DOI:
10.1039/C4AN01346H
(Paper)
Analyst, 2015,
140, 287-294
Binding studies and anion-selective electrodes with neutral isophthalamide-based receptors†
Received
23rd July 2014
, Accepted 7th October 2014
First published on 7th October 2014
Abstract
Two acyclic isophthalamide-based hosts have been synthesised and their anion binding properties have been evaluated by 1H-NMR titrations. Different binding modes have been detected for the series of tested anions. The attachment of aminomethylpyrrole groups resulted in an improved binding selectivity. Additionally, the receptors have been incorporated as ionophores in plasticised polymeric membrane-based anion-selective electrodes. The potentiometric studies were in agreement with the NMR experiments and revealed a good sensing ability, considering the structural simplicity of the receptors and their interactions purely based on hydrogen bonding. These preliminary experiments have revealed an interesting selectivity towards highly hydrophilic anions such as fluoride and sulfate. Moreover, a particularly low detection limit (9 × 10−7 M) has been determined for the fluoride anion.
Introduction
The topic of anion recognition has reached its maturity within the area of supramolecular chemistry.1,2 Throughout the last decades a vast diversity of anion receptors has been explored.3–7 Despite the rich variety of reported binding units, there are certain building blocks which, due to their structural and chemical properties, have become authentic corner stones in the supramolecular chemistry of anions. This is the case of the easily accessible isophthalamide unit. Since Crabtree and co-workers firstly reported the employment of simple acyclic isophthalamide derivatives as neutral hosts for halide recognition,8,9 that building block has been extensively incorporated into acyclic,10 macrocyclic11,12 and more intricate structures.13 Additionally, the 1,3-relative arrangement of the amide groups has inspired the synthesis of related anion receptors with different aromatic and heteroaromatic spacers.14–17 Interestingly, the easy derivatisation of the plain isophthalamide core has rendered many examples which illustrate how slight structural alterations can have a remarkable influence in the binding ability of the receptor.18–20 In this regard, our interest in the design of pyrrole-based receptors21–25 has led us to combine the isophthalamide fragment with pyrrole units which can contribute to expand and enhance the original binding cavity.
Focusing on the application of the isophthalamide motif, beyond the elemental anion recognition studies, recently, it has also been applied to a different discipline of the supramolecular chemistry of anions, playing the role of transmembrane anion transporters.26–32 Nevertheless, it is quite surprising that among all the studies reported about isophthalamide derivatives, which demonstrate their great potential as anion receptors, these compounds have scarcely ever been studied in plasticised polymeric membrane ion-selective electrodes (ISEs).33,34 The incorporation of synthetic ionophores into the membrane represents a useful strategy for the improvement of selectivity in ion-selective electrodes. Additionally, the development of ionophores for anion-selective electrodes is a challenging goal to enhance their analytical application.35,36 This becomes specially interesting when targeting hydrophilic anions in the Hofmeister series, since these species have to overcome the enthalpically unfavourable phase transfer from an aqueous solution into the electrode membrane. Thus, herein we report the synthesis and anion complexation studies of two neutral isophthalamide derivatives, as well as, the incorporation of these molecules as ionophores in anion-selective electrodes. These devices, fabricated with structurally simple isophthalamides, have rendered good results in terms of the response selectivity and sensibility towards highly hydrophilic anions such as fluoride and sulfate.
Results and discussion
Outlined in Scheme 1 is the synthesis of receptors 1 and 2. Treatment of 5-(tert-butyl)isophthalic acid with N,N′-carbonyldiimidazole (CDI) in N,N′-dimethylformamide (DMF), followed by addition of n-butylamine afforded the desired receptor 1. Additionally, receptor 2 was synthesised by reaction of the corresponding 5-(tert-butyl)isophthaloyl dichloride with 2-aminomethylpyrrole in the presence of triethylamine. Compounds 1 and 2 were fully characterised by NMR and HRMS analyses.
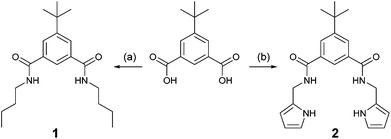 |
| Scheme 1 Synthesis of receptors 1 and 2. (a) CDI, n-butylamine, DMF, r.t.; (b) (i) SOCl2; (ii) 2-aminomethylpyrrole, Et3N, DMF, r.t. | |
These isophthalamide-based hosts define an internal binding cavity whose number of available hydrogen-bond donor sites increase from three, in the case of 1, to five, in 2. In order to evaluate the binding affinities of receptors 1 and 2 towards a range of anionic guests, 1H-NMR titration experiments were carried out with species of different geometries, namely spherical (F−, Cl−), angular (AcO−, PhO−) and tetrahedral (H2PO4−, SO42−)37 and DMF-d7 was chosen as solvent to work under a competitive environment.
Both receptors displayed qualitatively similar responses. In general, all the hydrogen bond donor groups, namely the amide and pyrrole NHs as well as the aromatic CH at position 2 in the central ring, experienced a downfield shift due to the deshielding effect resulting from the interaction with the anionic guests. As expected, receptor 2 yielded more stable complexes as a consequence of the presence of the two appended pyrrole units, which supported the suitability of the designed molecule.
