperatures and longer reaction time higher yields for C5 sugars can be obtained, the reaction was performed at 130 °C for 6 h, obtaining 77% C5 sugar yield.
3.4 Recycle study and stability of BAILs
The recycle study carried out with the [C3SO3HMIM][PTS] catalyst showed a slight decrease in the activity after each reaction (1st run: 76%; 2nd run: 61%; 3rd run: 49%). This is because we could recover only ca. 65% BAIL after each run, but we charged the same amount of substrate (0.6 g) in the reactor. The change of the substrate/catalyst ratio from 2.5 to 3.75 (wt/wt) might be responsible for lower yields.
The similar trend in the activity was also observed with the [C3SO3HMIM][HSO4] catalyst. Both the catalysts, [C3SO3HMIM][HSO4] and [C3SO3HMIM][PTS], were isolated after the 1st recycle run and were characterized by 1H NMR spectroscopy (D2O, 200 MHz) to check their stability. As observed from Fig. 3, in the 1H NMR spectra of the BAIL [C3SO3HMIM][HSO4], we could not detect any major change in the proton NMR of the fresh and recovered BAIL catalysts. Nevertheless, we could observe some small extra peaks in the spectrum recorded for the recycled BAIL, which indicates that the catalyst contains some impurities (mostly from the recovery process). However, the appearance of all the peaks in the NMR spectra of recycled BAILs that correspond to those of fresh BAILs may suggest that the BAILs are stable under the reaction conditions.
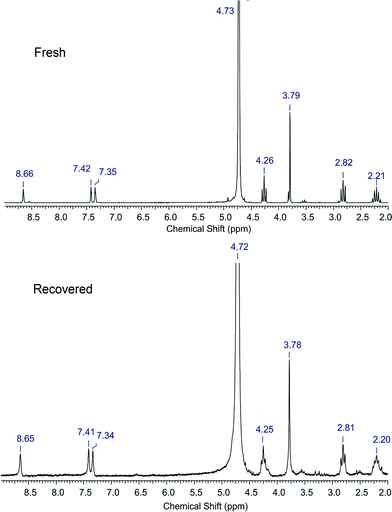 |
| Fig. 3
1H NMR spectra of fresh [C3SO3HMIM][HSO4] and recovered [C3SO3HMIM][HSO4] BAILs. | |
3.5 Conversion of different types of hemicellulose into C5 sugars using BAILS
To generalize the catalytic system, hemicellulose isolated from three different sources, such as hardwood hemicellulose (xylan obtained from birchwood) and softwood hemicellulose (xylan obtained from oat spelt and beechwood), were subjected to hydrolysis reactions. It was observed that when the reactions were performed using the [C3SO3HMIM][PTS] catalyst at 160 °C for 1 h, oat spelt-derived hemicellulose gave 86% C5 sugar yield, while beechwood-derived hemicellulose gave 83% yield. Compared to these, we observed 76% C5 sugar yield for birchwood hemicellulose. Along with C5 sugars, very low yields of furfural were also obtained in these reactions. The higher C5 sugar yield achieved for softwood-derived hemicellulose was because of lower DP (70-130) compared to that of hardwood-derived hemicellulose (100–250). Nevertheless, the results demonstrate the capability of BAILs to convert all types of hemicellulose in good yields.
3.6 Effect of substrate concentration and catalyst concentration on conversion of hemicellulose into C5 sugars
It is always desirable to use higher hemicellulose loadings (with respect to the solvent), hence to check the effect of hemicellulose concentrations on the catalytic results, the reaction was performed at 160 °C by charging 3 times more hardwood hemicellulose (1.8 g of birchwood hemicellulose, 3 wt%) in the reactor while maintaining similar loadings of catalyst ([C3SO3HMIM][PTS], 0.24 g) and water (60 mL). We observed 60% yield of C5 sugars in this reaction. This reveals that a marginal decrease in yield is possible within 1 h of reaction time, however when the reaction time was increased to 1.5 h we observed C5 sugar yields (73%) similar to those observed with lower hemicellulose loading (1 wt%, 76%). The above results are apparent since when the reaction was performed with higher hemicellulose concentration, the substrate/catalyst ratio was increased by 3 times compared to the standard reaction conditions (from 2.5 to 7.5 wt/wt and 7 to 21 mmol mmol−1).
