Packing and mobility of hydrocarbon chains in phospholipid lyotropic liquid crystalline lamellar phases and liposomes: characterisation by positron annihilation lifetime spectroscopy (PALS)†
Received
26th August 2014
, Accepted 10th November 2014
First published on 20th November 2014
Abstract
Lipid lamellar mesophases and their colloidal dispersions (liposomes) are increasingly being deployed in vivo as drug delivery vehicles, and also as models of biological membranes in fundamental biophysics studies. The permeability and diffusion of small molecules such as drugs is accommodated by a change in local curvature and molecular packing (mesophase behaviour) of the bilayer membrane molecules. Positron annihilation lifetime spectroscopy (PALS) is capable of providing in situ molecular level information on changes in free volume and void space arising from such changes in a non-perturbative manner. In this work PALS was used to systematically characterise the temperature-induced melting transitions (Tm) of saturated and unsaturated phospholipid–water systems while systematically varying lipid chain length, as both bulk lamellar mesophase and as aqueous colloidal dispersions (liposomes). A four-component fit of the data was used that provides separate PALS lifetimes for the aqueous (τ3) and organic domains (τ4). The oPs lifetime (τ4), for the lamellar phases of DSPC (C18:0), DPPC (C16:0), DMPC (C14:0) and DLPC (C12:0) was found to be independent of chain length, with characteristic lifetime value τ4 ∼ 3.4 ns. τ4 is consistently larger in the dispersed liposomes compared to the bulk mesophases, suggesting that the hydrocarbon chains are more mobile. The use of contemporary and consistent analytical approaches as described in this study is the key to future deployment of PALS to interrogate the in situ influence of drugs on membrane and cellular microenvironments.
Introduction
Transmembrane transport is often a protein-mediated process modulated by channels, carriers or pumps with the passage of molecules and ions across the membrane assisted by local fluctuations of the surrounding phospholipid bilayer. Changes in the lateral pressure profiles alter functionality and permeability1,2 and are regularly accommodated by a change in local curvature (phase behaviour).3 These dynamics are also a key determinant of the distribution profile and pharmacokinetic behaviour of drugs in vivo, because transmembrane transport of pharmacological agents is often a requisite to achieve the desired therapeutic effect.
Considerable effort has therefore been made to understand the structure–function relationships of biological membranes, with the lamellar phases of lipids fundamental to this understanding. The phospholipid bilayer model wherein the hydrophilic phosphate headgroups sandwich a hydrocarbon bilayer is commonly employed as a model membrane to understand physiological behaviour. Lamellar phases and their aqueous colloidal dispersions (liposomes) are also of considerable interest as they are an established self-assembled nanoscale delivery system,4,5 with liposomal formulations constituting the first nanomedicine approved by the United States Food and Drug Administration for clinical application.6 Liposomes offer a large internal aqueous cavity for enhanced payload and manipulation of surface chemistry to aid circulation and targeting. However, liposomal therapies remain a challenge as the effects of drug loading on the nanostructure, and the relationship between internal nanostructure and the drug release profile are still poorly understood. Hence, new insights into the transport of molecules across membrane structures is expected to have implications for drug delivery and pharmacological action.
Methods such as X-ray diffraction, nuclear magnetic resonance spectroscopy,7 fluorescence polarization8 as well as molecular dynamics simulations9–11 have been employed for studying the structure and dynamics of membrane-based systems. Positron annihilation lifetime spectroscopy (PALS)12 has been used less extensively, although its use in interrogating free volume, voids and defects in polymers is well established.13–15 In recent years PALS has also been demonstrated to have utility as an in situ molecular probe in soft condensed matter systems for which it is highly sensitive to conformational, structural and microenvironmental transformations. PALS has been used to determine the critical micelle concentration (CMC) in aqueous micellar systems;16–19 to distinguish transformations in micellar geometry20–25 as well as phase transitions in liquid crystalline systems26–30 and lipid bilayers.31–37
The basic principle of PALS is the thermalisation of a positron (e.g. from radioactive 22Na) upon injection into a material to form a bound state with an electron from the surrounding medium. This bound state is referred to as a “positronium” that may exist in two ground states, i.e. para-positronium (pPs) or ortho-positronium (oPs).12oPs is of the greatest interest as the primary mechanism of annihilation is via “pick-off” with electrons in low electron density regions. oPs therefore preferentially localises in free volumes, voids and pores. oPs is characterised by two parameters: oPs lifetime (τ) and oPs intensity (IoPs), where (τ) has been correlated with inter- and intra-chain packing (‘hole size’) and IoPs to the number of free volume elements present in the material.12 In comparison to fluorescence methods, the positron probe does not disrupt the local microenvironment of the lipid chains, and is able to provide a direct measurement of bilayer microfluidity.31–37 However studies of this nature have been sparse, with many of the observations conflicting due to differences in data analysis and experimental design, so that it is difficult to extract physicochemically-meaningful comparative information from these studies. Thus whilst PALS has demonstrated potential utility in this field a better understanding of the properties and behaviour of the PALS parameters is necessary if the technique is to emerge as a viable and generally useful method for characterising soft condensed matter systems.
The current study has therefore undertaken to understand the sensitivity of the PALS parameters to variations in the bilayer chemical structure, through a systematic characterisation of the melting transition (Tm) of a number of phospholipid–water systems varying in acyl chain length and saturation state. Specifically, the saturated phospholipid–water systems studied were DLPC (1,2-dilauroyl-sn-glycero-3-phosphocholine, C12:0), DMPC (1,2-dimyristoyl-sn-glycero-3-phosphocholine, C14:0), DPPC (1,2-dipalmitoyl-sn-glycero-3-phosphocholine, C16:0) and DSPC (1,2-distearoyl-sn-glycero-3-phosphocholine, C18:0), the chemical structures of which are illustrated in Fig. 1. In addition the effect of unsaturation was also studied by comparing the behaviour of DSPC (C18:0) to that of DOPC (1,2-dioleoyl-sn-glycero-3-phosphocholine, C18:1). Further, the state of the system with respect to dispersion as particles was studied by comparison of the non-dispersed lamellar mesophase and aqueous colloidal dispersions (liposomes) of DLPC, DPPC, DSPC, and di-C16:1 PC (1,2-dipalmitoleoyl-sn-glycero-3-phosphocholine), principally as both non-dispersed and dispersed lamellar phases have application as drug delivery vectors.38–40 To our knowledge, this is the first time these lipid self-assembly materials have been directly compared using PALS.