Association constants were determined by non-linear fitting of the experimental binding isotherm and are summarised in Table 1.38 Most of the titrated anions formed complexes with a 1
:
1 stoichiometry as it could be assessed by the method of continuous variation, Job plot (Fig. 1).39
Table 1 Anion binding parameters for receptors 1 and 2a
Host |
Parameter |
F−b |
Cl− |
AcO− |
BzO− |
H2PO4− |
SO42− |
Determined in DMF-d7 following the amide NH chemical shift, [Host] = 4 × 10−3 M, 293 K; error < 10% in all cases.
K
11 and K12.
The titration isotherm could not be accurately fitted due to a too strong interaction (see ESI).
|
1
|
K
a (M−1) |
880 |
73 |
185 |
153 |
115 |
1040b |
480 |
670 |
Δδ (ppm) |
2.13 |
0.71 |
1.55 |
1.51 |
1.23 |
2.28 |
2
|
K
a (M−1) |
935 |
124 |
683 |
472 |
1330 |
—c |
124 |
Δδ (ppm) |
2.18 |
0.55 |
1.54 |
1.65 |
1.48 |
1.74 |
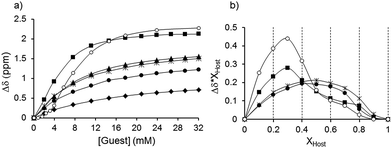 |
| Fig. 1 (a) Titration isotherms and (b) Job plots of 1. ■ F−, ◆ Cl−, ▲ AcO−, * PhCOO−, ● H2PO4−, ○ SO42−, following the NHamide. | |
Anyhow, it is worth highlighting the particular behaviour observed in the titrations with the fluoride anion (Fig. 2). Not surprisingly, the signals corresponding to the amide (and pyrrolic NHs in the case of compound 2) shifted downfield in the course of the titration experiment. Nevertheless, the signal assigned to the CH in position 2 of the benzene ring, which initially shifted downfield, experiences the opposite displacement towards lower chemical shifts when a slight excess of fluoride had been added. Simultaneously, the protons in the positions 4 and 6, which started shifting upfield, moved to higher chemical shifts. This two-phase titration curves are normally due to a change in the binding mode of the studied system.
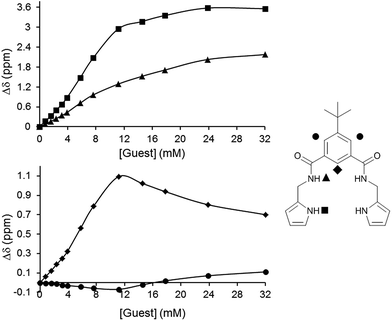 |
| Fig. 2 TBAF titration isotherms monitoring different nuclei from 2. | |
It is known that isophthalamide structures can adopt different conformations with a relative stability that follows the trend: syn–anti > syn–syn > anti–anti.11,40 In this case, the first aliquots of the fluoride anion caused an induced fit effect which oriented the amide groups according to a syn–syn conformation, in which all hydrogen bond donor groups converged to bind the anion. The excess of fluoride anion can force the isophthalamide receptors to adopt an anti–anti conformation where two equivalents of the anion would be accommodated. This conformational change perfectly correlates with the evolution of the 1H-NMR signals previously described. Moreover, a maximum located at a molar fraction value of 0.3 could be detected in the Job plot (Fig. 1) which confirmed the 1
:
2 (H
:
G) stoichiometry of the final complex. A similar behaviour was also evidenced for the sulfate complexation by receptor 1.
Concerning the cases with 1
:
2 (H
:
G) stoichiometry, in agreement with the measured binding constants (K11 > K12) these responses correspond to a multiple equilibria system with negative allosteric cooperativity. As a result, when the 1
:
1 stoichiometry prevails, the anion is bound by three and five hydrogen bonds in the receptors 1 and 2 respectively. In certain cases, the excess of anion induces a conformational change leading to 1
:
2 complexes where each anion is stabilised by two or three hydrogen bonds (Fig. 3). Accordingly, assuming that solvation effects are not relevant, a decrease in the enthalpy and the entropy of the system results in a thermodynamically unfavourable evolution from the 1
:
1 to the 1
:
2 complex.
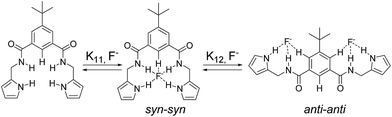 |
| Fig. 3 Proposed binding modes for the complexation equilibria with fluoride. | |
Due to the interest in developing anion-selective electrodes, particularly in the case of highly hydrophilic anions such as sulfate and fluoride, the anion binding affinity displayed by 1 and 2 encouraged us to further develop a sensing device through the incorporation of the receptors into plasticised membrane electrodes. Most of the examples of molecular receptors used as ionophores in plasticised polymeric membranes for the determination of anionic analytes are based on metalorganic compounds.41 The strong dative bond from a Lewis acid–base interaction between the metal centre and the anionic species represents the driving force which influences the ion-exchange equilibrium happening in the membrane. Thus, neutral receptors have less frequently been explored as anion ionophores in ISEs.42–46 Nevertheless the combination of several weak interacting sites, adequately arranged in the structure of the receptor, can contribute to an enhanced binding selectivity as it happens in biological systems.