Reactions were also performed at 160 °C for 1 h by varying the catalyst [C3SO3HMIM][PTS] loading but charging the similar amount of hardwood hemicellulose (0.6 g of birchwood hemicellulose) and water (60 mL) in the reactor. It was seen that with the increase in the substrate/catalyst (S/C) molar ratio from 7 to 14, the C5 sugar yield decreased from 76% to 62%. Further increasing the S/C ratio to 21 (60%) and 28 (40%) decreased the yields. Hence, the optimum substrate-to-catalyst molar ratio for hemicellulose conversion is 7 mmol mmol−1.
3.7 Ion–dipole interaction between BAIL and polysaccharide
Based on the above results, it is clear that the BAILs perform very well in this reaction and are particularly better than the mineral acids (H2SO4, HCl). This phenomenon we consider may arise from the interaction of the IL with the substrate, as illustrated in Fig. 4. It is suggested that the imidazolium ring (the cation) [C3SO3HMIM] interacts with the oxygen of the hydroxyl group present in the carbohydrate (hemicellulose, oligomers, monomers), and thus, in a way, the IL is attached to the substrate. At the same time, the anion [HSO4] will also interact with the hydrogen of the hydroxyl group and ensure that the IL is attached to the substrate. This ion–dipole-type interaction may force the IL catalyst to float along with the hemicellulose molecule in the reaction medium and make H+ from the ionic liquid (attached –SO3H group in the cation) in the periphery of the hemicellulose molecule available to undergo hydrolysis reaction.33 The interaction is also capable of breaking inter- and intramolecular hydrogen bonding present in the substrate which will loosen the structure and help to achieve better hydrolysis activity.
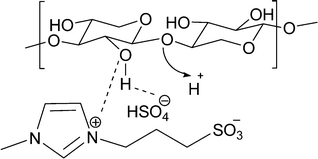 |
| Fig. 4 Ion–dipole-type interactions of alkyl imidazolium groups and hydrogen sulphate ions with hydroxyl groups of hemicellulose. | |
To propose this interaction, we performed an 1H NMR (200 MHz) study of cellobiose and a mixture of the [C3SO3HMIM][HSO4] BAIL catalyst (having acid functionality) and cellobiose in DMSO-d6 solvent. We chose cellobiose for this study in place of hemicellulose because cellobiose is soluble in DMSO-d6, while hemicellulose is not. Moreover, we used DMSO-d6 as a solvent in this study as with other solvents, H–D exchange of –OH protons is possible, which can hamper the purpose of this study. Since hydrogen bonding influences the chemical shifts, we were hopeful that this study will help us in proposing the ion–dipole-type interaction.34 The 1H NMR spectrum of cellobiose presented in Fig. 5a shows peaks of methylene and methine ‘H’ until 4.5 ppm and of hydroxyl (–OH) protons from 4.5 ppm onwards. These data match well with the earlier reported work on cellobiose.33 Further, to check the ion–dipole interaction, we recorded the 1H NMR spectrum of cellobiose + [C3SO3HMIM][HSO4], which is presented in Fig. 5c. As seen from the spectrum, peaks of the hydroxyl proton (>4.5 ppm) are missing and only one single peak is observed at 5.12 ppm. On the contrary, peaks due to methylene and methine protons are still observed in the spectrum. It is suggested that the absence of peaks is because of the fast exchange of –OH protons with –SO3H protons of [C3SO3HMIM][HSO4]. It is reported that in the presence of an acid, the rate of –OH proton exchange is fast, and hence it becomes almost impossible to detect the peaks due to –OH protons in the 1H NMR.35 A similar observation is also made in the spectrum (Fig. 5d) obtained when mineral acid is used along with cellobiose. This