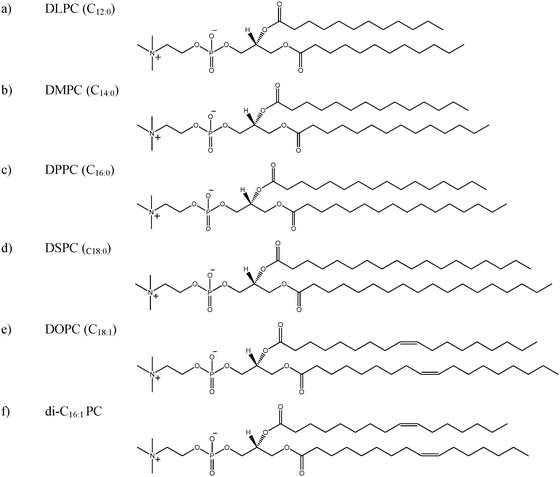 |
| Fig. 1 Chemical structure of the phospholipids investigated, (a) 1,2-dilauroyl-sn-glycero-3-phosphocholine (DLPC); (b) 1,2-dimyristoyl-sn-glycero-3-phosphocholine (DMPC); (c) 1,2-dipalmitoyl-sn-glycero-3-phosphocholine (DPPC); (d) 1,2-distearoyl-sn-glycero-3-phosphocholine (DSPC), (e) 1,2-dioleoyl-sn-glycero-3-phosphocholine (DOPC) and (f) 1,2-dipalmitoloyl-sn-glycero-3-phosphocholine (C16:1 PC). | |
Materials and methods
Saturated phospholipids DLPC (1,2-dilauroyl-sn-glycero-3-phosphocholine), DMPC (1,2-dimyristoyl-sn-glycero-3-phosphocholine), DPPC (1,2-dipalmitoyl-sn-glycero-3-phosphocholine), DSPC (1,2-distearoyl-sn-glycero-3-phosphocholine), and unsaturated phospholipids DOPC (1,2-dioleoyl-sn-glycero-3-phosphocholine) and di-C16:1 PC (1,2-dipalmitoleoyl-sn-glycero-3-phosphocholine) with a minimum purity of 99% were purchased from Avanti Polar Lipids (Alabaster, AL, USA) and used without further purification. Water purified using a MilliQ system (Millipore, Sydney, Australia) was used throughout the experiments. The 22Na source was purchased as an aqueous solution of NaCl from Perkin Elmer (Waltham, MA, US). A 1 × 1 cm Mylar source with thickness of 2.54 μm was used for PALS experiments. Silver behenate was obtained from Sigma Aldrich (St Louis, MO, USA). The sample grid used in cryo-transmission electron microscopy (cryo-TEM) measurements was obtained from ProSciTech (Turingowa QLD, Australia).
Preparation of amphiphile self-assembly materials
Lamellar mesophase samples.
Lamellar mesophases of DLPC, DMPC, DPPC and DSPC were prepared using lipid (20 mol%) in MilliQ water. Lipids were weighed into a glass vial and mixed with the appropriate weight of water with a vortex mixer. Samples were repeatedly mixed with the vortex and centrifuged until homogenous then stored at room temperature for two weeks before measurements were performed.
Aqueous colloidal dispersions (liposomes).
Liposomes of DLPC, DPPC, DSPC and DOPC were prepared by the dry-film method followed by sonication.41 Solid lipid (20 wt% final lipid concentration in water) was dissolved in a methanol–chloroform (1
:
2 v/v) mixture contained in a 250 ml round bottom flask. The solvent was removed via rotary evaporator to form a thin lipid film, to which purified water heated to above the main transition temperature (Tm) of the lipid was introduced (Table 1). The sample was mixed first via vortex mixer then on a rotary evaporator with the water bath set to approximately 10 °C above Tm until the lipid film was coarsely dispersed. The dispersed lipid was then transferred to a glass vial and sonicated with a probe sonicator (Misonix, Newtown, USA). The samples were stored at 4 °C for a maximum of 4 days until measurement. Unilamellar liposomes of ca. 50–100 nm in size with polydispersity index <0.4 were produced as measured using dynamic light scattering.
Table 1 Main transition temperature (Tm) measured for bulk lamellar mesophases
Lipid |
T
m (°C) |
Literature |
DSC |
SAXS |
DLPC |
−2.146 |
— |
— |
DMPC |
23.946 |
25.4 |
25–30 |
DPPC |
41.446 |
43.7 |
45–50 |
DSPC |
55.346 |
56.8 |
53 |
DOPC |
−16.547 |
— |
— |
di-C16:1 |
−3648 |
— |
— |
Characterization of amphiphile self-assembly materials
Positron annihilation lifetime spectroscopy (PALS).
Positron annihilation lifetime spectroscopy measurements were performed as a function of temperature, between 10–55 °C for the lamellar mesophase (‘bulk’) systems of DLPC, DMPC, DPPC and DSPC. A fixed composition of water was chosen so that all four systems had the same mole ratio of lipid to water molecules. At this water concentration, the systems undergo a melting transition (Tm) with increasing temperature (Fig. S1, ESI†). PALS measurements were conducted as a function of temperature as represented by the dashed lines (Fig. S1, ESI†) with the transition temperature confirmed by Small angle X-ray scattering (SAXS) and Differential Scanning Calorimetry (DSC).
PALS measurements were conducted on the aqueous colloidal dispersions of DLPC, DPPC, DSPC and DOPC. These were limited to a maximum of three temperatures as the time required for further PALS measurements exceeded the usable life of the samples. The lipid composition was selected so that oPs intensity of the dispersed systems yielded sufficient signal to noise (IoPs > 5%).