In this regard, plasticised polymeric membranes with different weight percentage compositions (Table 2) were prepared as described in the experimental section with polyvinyl chloride (PVC) as a polymeric matrix, 2-nitrophenyloctyl ether (NPOE) as a plasticiser, receptors 1 or 2 as ionophores and tridodecylmethylammonium chloride (TDMACl) as an ionic additive.
Table 2 Optimisation of membrane composition (w/w)a
Membrane |
PVC |
NPOE |
Receptor 1a |
Receptor 2a |
TDMACl |
Ionophore/TDMACl molar ratio is given in brackets.
|
A |
33 |
66 |
1.0 |
|
|
B |
33 |
66 |
|
|
1.0 |
C |
32.7 |
65.5 |
0.8 (1.4) |
|
1.0 |
D |
32.6 |
65.3 |
1.1 (2.0) |
|
1.0 |
E |
32.4 |
64.7 |
1.9 (3.3) |
|
1.0 |
F |
32.6 |
65.1 |
|
1.3 (2.0) |
1.0 |
Among the different available plasticisers,47–49 the choice of NPOE arises from its high dielectric constant, which prevents the formation of ion-paired species in the membrane and correlates well with the previously described binding experiments performed in solution. NPOE is commonly used when working with membranes based on hydrogen-bond forming ionophores.50,51
The presence of ionic additives, which promote the ion-exchange process, plays a critical role in the optimisation of the response for ionophore-based ion-selective electrodes.52 In our case, it was observed that a membrane containing the ionophore and no cationic additive (membrane A) showed only a weak response towards all the tested anions (perchlorate, salicylate, thiocyanate, iodide, nitrate, bromide, chloride, fluoride, sulfate, acetate, dihydrogenphosphate, oxalate and hydrogen carbonate anions). In contrast, when a cationic additive was incorporated (membrane D) a good potential response was observed, as shown in Fig. 4a and b for chloride and fluoride, respectively.
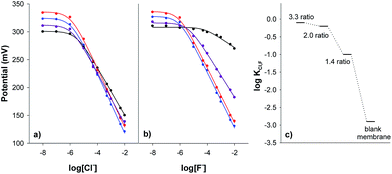 |
| Fig. 4 Calibration graphs for different molar concentrations of (a) chloride and (b) fluoride using membranes with different ionophore-to-ionic ratios (membrane B: black; C: purple; D: blue; E: red). (c) Plot of the logarithmic selectivity coefficients for different ionophore-to-ionic additive molar ratios. | |
The influence of the ionophore-to-ionic additive molar ratio on the potentiometric response of the membranes was studied by keeping the amount of ionic additive constant in the membrane and varying the amounts of receptor 1, to cover a molar ratio range between 0 and 3.3 (membranes B–E).
As can be seen in Fig. 4a and b, the total span of the potential response for chloride and fluoride increased with the ionophore-to-ionic additive ratio up to a molar ratio of 2.0, remaining almost constant beyond that point. This effect was more noticeable for fluoride than for chloride and it was manifested by an increase in the selectivity coefficient KpotCl,J (Fig. 4c). In other words, this change in the coefficient indicated an increase in the selectivity for fluoride versus chloride. This fact was in good agreement with the complexation constant values found for receptor 1 with these anions. Taking all these results into account an ionophore/TDMACl molar ratio of 2.0 was used for further studies.
Calibration plots with membranes B, D and F were obtained for all the studied anions (Fig. 5). The blank membrane (membrane B) revealed the expected Hofmeister trend dictated by the lipophilicity of the anion. Nevertheless, the incorporation of isophthalamide receptor 1 or 2 into the electrode membrane (membranes D and F) altered this order and the magnitude of the total potential response span. A significant enhancement in the potentiometric response was detected for the fluoride anion, in the case of ISEs containing receptor 1 and for fluoride and sulfate anions in the case of receptor 2.
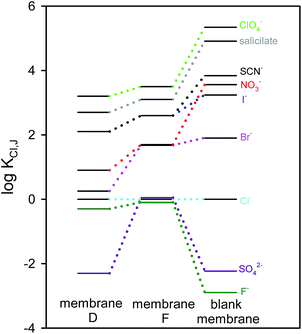 |
| Fig. 5 Plot of the logarithmic selectivity coefficients obtained for membranes containing receptor 1 or receptor 2 (membranes D and F respectively) and for the blank membrane (membrane B). | |
It is worth highlighting the difficulty in potentiometrically sensing the fluoride anion with plasticised polymeric membranes due to its high hydration energy,53 which reinforces the adequacy of the isophthalamide derivatives as neutral ionophores.
Additionally, the response of membranes containing either of two ionophores towards hydroxyl anions was also obtained by using sodium hydroxide. No potentiometric response was observed up to a concentration 5 × 10−4 M. From this concentration a small potential change was found (20 to 30 mV) for the maximum concentration assayed 10−2 M.