phenomenon can also be corroborated from the fact that the peak of α-H-1′ in cellobiose (Fig. 5a) appearing at 4.90–91 ppm shows no change in chemical shift when BAIL (Fig. 5c) or H2SO4 (Fig. 5d) is added to cellobiose. The extra peaks appearing in Fig. 5c are due to [C3SO3HMIM][HSO4] (Fig. S5†). Since under acidic environment it was not possible to show the interaction, we thus used the IL [BMIM][Cl], which does not have –SO3H functionality, to check the interaction between IL and cellobiose. Since [BMIM][Cl] is also an imidazolium-based IL, we can extrapolate the results obtained from this IL with those from BAILs used in this study. The 1H NMR spectrum recorded for the [BMIM][Cl] and cellobiose mixture in DMSO-d6 is presented in Fig. 5b. The spectrum clearly shows the peaks for hydroxyl protons from 4.5 ppm onwards. However, a careful look at both the spectra recorded for cellobiose (Fig. 5a) and cellobiose + [BMIM][Cl] (Fig. 5b) shows a difference in the peak positions and broadening of peaks. The protons of the hydroxyl group showed a slight downfield shift in the mixture of cellobiose and [BMIM][Cl] compared to those of cellobiose. For example, the peak of β-OH-3′,6′ shifts from 4.61 to 4.67 ppm and the peak of β-OH-1′ shifts from 6.64 to 6.76 ppm. To verify that these chemical shifts are not misinterpreted, the peak present for α-H-1′ (cellobiose) at 4.90 ppm is observed at the same chemical shift value in both 1H NMR spectra. This implies that only the peaks due to hydroxyl protons are shifted when cellobiose is mixed with the IL. The study reveals that the IL interacts with the substrate molecule, hence we could achieve higher catalytic activity. It is indicated that the broadening of peaks (Fig. 5b) is due to slow exchange of protons due to mild acidity of the IL [BMIM][Cl] (the presence of the hydrogen atom attached to carbon flanked between two nitrogen atoms). The 1H NMR spectrum of pure [BMIM][Cl] is presented in Fig. S6 (ESI†). To check the effect of water on the shift and broadening of peaks, we recorded the 1H NMR spectrum of water added cellobiose in DMSO-d6 solvent (spectrum not shown), however we did not observe any change in the spectrum. The similar interaction must be present when BAILs are used; however, as shown above, due to fast exchange of protons it was not possible to validate it.
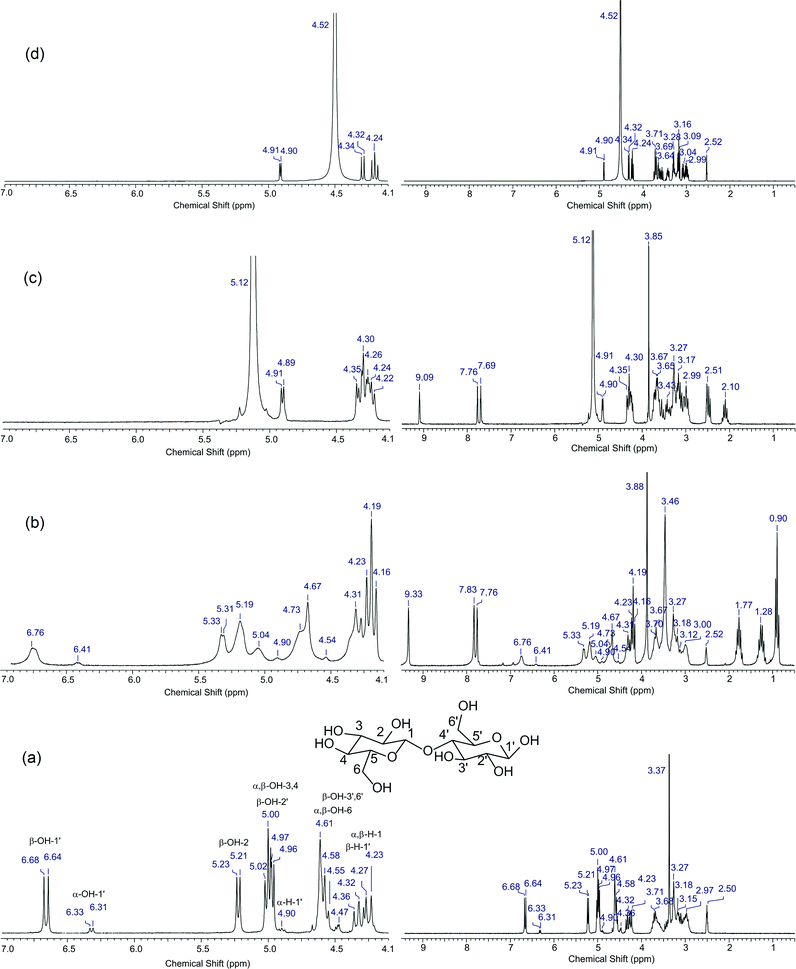 |
| Fig. 5
1H NMR study to understand the interaction of IL with cellobiose: a) Cellobiose, b) a mixture of cellobiose and [BMIM][Cl], c) a mixture of cellobiose and [C3SO3HMIM][HSO4] BAIL, and d) a mixture of cellobiose and H2SO4. All of the 1H NMR spectra were recorded in DMSO-d6 solvent. | |
The ion–dipole-type of interaction between the catalyst and the substrate is not possible with the mineral acids and therefore we suggest that BAILs perform better than H2SO4. Moreover, in an earlier study,23 the use of ILs along with H2SO4 is reported and in that work also, though not reported, it is still possible for the IL to interact with the substrate by way of an ion–dipole-type interaction but the absence of an acidic group (–SO3H) in the IL as well as the separate presence of H2SO4 is not sufficient to show higher activity, as observed in this work. The same explanation can also be extended to the [BMIM][Cl] catalyst. For this IL, we propose the presence of an interaction (by NMR study), but still it shows lower catalytic activity (50%) compared to BAILs. This information clearly suggests that the presence of the –SO3H group in the IL helps in achieving higher activity.