The PALS instrumental set-up, methodologies and data analysis protocols have previously been described.26,27,42 The samples were contained in a specially designed insulated copper holder sealed using o-rings to prevent evaporation and temperature controlled using a peltier that is accurate to ±1 °C. Samples were equilibrated for 30 min at each temperature prior to obtaining PALS spectra. The Mylar source (30 μCi) was washed with absolute ethanol and air-dried between samples. A minimum of five spectra each with 5 × 105 counts were collected at each temperature for both the lyotropic liquid crystalline mesophases and aqueous colloidal dispersions. Data were analysed initially using LT9 and then re-analysed with PAScual using a Local fit and Markov Chain Monte-Carlo Bayesian Inference (MCMC-BI) using a four component fit. PAScual provided similar fitting solutions to LT9.26,27 The components in increasing order of longevity correspond to the annihilation of para-positroniums (τ1), direct annihilation of free positrons (τ2) and the annihilation of ortho-positroniums in the aqueous and organic phases (τ3, τ4 respectively). Each lifetime has a corresponding intensity IoPs that is related to the concentration of void sites in the medium. For the fitting procedure, τ1 was fixed at 0.125 ns, with the lifetime for water (τ3) fixed at 1.8 ns (ref. 44) and a source correction of 1.857 ns and 3.52% also applied. Fig. 2 shows a typical PALS spectrum and the contributions to lifetime and intensity.
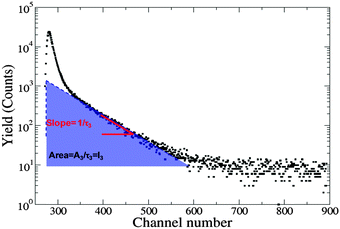 |
| Fig. 2 PALS spectrum depicting lifetime (τ3) being the slope of the oPs curve and intensity (I3) is the area under the oPs curve. | |
Small angle X-ray scattering (SAXS).
SAXS measurements were performed at the Australian Synchrotron SAXS-WAXS Beamline.45 The temperature of the sample holder was maintained by a Peltier controller to an accuracy of ±0.1 °C. Samples were equilibrated at set temperature for five min before measurement using an exposure time of 1 s for each sample. Silver behenate (lattice parameter a = 58.38 Å) was used as a calibration standard.
Differential scanning calorimetry (DSC).
DSC was performed on a Mettler Toledo 821 (Greifensee, Switzerland) with “Star Software” version 9. Samples were sealed in lightweight aluminium pans (∼10 mg) and stored at room temperature before calorimetric analysis. Measurements were conducted from 20 to 80 °C at a heating rate of 0.5 °C min−1.
Dynamic light scattering (DLS).
DLS experiments were performed on a Zetasizer Nano ZS (Malvern, Worcestershire, UK). The samples were diluted with MilliQ water and placed into a plastic micro volume cuvette (Sarstedt, Nümbrecht, Germany). Measurement temperatures were chosen to match PALS experiments with three minutes equilibration time performed at each temperature. Triplicate measurements with a minimum of 12 runs were performed.
Cryogenic transmission electron microscopy (Cryo-TEM).
The aqueous colloidal dispersions were diluted with MilliQ water (1
:
20 v/v) for cryo-TEM measurements. Approximately 4 μl of the sample that had been heated to above the Tm of the phospholipid was placed onto a 300 mesh copper TEM grid coated with a lacy carbon film (ProSciTech, Turingowa QLD, Australia) that was then rendered hydrophilic via glow discharge. Excess sample was removed by manual blotting with filter paper and the sample grid was vitrified by being rapidly plunged into liquid ethane (−190 °C). The grids were stored in liquid nitrogen before being transferred into a Gatan 626-DH Cryo-holder, where samples were maintained at −180 °C during imaging. Cryo-TEM imaging was performed on an FEI Tecnai 12 TEM (120 kV) with a MegaView III CCD camera and AnalySis imaging software (Olympus Soft Imaging Solutions). Cryo-TEM confirmed that the liposomes were unilamellar (Fig. S2, ESI†).
Results
Bulk non-dispersed lamellar mesophase systems
A discontinuity in oPs lifetime can be used to identify the transition temperature (Tm) at which a change in the membrane fluidity occurs as the bilayer undergoes transition from the rigid phases to the fluid lamellar phase (Lα) (Fig. 3). The PALS data was analysed using a four-component fit as described elsewhere.26,27,42 The two parameters of interest in the following discussion are oPs lifetime (τ) and the oPs intensity (I) which are related to molecular packing, with the lifetime representing the relative size of oPs annihilation sites and the intensity representing the relative concentration of sites. These have been further deconvoluted with both an organic (τ4, I4) and aqueous (I3) component to enable better distinction between the contributions from the hydrophobic and hydrophilic domains.27,42
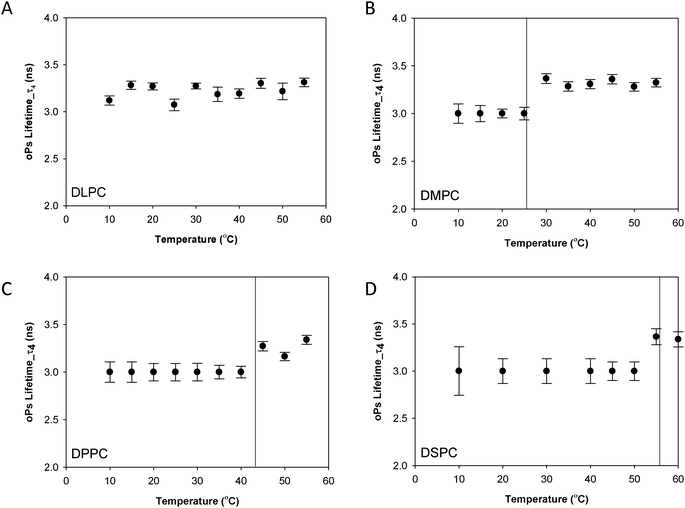 |
| Fig. 3
oPs lifetime in the organic region (τ4) for the lamellar mesophase (A) DLPC, (B) DMPC, (C) DPPC and (D) DSPC systems. Solid lines represent main transition temperatures (Tm) measured by DSC. | |
Fig. 3 shows the oPs lifetime behaviour in the organic region (τ4) as a function of temperature for the four phospholipid–water systems. The oPs lifetime for the DLPC–water system fluctuated between 3.0 to 3.4 ± 0.1 ns and showed no significant change with an increase in temperature. Similarly I4 was not affected by temperature and remained constant at approximately 10 ± 0.7% (Fig. 4A). As the Tm of this lipid occurs at −2.1 °C;46 the DLPC was already in the lamellar phase at 10 °C with no further phase transition expected (Table 1). For all DMPC, DPPC and DSPC the oPs lifetime was τ4 ∼ 3.0 ± 0.1 ns in the rigid lamellar phase (Lβ′), increasing to τ4 ∼ 3.4 ± 0.1 ns following the transition to the fluid lamellar phase (Lα). The observed discontinuity in τ4 correlated with the transition temperatures measured by DSC and SAXS in this study, and the literature Tm values. Trends in the PALS intensities for both the hydrophobic and aqueous domains were less distinct and generally demonstrated opposite effects. I4 did not change as abruptly as τ4 around the melting transition, and continued to increase as a function of temperature for DMPC, DPPC and DSPC (Fig. 4B–D). With the exception of DLPC, where I3 ∼ 3.0 ± 0.9% irrespective of temperature (Fig. 5A), the I3 of DMPC, DPPC and DSPC decreased until T ∼ Tm, after which it remained essentially invariant. The change in I3 for DSPC however was not as pronounced as for the shorter chained lipids (Fig. 5B–D).