This screening of anionic species enabled the calculation of the selectivity coefficients54 by the separate solutions method for equal concentration of primary (chloride) and interfering anions (J) (Fig. 5 and Table S1 in the ESI†).55 Due to the frequent use of chloride as the primary ion in the literature, we adopted this criterion for comparative purposes.
As observed in Fig. 5, the most relevant change in the selectivity of the electrodes containing isophthalamide receptors, when compared to the blank membrane, corresponded to fluoride, whose selectivity over chloride increased about 500-fold. Regarding receptor 2 the response for sulfate and dihydrogenphosphate anions, produced an increase in the selectivity over chloride of about 200-fold and 10-fold respectively. Calibration parameters obtained for fluoride and sulfate anions are shown in Table 3.
Table 3 Potentiometric response characteristicsa
Parameter |
Membrane D |
Membrane F |
Fluoride |
Fluoride |
Sulfate |
Mean value ± SD (n = 3).
Time required to reach 95% of equilibrium potential at different anion concentrations. This time was measured for all the points in the calibration plot.
|
Total span (mV) |
200 |
164 |
220 |
Slopea (mV dec−1) |
−51.7 ± 0.2 |
−47.3 ± 0.1 |
−31.1 ± 0.2 |
Linear range (M) |
10−5 to 10−2 |
10−5 to 10−2 |
5 × 10−5 to 10−2 |
Detection limita (M) |
(9.0 ± 0.3) × 10−7 |
(1.7 ± 0.2) × 10−6 |
(2.5 ± 0.2) × 10−5 |
Response timeb (s) |
<10 |
<11 |
<12 |
Interestingly, a fast response time was obtained for both electrodes (see time trace in ESI†). The electrode with receptor 1 as the ionophore displayed a higher slope and a lower detection limit for fluoride than the electrode with receptor 2. Moreover, the fabricated electrodes displayed a linear response over three concentration decades. Additionally, a detection limit of 9.0 × 10−7 M could be determined from these preliminary studies, which is slightly lower than that commonly described for commercially available electrodes based on lanthanum fluoride membranes. Recently, several fluoride ionophores mainly based on metalorganic complexes Al and Zr have been published in the literature.56–63 These compounds can bind fluoride by forming dative-covalent bonds with the metal centres. Despite the good selectivity claimed for these compounds as potentiometric sensors, the reported limits of detection do not reach the sensitivity achieved by the isophthalamide-based ISEs. This highlights the goodness of our results, which have been obtained with ionophores that exclusively interact through hydrogen bonds in plasticised membrane electrodes showing a highly selective profile towards fluoride, a very good limit of detection and a rapid response time. These characteristics point to real application opportunities, i.e. determination of fluoride in mouthwashes, toothpastes64 and certain dietary supplements65 and food stuffs,66,67 but this purpose escapes from the scope of the present work.
Moreover, a significant response was obtained for the electrode with receptor 2, which showed a near-Nernstian behaviour over 2.5 concentration decades for the very hydrophilic sulfate anion. It is worth recalling the difficulty in detecting anions with high hydration energy using ion-selective electrodes, due to the biphasic transfer of the analyte that governs the functioning of these devices. This hydrophilicity is the reason why the electrode with receptor 1 did not detect the sulfate anion. The Gibbs free energies of sulfate and fluoride hydration are −1080 kJ mol−1 and −465 kJ mol−1 respectively.68 In agreement with the previously discussed NMR experiments, receptor 1 had very similar binding constants for fluoride and sulfate. Thus, the anion hydration becomes an energy barrier for the sulfate to be sensed by the electrode. Accordingly, the hydration energy also justifies the results obtained for the electrode with receptor 2. Although a better interaction could be qualitatively determined for sulfate over fluoride by NMR titration experiments, the higher hydration energy of sulfate makes more difficult its transfer to the plasticised membrane. Nevertheless, the very strong interaction determined for receptor 2 with sulfate, still makes possible that this anion can be detected by the electrode. Only few examples of sulfate-selective electrodes have been reported in the literature using synthetic ionophores (either metalorganic,69 positively charged70 or neutral71–76). Regarding the electrode fabricated with receptor 2, the wide concentration range with a Nernstian response, the low detection limit and the fast response time (Table 3) are features that support its suitability to be applied for the determination of the sulfate anion.
Conclusion
The synthesis of isophthlamide based receptors with different numbers of hydrogen bond donor sites has been described. Anion binding experiments in DMF solution revealed a preferential complexation of fluoride, dihydrogenphosphate and sulfate with different binding modes being detected by 1H-NMR. The incorporation of these isophthalamide derivatives as ionophores in ion-selective electrodes has been described. A study on potentiometric anion sensing, exclusively based on hydrogen bond interactions, has revealed a good selectivity and sensitivity towards the fluoride anion, and the sulfate anion in the case of receptor 2. These results prove the adequacy of isophthalamide-based receptors to work as ionophores for the preparation of anion-selective electrodes. A detection limit lower than the commercially available fluoride ion-selective electrodes based on the lanthanum fluoride membrane has been achieved.