3.8 Characterization of catalysts
As mentioned earlier (section 2.2), the purity of the synthesized ILs was checked by 1H and 13C NMR analysis (ESI†) and we could not detect any peak for impurity present in ILs. The elemental analysis of the synthesized ILs was also carried out and it was found that the results are in close proximity with the theoretical values (ESI†). The thermal stability of ILs was studied using the TGA technique and it was observed that under the reaction conditions ILs are stable (Fig. S1†). The acidity of the solid acids (HUSY and HMOR) was checked by TPD-NH3 studies (Fig. S3†).
Next, to understand why various BAILs show difference in activity, we determined the Hammett acidity functions (HO) of catalysts by means of a UV-vis spectrophotometer using p-nitroaniline as an indicator.36,37 The Hammett function, HO, which gives information on the relative acid strength of acidic catalysts, was calculated using eqn (1). The [I]/[IH+] ratio in eqn (1) is determined from the difference in the measured absorbance after the addition of the catalyst and before addition of the catalyst to the indicator solution.
The Hammett function, HO, was calculated using eqn (1).
| HO = pK(I)aq + log([I]/[IH+]) | (1) |
Where pK(I)aq is the pKa value of the indicator in water, and [IH+] and [I] are the molar concentrations of the protonated and unprotonated forms of the indicator in the solvents, respectively. We observed the maximum absorbance for the unprotonated indicator in UV-vis spectra at 380 nm (Fig. 6); the data for HO are summarized in Table 1. The UV-vis spectra measured for water solution containing only BAIL did not show any peak at 380 nm, which confirms the fact that the peak observed at 380 nm is only due to the indicator (p-nitroaniline).
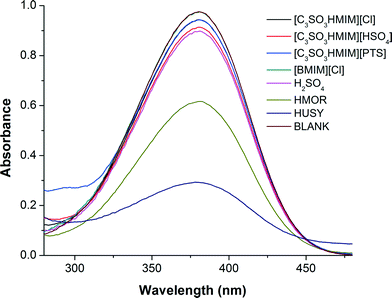 |
| Fig. 6 UV-vis spectra for measuring HO of various catalysts. | |
Table 1 Hammett acidity function (HO) data for different acidic catalysts used in this studya
Catalyst |
A
max
|
[I]% |
[IH+]% |
H
O
|
0.075 g of catalyst is mixed with 50 mL of water solution of p-nitroaniline (10 mg L−1p-nitroaniline, pKa of p-nitroaniline = 0.99).