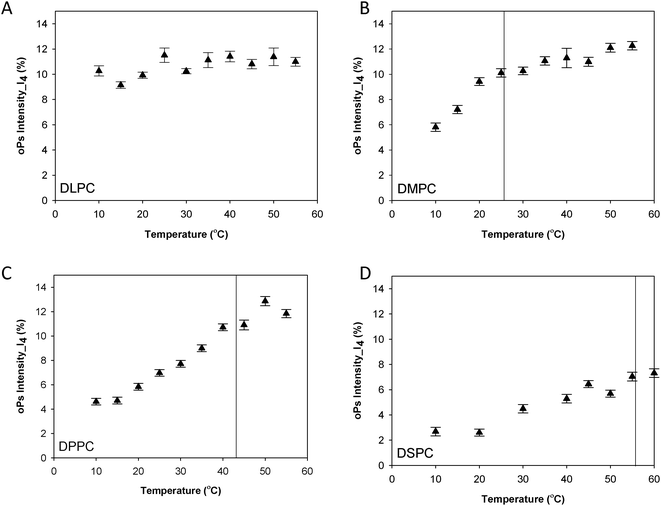 |
| Fig. 4
oPs intensity in the organic region (I4) for the lamellar mesophase (A) DLPC, (B) DMPC, (C) DPPC and (D) DSPC systems. Solid lines represent main transition temperatures (Tm) measured by DSC. | |
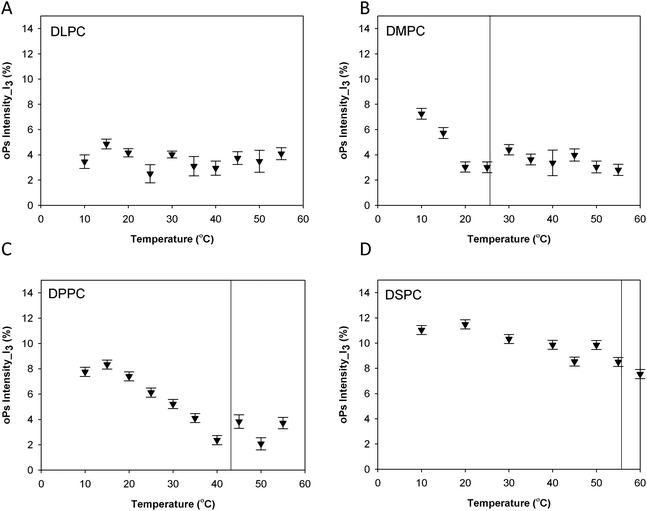 |
| Fig. 5 Intensity in the aqueous region (I3) for the lamellar mesophase (A) DLPC, (B) DMPC, (C) DPPC and (D) DSPC systems. Solid lines represent main transition temperatures (Tm) measured by DSC. | |
Effect of nanostructure on the PALS parameters of lamellar mesophases versus liposomal (colloidal) dispersions
The sensitivity of PALS to internal nanostructure has been investigated through comparison of oPs and IoPs of both the colloidal dispersions (liposomes) and lamellar mesophases of DLPC, DPPC and DSPC. The oPs lifetime in the organic region (τ4) was consistently larger in the dispersed colloidal lipid systems than in the mesophase systems, however the uncertainties for τ4 were also more significant in the liposomes. The DLPC liposomes showed no significant change in τ4 as a function of temperature (Fig. 6A) whereas, for both the DPPC and DSPC systems, the lifetime values for oPs in the liposome systems were significantly larger at temperatures above the main transition temperature (Fig. 6B and C). These observations are consistent with trends from the lamellar mesophase systems.
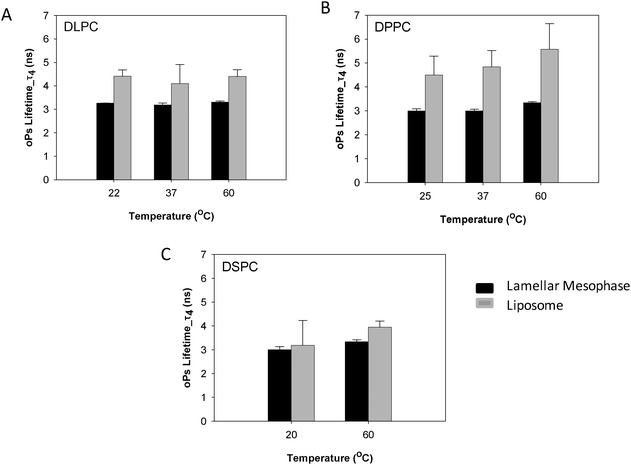 |
| Fig. 6 Comparison of oPs lifetime in the organic region (τ4) of liposomes and lamellar mesophase (A) DLPC, (B) DPPC and (C) DSPC systems. | |
The oPs intensity in the organic region (I4) was smaller overall in the liposome systems than in the non-dispersed lamellar mesophase. For the DLPC system there was no change in I4 as a function of temperature, which is consistent with the lamellar mesophase system (Fig. 7A). In contrast, the lamellar mesophase of DPPC exhibited a gradual increase in I4 with an increase in temperature. This same trend was observed in the colloidal DPPC system, but was less pronounced with only a slight increase of ∼0.5 ns at 60 °C (Fig. 7B). No significant change in I4 was observed for the DSPC liposomes as a function of temperature (Fig. 7C). This is in contrast to the lamellar mesophase system, where an increase in intensity was observed above the Tm.