Experimental section
General
Reagents used as starting materials were commercially available and were used without further purification. Solvents were dried following the usual protocols. Unless stated otherwise, all reactions were carried out under nitrogen atmosphere. Column chromatography was run with silica gel 60 A CC 70–200 μm as the stationary phase and using HPLC grade solvents. Melting points were measured in a Reichert instrument and are not corrected. 1H-NMR and 13C-NMR experiments were recorded on either a Bruker AV300 or AV 400 instrument. Chemical shifts are referred to the residual peak of the solvent and are given in ppm. J values are given in Hz. Mass spectrometry was recorded on a HPLC-MS TOF 6220 instrument. Potentiometric measurements were recorded using a homemade high-impedance data acquisition 16-channel box connected to a PC by USB. An Orion Ag/AgCl double-junction reference electrode (Orion 90-02) containing 10−2 M KCl in the outer compartment was also used. Polyvinyl chloride (PVC) of high molecular weight, 2-nitrophenyl octyl ether (NPOE), tridodecylmethylammonium chloride (TDMACl) and tetrahydrofuran (THF) were purchased from Fluka. Perchlorate, salicylate, thiocyanate, iodide, nitrate, bromide, chloride, sulfate, acetate, dihydrogenphosphate, fluoride and hydrogen carbonate solutions were prepared by dissolving the corresponding sodium salts in Milli-Q water.
Synthetic procedure
5-(tert-Butyl)-N,N′-dibutylisophthalamide, 1.
Over a stirred solution of 5-(tert-butyl)isophthalic acid (0.4 g, 1.8 mmol) in anhydrous dimethylformamide (30 mL), under nitrogen atmosphere, N,N′-carbonyldiimidazole (1.17 g, 7.2 mmol) was added. The resulting mixture was stirred at room temperature for 3 hours. Next, freshly distilled n-butylamine (0.89 mL, 9.0 mmol) was added dropwise and the reaction mixture was then stirred at room temperature for 8 hours. The solvent was removed under reduced pressure and the residue was treated with n-hexane. The resulting precipitate was filtered and dried under vacuum. The isolated white solid corresponds to the desired pure compound 1 (0.48 g, 80%). Mp 125–127 °C. 1H-NMR δ (300 MHz, CDCl3) 0.89–0.94 (6H, m), 1.30–1.40 (13H, m), 1.5–1.6 (4H, m), 3.35–3.55 (4H, m), 6.7 (2H, br s, NHamide), 7.90–8.00 (3H, m); 13C-NMR δ (75 MHz, CDCl3) 13.8 (2×CH3), 20.1 (2×CH2), 31.1 (3×CH3), 31.6 (2×CH2), 34.9 (Cq), 39.9 (2×CH2), 122.3 (CH), 127.1 (2×CH), 134.6 (2×Cq), 152.4 (Cq), 167.4 (2×C
O). HRMS-(m/z) for C20H33N2O2, found 333.2538 (M + H)+, calcd: 333.2537.
5-(tert-Butyl)-N,N′-(pyrrol-2-ylmethyl)-isophthalamide, 2.
A solution of 5-(tert-butyl)isophthalic acid (0.6 g, 2.7 mmol) in SOCl2 (60 mL) was refluxed for 20 hours under nitrogen atmosphere. Then, the reaction mixture was allowed to cool to room temperature and the remaining SOCl2 was evaporated under reduced pressure. The resulting residue was washed three times with dry toluene and concentrated under vacuum. Following, the residue was dissolved in dry dichloromethane (20 mL) and added dropwise over a stirred solution of 2-aminomethylpyrrole (0.65 g, 6.75 mmol) and freshly distilled triethylamine (1.76 mL, 13.50 mmol) in dry dichloromethane (15 mL) under nitrogen atmosphere. The reaction mixture was stirred under these conditions for 8 h. The solvent was removed under vacuum and the residue was purified by column chromatography on silica gel eluting with ethyl acetate–dichloromethane (1
:
2) to isolate 2 as a white solid (0.61 g, 60%). Mp 113–116 °C. 1H-NMR δ (300 MHz, DMSO-d6) 1.33 (9H, s), 4.42 (4H, d, J = 5.4), 5.91–5.93 (4H, m), 6.62–6.65 (2H, m), 8.01 (2H, s), 8.18 (1H, s), 8.85 (2H, t, J = 5.4, NHamide), 10.57 ppm (2H, br s, NHpyrrole); 13C-NMR δ (75 MHz, DMSO-d6) 31.0 (3×CH3), 34.8 (Cq), 36.3 (2×CH2), 105.9 (2×CH), 107.2 (2×CH), 117.1 (2×CH), 123.9 (CH), 126.7 (2×CH), 128.9 (2×Cq), 134.3 (2×Cq), 150.9 (Cq), 166.0 (2×C
O). HRMS-(m/z) for C22H27N4O2, found 379.2116 (M + H)+, calcd: 379.2129.