|
Blank |
0.94 |
100 |
0.0 |
— |
HUSY (Si/Al = 15) |
0.46 |
48.9 |
51.1 |
0.97 |
HMOR (Si/Al = 10) |
0.64 |
68.0 |
32. |
1.31 |
H2SO4 |
0.78 |
82.9 |
17.1 |
1.67 |
[C3SO3HMIM][HSO4] |
0.87 |
92.5 |
7.5 |
2.08 |
[C3SO3HMIM][PTS] |
0.90 |
95.7 |
4.3 |
2.33 |
[C3SO3HMIM][Cl] |
0.91 |
96.8 |
3.2 |
2.47 |
[BMIM][Cl] |
0.94 |
100 |
— |
— |
It is understood from the literature that as the HO value decreases, the acid strength increases. Among the ILs evaluated in this study, [C3SO3HMIM][HSO4] has an HO of 2.08, which is lower than those of other BAILs, [C3SO3HMIM][PTS] and [C3SO3HMIM][Cl]. This is reflected in the activity of the catalyst, as [C3SO3HMIM][HSO4] showed the maximum yield (87%). The [BMIM][Cl] IL does not exhibit Brönsted acidity, and hence its activity is the lowest amongst all of the ILs evaluated in this study. Moreover, mineral acid (H2SO4) is observed to have a lower HO than the BAILs; however, there is a clear difference in their activities. This again points out that the BAILs possibly exhibit an ion–dipole-type interaction with the substrate molecules and hence they show better activity than mineral acid. It is evident that HUSY has a higher acid strength than HMOR, thus it showed enhanced activity (Fig. 2). Although solid acids have higher acid strength, their catalytic activity is lower than those of BAILs because they form a heterogeneous phase in reaction.
4. Conclusions
In summary, it is shown that the BAILs could efficiently convert hemicellulose derived from different sources (softwood and hardwood) in a one-pot method into C5 sugars in high yields (87%). The BAILs exhibit an ion–dipole-type interaction with the substrate molecules, as proposed by 1H NMR spectroscopy, which helps in achieving higher yields. The Hammett acidity function HO of the BAILs observed is in the order: [C3SO3HMIM][Cl] > [C3SO3HMIM][PTS] > [C3SO3HMIM][HSO4]; thus we could clearly establish a correlation with the activity and HO. It is shown that BAILs show better catalytic activity compared to solid acid catalysts (HUSY and HMOR). These BAILs thus can be used in many other acid-catalyzed reactions to improve the yields.
Acknowledgements
B. M. Matsagar thanks the Council of Scientific and Industrial Research (CSIR), New Delhi, India, for the research fellowship.
Notes and references
- M. S. Singhvi, S. Chaudhari and D. V. Gokhale, RSC Adv., 2014, 4, 8271–8277 RSC.
- J. A. Melero, J. Iglesias and A. Garcia, Energy Environ. Sci., 2012, 5, 7393–7420 CAS.
- B. Yang and C. E. Wyman, Biofuels, Bioprod. Biorefin., 2008, 2, 26–40 CrossRef CAS.
- C. E. Wyman, Annu. Rev. Energy Environ., 1999, 24, 189–226 CrossRef.
- K. Liab, P. Azadi, R. Collins, J. Tolan, J. S. Kim and K. E. L. Eriksson, Enzyme Microb. Technol., 2000, 27, 89–94 CrossRef CAS.
- Z. C. Zhang, Wiley Interdiscip. Rev.: Energy Environ., 2013, 2, 655–672 CrossRef CAS.
- T. E. Timell, Wood Sci. Technol., 1967, 1, 45–70 CrossRef CAS.
- M. Yadav, D. K. Mishra and J.-S. Hwang, Appl. Catal., A, 2012, 425–426, 110–116 CrossRef CAS PubMed.
- H. Liu, K. N. G. Valdehuesa, G. M. Nisola, K. R. M. Ramos and W.-J. Chung, Bioresour. Technol., 2012, 115, 244–248 CrossRef CAS PubMed.
- H. Kobayashi and A. Fukuoka, Green Chem., 2013, 15, 1740–1763 RSC.
- C. M. Cai, T. Zhang, R. Kumar and C. E. Wyman, J. Chem. Technol. Biotechnol., 2014, 89, 2–10 CrossRef CAS.
- F. M. A. Geilen, B. Engendahl, A. Harwardt, W. Marquardt, J. Klankermayer and W. Leitner, Angew. Chem., 2010, 122, 5642–5646 CrossRef.
- A. S. Mamman, J.-M. Lee, Y.-C. Kim, I. T. Hwang, N.-J. Park, Y. K. Hwang, J.-S. Chang and J.-S. Hwang, Biofuels, Bioprod. Biorefin., 2008, 2, 438–454 CrossRef CAS.
- P. Mäki-Arvela, T. Salmi, B. Holmbom, S. Willför and D. Y. Murzin, Chem. Rev., 2011, 111, 5638–5666 CrossRef PubMed.