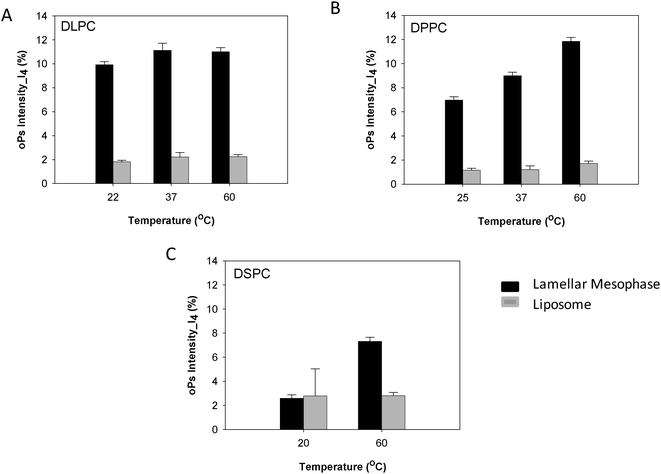 |
| Fig. 7 Comparison of oPs intensity in the organic region (I4) liposomes and lamellar mesophase (A) DLPC, (B) DPPC and (C) DSPC systems. | |
The oPs intensity in the aqueous region (I3) was significantly larger in the liposomal system than in the lamellar mesophase. For the DLPC system, no change in intensity with temperature was observed in the dispersed colloidal system, consistent with the lamellar mesophase (Fig. 8A). However, for both the DPPC and DSPC systems a decreasing trend in I3 was present as a function of temperature in the lamellar mesophase system. This trend was not observed with the liposomes (Fig. 8B and C).
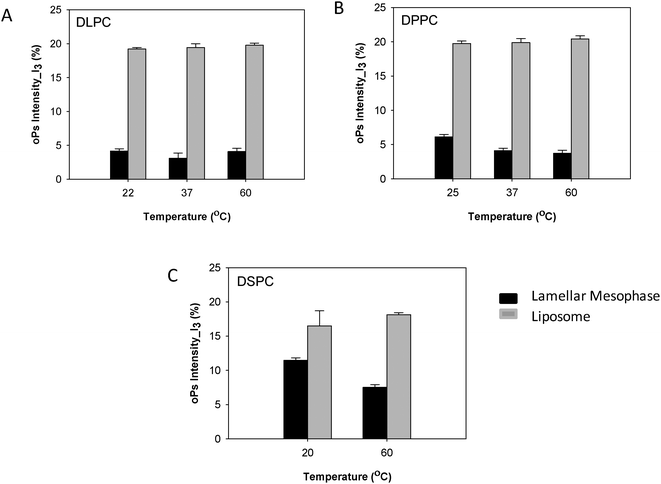 |
| Fig. 8 Comparison of oPs intensity in the aqueous region (I3) liposomes and lamellar mesophase (A) DLPC, (B) DPPC and (C) DSPC systems. | |
The total oPs intensity (I3 + I4) was ∼6% greater in the colloidal liposome system than in the lamellar mesophase system for all three lipids (Fig. 9). There was no significant difference in the total oPs intensity between the lipids of varying hydrocarbon chain lengths.
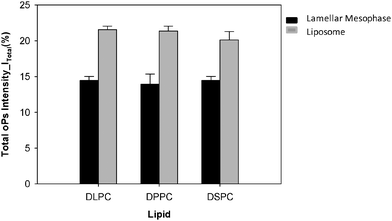 |
| Fig. 9 Total oPs intensity of liposomes and lamellar mesophase DLPC, DPPC and DSPC. | |
Effect of lipid chain structure on the PALS parameters
The effect of the lipid chain saturation on the PALS parameters of liposomal formulations with hydrocarbon chain length C16 (DPPC and di-C16:1) and C18 (DSPC and DOPC) was investigated. No significant differences were observed between the saturated and unsaturated lipids of the same chain length for the oPs lifetimes and intensities of the hydrocarbon domains (τ4, I4). Similarly, the oPs intensity in the aqueous region did not change as a function of temperature, nor did I3 differ for lipids varying in chain saturation (Fig. S3–S6, ESI†).
Discussion
Biomembrane phase transitions by positron annihilation lifetime spectroscopy
In order to understand the sensitivity of the PALS parameters to variations in the bilayer chemical structure, we have systematically undertaken to characterize the PALS behaviour of a series of phospholipid–water systems as the systems were heated through their Tm phase transition. Whilst these systems have previously been studied in the literature, interpretation and comparison of results is difficult, as the evolution of instrumentation and data analysis methods over the decades has made it difficult to directly compare the available data sets. In the current study high resolution data has been obtained across all systems enabling a much more discriminating analysis using a four-component fit of the PALS spectra to distinguish the organic and water domains.
A discontinuity in the PALS lifetime, τ4 that corresponds to the melting transition has been observed, with τ4 increasing from ca. 3 ns to 3.4 ns following the transition to the fluid lamellar phase (Lα). This trend in lifetime agrees well with past studies34–36,49 with a ΔτoPs of 400 ps consistent with that reported by both McGrath et al.35 and Jean et al.,34 although the absolute lifetime values differ. This variation is attributed to a difference in the data fitting procedures, in particular the number of components used in the analysis. In the current study the high resolution of the data has enabled a four-component fit that distinguishes separate organic and water domains.27 The abrupt change in gradient of the measured oPs lifetime of the DMPC, DPPC and DSPC bulk systems are consistent with the Tm measured by DSC and from the literature.46 Further, as τ4 is sensitive to changes in lipid chain packing, the increase in τ4 upon transition to the lamellar phase suggests a change in the free volume as a result of greater fluidity of the hydrocarbon chains in this phase. This has been observed through atomistic simulations by Sane et al.49 for similar phospholipid–water systems. The PALS lifetimes for transition to the lamellar phase are independent of the chain length and characteristic of the particular lamellar phase.35 Specifically, at the pre-transition a constant oPs lifetime τ4 ∼ 3 ns was observed, increasing to τ4 ∼ 3.4 ns for the Lα phase.