Titration experiments
A stock solution of the corresponding receptor was prepared in deuterated dimethylformamide with an estimated water content of 0.15% v/v and [Host] = 4 × 10−3 M. The anions, used as tetrabutylammonium salts, were then dissolved with the appropriate volume of the former solution to obtain the correct concentration of the titrant. Aliquots of the latter solution were added to the solution which contained the receptor, without having to consider any dilution effects on the titrated species.
Membranes and electrodes preparation
Membranes summarised in Table 2 were prepared by dissolving appropriate amounts of the corresponding membrane components (PVC, NPOE, ionophore and ionic additive) in 3 mL of THF. This solution was poured into a Fluka glass ring (inner diameter 28 mm, height 30 mm) and allowed to settle overnight until total evaporation of THF had occurred, to obtain a thin plastic membrane. A 6 mm diameter piece was cut out with a punch for ion-selective membranes and incorporated into a Fluka electrode body ISE containing 1 × 10−4 M KCl as internal filling solution. The electrodes were conditioned in water until they reached a constant potential. When not in use, electrodes were kept immersed in water.
Potentiometric measurements
Dynamic calibrations of the electrodes were made by adding, while stirring, small aliquots of the corresponding standard solution of the anion, used as sodium salts, to cover the concentration range from 1 × 10−7 to 1 × 10−2 M. The electrode was conditioned in water until the original potential was recovered before each new calibration. If the base line did not fully reach that value, the water was renewed. The steady-state potentials were plotted versus logarithmic values of the corresponding concentrations. When the potentiometric response for the anion was of the Nikolsky–Eisenman type, data were fitted to the equation: | E = E0 + S log(CA + DL) | (1) |
where E0 is the standard potential of the cell, S is the calibration slope, CA is the concentration of the corresponding anion and DL is the detection limit. When the potentiometric response for the anion displayed an initial super-Nernstian behaviour, only the data in the linear range (Nernstian response) were fitted to a linear function, and the limit of detection was calculated using the extended definition given by IUPAC as the measured potential which deviates S
log
2 from the extrapolated Nernstian response.77 All potentiometric measurements were performed at room temperature in non-buffered solutions. From pH measurements of these solutions it was checked that all the anions assayed are almost entirely as in the mentioned anionic form.
Logarithmic selectivity coefficients were calculated applying the separate solution method.55 The potentials (E) obtained at the same concentration (10−2 M) of primary (chloride) and interfering anions (J) were substituted in eqn (2):
| 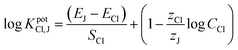 | (2) |
where
SCl is the calibration slope for chloride,
zCl and
zJ are the ionic charges of the anions and
CCl is 10
−2 M.
Acknowledgements
M.M.-M., D.C. and A.T. are grateful to the Ministerio de Ciencia y Tecnología (project CTQ2011-27175) and Fundación Séneca (project 04509/GERM/06). M.M.-M. also acknowledges Ministry of Education for a FPU fellowship. M.C. thanks the University of Murcia for a FPU-UMU fellowship. M.C., M.S.G. and J.A.O. gratefully acknowledge the financial support of the Ministerio de Ciencia y Tecnología, Spain (project CTQ2011-27049).
Notes and references
-
J. Seesler, P. A. Gale and W.-S. Cho, Anion Receptor Chemistry, Royal Society of Chemistry, Cambridge, 2006 Search PubMed.
-
A. Bianchi, K. Bowman-James and E. García-España, Supramolecular Chemistry of Anions, Wiley-VCH, New York, 1997 Search PubMed.
- P. A. Gale, N. Busschaert, C. J. E. Haynes, L. E. Karagiannidis and I. L. Kirby, Chem. Soc. Rev., 2014, 43, 205–241 RSC.
- L. E. Santos-Figueroa, M. E. Moragues, E. Climent, A. Agostini, R. Martínez-Máñez and F. Sancenón, Chem. Soc. Rev., 2013, 42, 3489–3613 RSC.
- P. Dydio, D. Lichosyt and J. Jurczak, Chem. Soc. Rev., 2011, 40, 2971–2985 RSC.
- M.-O. M. Piepenbrock, G. O. Lloyd, N. Clarke and J. W. Steed, Chem. Rev., 2010, 110, 1960–2004 CrossRef CAS PubMed.
- For monographic issues about the supramolecular chemistry of anionic species see: Chem. Soc. Rev., 2010, 39(10), 3595–4003 Search PubMed;
Coord. Chem. Rev., 2006, 250(23–24), 2918–3244 Search PubMed;
Coord. Chem. Rev, 2003, 240(1–2), 3–221 Search PubMed.
- K. Kavallieratos, C. M. Bertao and R. H. Crabtree, J. Org. Chem., 1999, 64, 1675–1683 CrossRef CAS PubMed.
- K. Kavallieratos, S. R. de Gala, D. J. Austin and R. H. Crabtree, J. Am. Chem. Soc., 1997, 119, 2325–2326 CrossRef CAS.
- P. A. Gale, Acc. Chem. Res., 2006, 39, 465–475 CrossRef CAS PubMed.
- M. J. Chmielewski and J. Jurczak, Chem.–Eur. J., 2006, 12, 7652–7667 CrossRef CAS PubMed.
- M. A. Hossain, J. M. Llinares, D. Powell and K. Bowman-James, Inorg. Chem., 2001, 40, 2936–2937 CrossRef CAS.