- Y. Lu and N. S. Mosier, Biotechnol. Bioeng., 2008, 101, 1170–1181 CrossRef CAS PubMed.
- P. L. Dhepe and R. Sahu, Green Chem., 2010, 12, 2153–2156 RSC.
- P. Bhaumik and P. L. Dhepe, ACS Catal., 2013, 3, 2299–2303 CrossRef CAS.
- P. D. Cara, M. Pagliaro, A. Elmekawy, D. R. Brown, P. Verschuren, N. R. Shiju and G. Rothenberg, Catal. Sci. Technol., 2013, 3, 2057–2061 CAS.
- L. Zhou, M. Shi, Q. Cai, L. Wu, X. Hu, X. Yang, C. Chen and J. Xu, Microporous Mesoporous Mater., 2013, 169, 54–59 CrossRef CAS PubMed.
- R. Sahu and P. L. Dhepe, ChemSusChem, 2012, 5, 751–761 CrossRef CAS PubMed.
- Z. Zhang and Z. K. Zhao, Bioresour. Technol., 2010, 101, 1111–1114 CrossRef CAS PubMed.
- H. Olivier-Bourbigou, L. Magna and D. Morvan, Appl. Catal., A, 2010, 373, 1–56 CrossRef CAS PubMed.
- T. L. Greaves and C. J. Drummond, Chem. Rev., 2008, 108, 206–237 CrossRef CAS PubMed.
- K. R. Enslow and A. T. Bell, RSC Adv., 2012, 2, 10028–10036 RSC.
- S. Lima, P. Neves, M. M. Antunes, M. Pillinger, N. Ignatyev and A. A. Valente, Appl. Catal., A, 2009, 363, 93–99 CrossRef CAS PubMed.
- J. B. Binder, J. J. Blank, A. V. Cefali and R. T. Raines, ChemSusChem, 2010, 3, 1268–1272 CrossRef CAS PubMed.
- S. J. Dee and A. T. Bell, ChemSusChem, 2011, 4, 1166–1173 CrossRef CAS PubMed.
- S. Hu, Z. Zhang, Y. Zhou, B. Han, H. Fan, W. Li, J. Song and Y. Xie, Green Chem., 2008, 10, 1280–1283 RSC.
- X. Qi, M. Watanabe, T. M. Aida and R. L. Smith, ChemSusChem, 2010, 3, 1071–1077 CrossRef CAS PubMed.
- R. P. Swatloski, S. K. Spear, J. D. Holbrey and R. D. Rogers, J. Am. Chem. Soc., 2002, 124, 4974–4975 CrossRef CAS PubMed.
- S.-J. Kim, A. A. Dwiatmoko, J. W. Choi, Y.-W. Suh, D. J. Suh and M. Oh, Bioresour. Technol., 2010, 101, 8273–8279 CrossRef CAS PubMed.
- I. Kilpeläinen, H. Xie, A. King, M. Granstrom, S. Heikkinen and D. S. Argyropoulos, J. Agric. Food Chem., 2007, 55, 9142–9148 CrossRef PubMed.
- J. Zhang, H. Zhang, J. Wu, J. Zhang, J. He and J. Xiang, Phys. Chem. Chem. Phys., 2010, 12, 1941–1947 RSC.
- C. S. Lovell, A. Walker, R. A. Damion, A. Radhi, S. F. Tanner, T. Budtova and M. E. Ries, Biomacromolecules, 2010, 11, 2927–2935 CrossRef CAS PubMed.
-
D. Pavia, G. Lampman, G. Kriz and J. Vyvyan, Introduction to Spectroscopy, Cengage Learning, 2008 Search PubMed.
- C. C. Thomazeau, H. Olivier-Bourbigou, L. Magna, S. P. Luts and B. Gilbert, J. Am. Chem. Soc., 2003, 125, 5264–5265 CrossRef CAS PubMed.
- Y. Wang, X. Gong, Z. Wang and L. Dai, J. Mol. Catal. A: Chem., 2010, 322, 7–16 CrossRef CAS PubMed.
Footnote |
† Electronic supplementary information (ESI) available: Catalyst synthesis, 1H and 13C NMR data for ionic liquids, microanalysis data, TGA of ionic liquids and temperature-programmed desorption data for zeolites. See DOI: 10.1039/c4cy01047g |
|
This journal is © The Royal Society of Chemistry 2015 |