The oPs intensity in both the organic (I4) and aqueous (I3) regions was also affected by a change in temperature. However, changes in IoPs were not as distinct at the Tm as τoPs. I3 decreased with temperature and plateaued after the phase transition to the lamellar phase. On the other hand, I4 increased gradually as a function of temperature, suggesting increased oPs formation and annihilation among the hydrophobic chains in the fluid lamellar phase. This has been explained by the more abundant transient void space in which the oPs can exist as a result of more mobile lipid chains (Fig. 10). In contrast, McMahon et al.36 observed a decrease in oPs intensity as a function of temperature for similar liposome systems. However these authors only deconvoluted the raw PALS data into two components and did not separate the organic phase from the aqueous phase, so that it is highly probable the oPs intensity in water (I3) masked the trend for I4. Jean and Hancock34 have also previously investigated lamellar mesophase DPPC–water systems with similar lipid concentrations to this study. They reported no change in total oPs intensity as a result of phase transition, similar to the present observations.
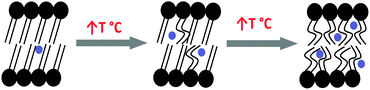 |
| Fig. 10 Schematic showing increased transient void space in lipid bilayers with increased temperature. Blue circles represent oPs molecule. | |
Comparison of hydrocarbon chain packing between lamellar mesophase and dispersed colloidal liposome systems
Previous studies that investigated the behaviour of lamellar phases using PALS often have not placed great emphasis on stating the composition of the sample. Some authors, such as Jean et al.34 utilised concentrated lamellar mesophase systems with reduced water content, while others like McMahon et al.36 measured dispersed colloidal liposome systems in excess water. It is of interest whether there is a difference in PALS parameters between lamellar mesophase and dispersed colloidal systems, as nanostructure has been shown to be an important parameter in the release kinetics for drug delivery (i.e. intravenous vs. depot) and several workers have shown that the nanostructure of the phase impacts upon the release profile of the drug.38–40 To this end, the current study has compared the PALS parameters for the saturated lipids DLPC, DPPC and DSPC. Here the oPs lifetime in the organic region is consistently larger in the colloidal dispersions, suggesting that the hydrocarbon chains are more mobile within liposomes in excess water. In both forms τ4 increased above the main transition temperature, following the transition to Lα which is consistent with increased disorder of the hydrophobe region above Tm. Based upon the PALS lifetimes it may be anticipated that the diffusion of hydrophilic moieties (e.g. drugs) from the colloidal dispersion would be more rapid than the lamellar mesophase. However the correlation between diffusion-dependent transport and hydrocarbon chain mobility is more complicated, with the release rate also affected by the nature of the drug i.e. hydrodynamic radius, hydrogen bonding, hydrophilicity, and solubility, as well as host matrix chemical and mesophase structure.
In the lamellar mesophase systems, both I3 and I4 varied with temperature however, no such trend was observed in the dispersed systems. The oPs intensity in the organic phase (I4) was greatly reduced, while that in the aqueous region (I3) increased considerably for the liposomes. This is attributed to the abundance of water in the dispersed colloidal systems, resulting in a larger proportion of oPs annihilating in the aqueous region. The reduced oPs intensity in the organic region also resulted in larger uncertainty in data fitting for the corresponding oPs lifetime. A plot of I4versus the standard deviation of τ4 for the lamellar mesophase and dispersed colloidal systems of DPPC is presented in Fig. S9 in the (ESI†) section shows that reducing oPs intensity values (I4) results in increasing uncertainties for the corresponding oPs lifetime (τ4).
However, the total intensity values (Itotal = I3 + I4) between the lamellar mesophase and dispersed colloidal systems are not equal with the overall Itotal of the liposomal systems larger than the lamellar mesophases by approximately 6%, suggesting that a larger proportion of total positrons have annihilated in the dispersed colloidal liposomes of identical composition to the lamellar systems. In other words the morphology or nanostructure of the system plays a role, with the positronium annihilation efficiency greater in liposomes. This is most likely due to the greater surface area to volume ratio afforded by the colloidal dispersion compared to the lamellar mesophase.
Comparison of hydrocarbon chain packing between saturated and unsaturated lipids
The current study has compared the PALS parameters of colloidal dispersions of phospholipids with unsaturated hydrophobic chains (C16 and C18). It is well known that the introduction of unsaturation expands the lateral packing and disrupts the order of the hydrocarbon chain region. The unsaturated hydrophobes are highly ‘kinked’ due to their cis conformations and produce an increased chain volume relative to the saturated analogues.40,50 It was anticipated that the PALS parameters, in particular τ4, would be affected however, no significant difference between the PALS parameters of the saturated and unsaturated phospholipids was observed. A potential explanation for this is that the impact of lipid saturation upon oPs is small compared to the highly dynamic and mobile lipid chains that exist in the dispersed colloidal environment.
Conclusions
In the current systematic study we have demonstrated the sensitivity of the PALS parameters to variations in bilayer chemical structure and nanostructure (lamellar mesophase versus liposomes). We therefore suggest that PALS can assist the understanding of the dynamics, diffusion and permeability of biological cell membranes. The use of contemporary PALS experimental and analysis approaches can be deployed with confidence in future studies to probe effects of drug localization and transit on the properties of membranes as a new non-invasive approach to understand molecular level interactions in systems of biological and drug delivery relevance.4,6,51 This area provides a potentially exciting and fruitful application of PALS which is uniquely able to gauge diffusion and permeability properties on a nanosecond timescale that is on par with the timescales of these events. In-situ observation of the molecular mechanism of liposome–drug and drug–membrane interactions is desirable and will improve our understanding of drug encapsulation and release from materials from lipid self-assembly materials.
Acknowledgements
This research was supported under the Australian Research Council’s Discovery Projects funding scheme (project number DP0667189). This research was undertaken on the SAXS/WAXS beamline at the Australian Synchrotron, Victoria, Australia. AD thanks the Faculty of Pharmacy and Pharmaceutical Sciences for a postgraduate scholarship to conduct these studies, and thanks CSIRO for a top up scholarship. CJD was the recipient of an Australian Research Council (ARC) Federation Fellowship, and BJB is the recipient of an ARC Future Fellowship.
References
- R. S. Cantor, Lipid composition and the lateral pressure profile in bilayers, Biophys. J., 1999, 76, 2625–2639 CrossRef CAS.
- R. S. Cantor, Lateral pressure in cell membranes: a mechanism for modulation of protein function, J. Phys. Chem. B, 1997, 101, 1723–1725 CrossRef CAS.