- M. D. Lankshear and P. D. Beer, Acc. Chem. Res., 2007, 40, 657–668 CrossRef CAS PubMed.
- T. Zieliński, M. Kedziorek and J. Jurczak, Chem.–Eur. J., 2008, 14, 838–846 Search PubMed.
- S. O. Kang, J. M. Llinares, D. Powell, D. VanderVelde and K. Bowman-James, J. Am. Chem. Soc., 2003, 125, 10152–10153 CrossRef CAS PubMed.
- S. Camiolo, P. A. Gale, M. B. Hursthouse, M. E. Light and C. N. Warriner, Tetrahedron Lett., 2003, 44, 1367–1369 CrossRef CAS.
- P. A. Gale, S. Camiolo, G. J. Tizzard, C. P. Chapman, M. E. Light, S. J. Coles and M. B. Hursthouse, J. Org. Chem., 2001, 66, 7849–7853 CrossRef CAS PubMed.
- G. W. Bates, P. A. Gale and M. E. Light, Chem. Commun., 2007, 2121–2123 RSC.
- S.-i. Kondo, Y. Hiraoka, N. Kurumatani and Y. Yano, Chem. Commun., 2005, 1720–1722 RSC.
- A. Szumna and J. Jurczak, Eur. J. Org. Chem., 2001, 2001, 4031–4039 CrossRef.
- D. Curiel, G. Sánchez, C. Ramírez de Arellano, A. Tárraga and P. Molina, Org. Biomol. Chem., 2012, 10, 1896–1904 CAS.
- D. Curiel, G. Sánchez, M. Más-Montoya, A. Tárraga and P. Molina, Analyst, 2012, 137, 5499–5501 RSC.
- D. Curiel, M. Más-Montoya, G. Sánchez, R. A. Orenes, P. Molina and A. Tárraga, Org. Biomol. Chem., 2010, 8, 4811–4814 CAS.
- D. Curiel, A. Espinosa, M. Más-Montoya, G. Sánchez, A. Tárraga and P. Molina, Chem. Commun., 2009, 7539–7541 RSC.
- D. Curiel, A. Cowley and P. D. Beer, Chem. Commun., 2005, 236–238 RSC.
- C. R. Yamnitz, S. Negin, I. A. Carasel, R. K. Winter and G. W. Gokel, Chem. Commun., 2010, 46, 2838–2840 RSC.
- J. T. Davis, O. Okunola and R. Quesada, Chem. Soc. Rev., 2010, 39, 3843–3862 RSC.
- G. W. Gokel and N. Barkey, New J. Chem., 2009, 33, 947–963 RSC.
- P. V. Santacroce, J. T. Davis, M. E. Light, P. A. Gale, J. C. Iglesias-Sánchez, P. Prados and R. Quesada, J. Am. Chem. Soc., 2007, 129, 1886–1887 CrossRef CAS PubMed.
- X. Li, B. Shen, X.-Q. Yao and D. Yang, J. Am. Chem. Soc., 2007, 129, 7264–7265 CrossRef CAS PubMed.
- P. A. Gale, J. Garric, M. E. Light, B. A. McNally and B. D. Smith, Chem. Commun., 2007, 1736–1738 RSC.
- A. P. Davis, D. N. Sheppard and B. D. Smith, Chem. Soc. Rev., 2007, 36, 348–357 RSC.
- A. K. Jain, J. Raisoni, R. Kumar and S. Jain, Int. J. Environ. Anal. Chem., 2007, 87, 553–563 CrossRef CAS.
- A. K. Jain, V. K. Gupta and J. R. Raisoni, Talanta, 2006, 69, 1007–1012 CrossRef CAS PubMed.
- P. Bühlmann, E. Pretsch and E. Bakker, Chem. Rev., 1998, 98, 1593–1688 CrossRef PubMed.
-
P. Bühlmann and L. D. Chen, in Supramolecular Chemistry from Molecules to Nanomaterials, John Wiley & Sons, Ltd, 2012, pp. 2539–2579 Search PubMed.
- NMR titrations were performed with the anions as tetrabutylammonium salts.
- M. J. Hynes, J. Chem. Soc., Dalton Trans., 1993, 311–312 RSC.
-
K. A. Connors, Binding Constants: The Measurements of Molecular Complex Stability, 1987 Search PubMed.
- C. A. Hunter and D. H. Purvis, Angew. Chem., Int. Ed. Engl., 1992, 31, 792–795 CrossRef.
- E. Bakker, P. Bühlmann and E. Pretsch, Electroanalysis, 1999, 11, 915–933 CrossRef CAS.
- M. Cuartero, M. Más-Montoya, M. Soledad García, D. Curiel and J. A. Ortuño, Talanta, 2014, 123, 200–206 CrossRef CAS PubMed.
- V. K. Gupta, A. K. Jain, M. K. Pal and A. K. Bharti, Electrochim. Acta, 2012, 80, 316–325 CrossRef CAS PubMed.