- J. M. Seddon, Structure of the inverted hexagonal phase and non-lamellar phase transitions of lipids, Biochim. Biophys. Acta, 1990, 1031, 1–69 CrossRef CAS.
- W. T. Al-Jamal and K. Kostarelos, Liposomes: From a Clinically Established Drug Delivery System to a Nanoparticle Platform for Theranostic Nanomedicine, Acc. Chem. Res., 2011, 44, 1094–1104 CrossRef CAS PubMed.
- J. L. Arias, Liposomes in drug delivery: a patent review (2007-present), Expert Opin. Ther. Pat., 2013, 23, 1399–1414 CrossRef CAS PubMed.
- M. S. Muthu and S. S. Feng, Theranostic liposomes for cancer diagnosis and treatment: current development and pre-clinical success, Expert Opin. Drug Delivery, 2013, 10, 151–155 CrossRef CAS PubMed.
- B. A. Lewis, S. K. Das Gupta and R. G. Griffin, Solid-state NMR studies of the molecular dynamics and phase behavior of mixed-chain phosphatidylcholines, Biochemistry, 1984, 23, 1988–1993 CrossRef CAS.
- B. R. Lentz, Y. Barenholz and T. E. Thompson, Fluorescence depolarization studies of phase transitions and fluidity in phospholipid bilayers. 1. Single component phosphatidylcholine liposomes, Biochemistry, 1976, 15, 4521–4528 CrossRef CAS.
- D. P. Tieleman and S.-J. Marrink, Lipids Out of Equilibrium: Energetics of Desorption and Pore Mediated Flip-Flop, J. Am. Chem. Soc., 2006, 128, 12462–12467 CrossRef CAS PubMed.
- B. W. F. Drew, J. L. MacCallum and D. P. Tieleman, Thermodynamic Analysis of the Effect of Cholesterol on Dipalmitoylphosphatidylcholine Lipid Membranes, J. Am. Chem. Soc., 2009, 131, 1972–1978 CrossRef PubMed.
- I. V. Khavrutskii, A. A. Gorfe, B. Lu and J. A. McCammon, Free Energy for the Permeation of Na+ and Cl− Ions and Their Ion-Pair through a Zwitterionic Dimyristoyl Phosphatidylcholine Lipid Bilayer by Umbrella Integration with Harmonic Fourier Beads, J. Am. Chem. Soc., 2009, 131, 1706–1716 CrossRef CAS PubMed.
- H. J. Ache, Chemistry of the Positron and of Positronium, Angew. Chem., Int. Ed. Engl., 1972, 11, 179–199 CrossRef CAS.
- G. Dlubek, U. De, J. Pionteck, N. Y. Arutyunov, M. Edelmann and R. Krause-Rehberg, Temperature dependence of free volume in pure and silica-filled poly(dimethyl siloxane) from positron lifetime and PVT experiments, Macromol. Chem. Phys., 2005, 206, 827–840 CrossRef CAS.
- M. Schmidt and F. H. J. Maurer, Isotropic pressure-densified atactic poly(methyl methacrylate) glasses: Free-volume properties from equation-of-state data and positron annihilation lifetime spectroscopy, Macromolecules, 2000, 33, 3879–3891 CrossRef CAS.
- Y. C. Jean, J. D. Van Horn, W.-S. Hung and K.-R. Lee, Perspective of positron annihilation spectrosopy in polymers, Macromolecules, 2013, 46, 7133–7145 CrossRef CAS.
- F. Bockstahl and G. Duplatre, A new method to determine micellar aggregation numbers: positron annihilation lifetime spectroscopy, Phys. Chem. Chem. Phys., 2000, 2, 1–5 RSC.
- F. Bockstahl and G. Duplatre, Quantitative determination of a sodium dodecylsulfate micellar radius through positron annihilation lifetime spectroscopy, Phys. Chem. Chem. Phys., 1999, 1, 2767–2772 RSC.
- F. Bockstahl, E. Pachoud, G. Duplatre and I. Billard, Size of sodium dodecyl sulphate micelles in aqueous NaCl solutions as studied by positron annihilation lifetime spectroscopy, Chem. Phys., 2000, 256, 307–313 CrossRef CAS.
- G. Duplatre, M. F. Ferreira Marques and M. da Graca Miguel, Size of Sodium Dodecyl Sulfate Micelles in Aqueous Solutions as Studied by Positron Annihilation Lifetime Spectroscopy, J. Phys. Chem., 1996, 100, 16608–16612 CrossRef CAS.
- S. R. Choudhury, R. Yadav, A. N. Maitra and P. C. Jain, Structural transformations in CTAB aggregated systems investigated by positron lifetime spectroscopy 1. Binary systems, Colloids Surf., A, 1994, 82, 49–58 CrossRef.
-
S. R. Choudhury, R. Yadav, A. N. Maitra and P. C. Jain, Positron annihilation studies in ctab reversed micellar systems, in ICPA-8, ed. L. Dorikens-Vanpraet, M. Dorikens and D. Segers, World Scientific, Gent Belgium, 29 August–3 September, 1989, pp. 818–820.
- P. C. Jain, Positron annihilation spectroscopy and micellar systems, Adv. Colloid Interface Sci., 1995, 54, 17–54 CrossRef CAS.
- R. Yadav, K. C. Singh, S. R. Choudhury and P. C. Jain, Detection of bicontinuous phase in some reverse micellar systems by positron lifetime spectroscopy, Phys. Status Solidi C, 2007, 4, 3785–3788 CrossRef CAS.
- Y. C. Jean and H. J. Ache, Positronium reactions in micellar systems, J. Am. Chem. Soc., 1977, 99, 7504–7509 CrossRef CAS.
- Y.-C. Jean and H. J. Ache, Determination of critical micelle concentrations in micellar and reversed micellar systems by positron annihilation techniques, J. Am. Chem. Soc., 1978, 100, 984–985 CrossRef CAS.
- A. W. Dong, P. Pascual-Izarra, S. J. Pas, A. J. Hill, B. J. Boyd and C. J. Drummond, Positron Annihilation Lifetime Spectroscopy (PALS) as a Characterisation Technique for Nanostructured Self-Assembled Amphiphile Systems, J. Phys. Chem. B, 2009, 113, 84–91 CrossRef CAS PubMed.
- C. Pascual-Izarra, A. W. Dong, S. J. Pas, A. J. Hill, B. J. Boyd and C. J. Drummond, Advanced fitting algorithms for analysing positron annihilation lifetime spectra, Nucl. Instrum. Methods Phys. Res., Sect. A, 2009, 603, 456–466 CrossRef CAS PubMed.
-
P. C. Jain, Positron annihilation in liquid crystalline materials, in Positron annihilation, ed. P. C. Jain, R. M. Singru and K. P. Gopinathan, World scientific publishing Co., 1985, pp. 692–695 Search PubMed.
- K. C. Singh, On the study of liquid crystalline materials using positron annihilation spectroscopy, Phys. Status Solidi C, 2009, 6, 2482–2486 CrossRef CAS.
- A. W. Dong, C. Fong, A. J. Hill, B. J. Boyd and C. J. Drummond, Probing the amphiphile micellar to hexagonal phase transition using Positron Annihilation Lifetime Spectroscopy, J. Colloid Interface Sci., 2013, 402, 173–179 CrossRef CAS PubMed.
- D. R. Gustafson, Positronium formation in muscle: an investigation of the structure of cell water, Biophys. J., 1970, 10, 316–322 CrossRef CAS.
- Y. Y. Wang and Y. C. Jean, Biological applications of positron annihilation, Stud. Phys. Theor. Chem., 1988, 57, 353–378 CAS.
-
G. Graf, E. D. Handel, P. L. McMahon and J. C. Glass, Biochemical Applications of the Positrion Annihilation Techniques, in Positronium and Muonium Chemistry, ed. H. J. Ache, ACS Press, Washington D.C, 1979, pp. 67–87 Search PubMed.
- Y. C. Jean and A. J. Hancock, Positron lifetime studies on phase transitions of phospholipids, J. Chem. Phys., 1982, 77, 5836–5839 CrossRef CAS PubMed.
- A. E. McGrath, C. G. Morgan and G. K. Radda, Positron lifetimes in phospholipid dispersions, Biochim. Biophys. Acta, Biomembr., 1977, 466, 367–372 CrossRef CAS.
- P. L. McMahon, G. Graf and J. C. Glass, The phase transition in phospholipid vesicles probed by the positron annihilation lifetime technique, Phys. Lett. A, 1979, 72, 384–386 CrossRef.
- S. Baluch, J. Cirak and P. Balgavy, A Biomembrane Phase Transition by positron Annihilation Lifetime Method, Stud. Biophys., 1990, 136, 65–70 CAS.
- B. J. Boyd, D. V. Whittaker, S. M. Khoo and G. Davey, Hexosomes Formed From Glycerate Surfactants - Formulation as a Colloidal Carrier for Irinotecan, Int. J. Pharm., 2006, 318, 154–162 CrossRef CAS PubMed.
- B. J. Boyd, D. V. Whittaker, S.-M. Khoo and G. Davey, Lyotropic lqiuid crystalline phases formed from glycerate surfactants as sustained release drug delivery systems, Int. J. Pharm., 2006, 309, 218–226 CrossRef CAS PubMed.
- C. Fong, T. Le and C. J. Drummond, Lyotropic liquid crystal engineering-ordered nanostructured small molecule amphiphile self-assembly materials by design, Chem. Soc. Rev., 2012, 41, 1297–1322 RSC.
-
R. R. C. New, Liposomes: A Practical Approach, in The Practical Approach Series, ed. D. Rickwood and B. D. Hames, Oxford University Press, New York, 1990, pp. 301 Search PubMed.
-
A. W. Dong, Application of Positron Annihilation Lifetime Spectroscopy (PALS) to the Characterization of Nanostructure in Ordered Self-assembled Systems, PhD thesis, Monash University,
2012.
- P. D. Pascual-Izarra, A. W. Dong, S. J. Pas, A. J. Hill, B. J. Boyd and C. J. Drummond, Advanced fitting algorithms for analysing positron annihilation lifetime spectra, Nucl. Instrum. Methods Phys. Res., Sect. A, 2009, 603, 456–466 CrossRef PubMed.
- K. Kotera, T. Saito and T. Yamanaka, Measurement of positron lifetime to probe the mixed molecular states of liquid water, Phys. Lett. A, 2005, 345, 184–190 CrossRef CAS PubMed.
- N. M. Kirby, S. T. Mudie, A. M. Hawley, D. J. Cookson, H. D. T. Mertens, N. Cowieson and V. Samardzic-Boban, A low-background-intensity focusing small-angle X-ray scattering undulator beamline, J. Appl. Crystallogr., 2013, 46, 1670–1680 CrossRef CAS.
- R. N. A. H. Lewis, N. Mak and R. N. McElhaney, A differential scanning calorimetric study of the thermotropic phase behavior of model membranes composed of phosphatidylcholines containing linear saturated fatty acyl chains, Biochemistry, 1987, 26, 6118–6126 CrossRef CAS.
- A. S. Ulrich, M. Sami and A. Watts, Hydration of DOPC bilayers by differential scanning calorimetry, Biochim. Biophys. Acta, Biomembr., 1994, 1191, 225–230 CrossRef CAS.
-
http://avantilipids.com
.
- P. Sane, E. Salonen, E. Falck, J. Repakova, F. Tuomisto, J. M. Holopainen and I. Vattulainen, Probing Biomembranes with Positrons, J. Phys. Chem. B, 2009, 113, 1810–1812 CrossRef CAS PubMed.
- D. Wells, C. Fong and C. J. Drummond, Nonionic Urea Surfactants: Formation of Inverse Hexagonal Lyotropic Liquid Crystalline Phases by Introducing Hydrocarbon Chain Unsaturation, J. Phys. Chem. B, 2006, 110, 12660–12665 CrossRef CAS PubMed.
- A. L. Petersen, A. E. Hansen, A. Gabizon and T. L. Andresen, Liposome imaging agents in personalized medicine, Adv. Drug Delivery Rev., 2012, 64, 1417–1435 CrossRef CAS PubMed.
Footnote |
† Electronic supplementary information (ESI) available: Containing generalised phase diagrams for the systems studied, cryoTEM images of liposomes, PALS data for the various systems as referred to in the text, and the relationship between uncertainties in positronium intensities and lifetime values. See DOI: 10.1039/c4cp03822c |
|
This journal is © the Owner Societies 2015 |
Click here to see how this site uses Cookies. View our privacy policy here.