- M. Cuartero, J. A. Ortuño, M. S. García, G. Sánchez, M. Más-Montoya and D. Curiel, Talanta, 2011, 85, 1876–1881 CrossRef CAS PubMed.
- M. J. Berrocal, A. Cruz, I. H. A. Badr and L. G. Bachas, Anal. Chem., 2000, 72, 5295–5299 CrossRef CAS.
- S. Amemiya, P. Bühlmann, Y. Umezawa, R. C. Jagessar and D. H. Burns, Anal. Chem., 1999, 71, 1049–1054 CrossRef CAS PubMed.
- M. A. A. de los Arada Pérez, L. P. Marín, J. C. Quintana and M. Yazdani-Pedram, Sens. Actuators, B, 2003, 89, 262–268 CrossRef.
- W. S. Gibbons and R. P. Kusy, Thermochim. Acta, 1996, 284, 21–45 CrossRef CAS.
- M. A. Simon and R. P. Kusy, J. Biomed. Mater. Res., 1996, 30, 313–320 CrossRef CAS PubMed.
- E. M. Zahran, Y. Hua, Y. Li, A. H. Flood and L. G. Bachas, Anal. Chem., 2010, 82, 368–375 CrossRef CAS PubMed.
- K. P. Xiao, P. Bühlmann, S. Nishizawa, S. Amemiya and Y. Umezawa, Anal. Chem., 1997, 69, 1038–1044 CrossRef CAS.
- U. Schaller, E. Bakker, U. E. Spichiger and E. Pretsch, Anal. Chem., 1994, 66, 391–398 CrossRef CAS.
- Y. Marcus, J. Chem. Soc., Faraday Trans., 1991, 87, 2995–2999 RSC.
- Bearing in mind that in order to avoid biased vaues, selectivity coefficients should not be calculated for anions which do not show a Nernstian response. The coefficients for fluoride and dihydrogenphosphate in the blank membrane have been estimated for comparative purposes only.
- Y. Umezawa, P. Bühlmann, K. Umezawa, K. Tohda and S. Amemiya, Pure Appl. Chem., 2000, 72, 1851–2082 CrossRef CAS.
- A. Matusevich, M. Pietrzak and E. Malinowska, Sens. Actuators, B, 2012, 168, 62–73 CrossRef CAS PubMed.
- L. Górski, A. Matusevich, P. Parzuchowski, I. Luciuk and E. Malinowska, Anal. Chim. Acta, 2010, 665, 39–46 CrossRef PubMed.
- L. Górski, M. Mroczkiewicz, M. Pietrzak and E. Malinowska, Anal. Chim. Acta, 2009, 633, 181–187 CrossRef PubMed.
- M. Pietrzak, M. E. Meyerhoff and E. Malinowska, Anal. Chim. Acta, 2007, 596, 201–209 CrossRef CAS PubMed.
- K. Wojciechowski, W. Wroblewski, J. Przygorzewska, G. Rokicki and Z. Brzozka, Chem. Anal., 2002, 47, 335–346 CAS.
- E. Steinle, U. Schaller and M. E. Meyerhoff, Anal. Sci., 1998, 14, 79–84 CrossRef CAS.
- S. Chandra, A. Ruzicka, P. Svec and H. Lang, Anal. Chim. Acta, 2006, 577, 91–97 CrossRef CAS PubMed.
- Y. Kang, C. Lutz, S. A. Hong, D. Sung, J. S. Lee, J. H. Shin, H. Nam, G. S. Cha and M. E. Meyerhoff, Bull. Korean Chem. Soc., 2010, 31, 1901 Search PubMed.
- R. Pérez-Olmos, J. C. Soto, N. Zárate and I. Díez, J. Pharm. Biomed. Anal., 2008, 47, 170–176 CrossRef PubMed.
- L. Singer and R. H. Ophang, J. Agric. Food Chem., 1986, 34, 510–513 CrossRef CAS.
- M. K. Malde, K. Bjorvatn and K. Julshamn, Food Chem., 2001, 73, 373–379 CrossRef CAS.
- S. M. Levy and G. Muchow, Am. J. Public Health, 1992, 82, 281–284 CrossRef CAS PubMed.
- Y. Marcus, J. Chem. Soc., Faraday Trans., 1991, 87, 2995–2999 RSC.
- M. Shamsipur, M. Yousefi, M. Hosseini, M. R. Ganjali, H. Sharghi and H. Naeimi, Anal. Chem., 2001, 73, 2869–2874 CrossRef CAS.
- M. Fibbioli, M. Berger, F. P. Schmidtchen and E. Pretsch, Anal. Chem., 2000, 72, 156–160 CrossRef CAS.
- S. Firouzabadi, I. Razavinapanah, R. Zhiani, M. Ghanei-Motlagh and M. R. Salavati, Monatsh. Chem., 2013, 144, 113–120 CrossRef CAS.
- A. Sathyapalan, A. Zhou, T. Kar, F. Zhou and H. Su, Chem. Commun., 2009, 325–327 RSC.
- V. V. Egorov, V. A. Nazarov, E. B. Okaev and T. E. Pavlova, J. Anal. Chem., 2006, 61, 382–388 CrossRef CAS.
- M. J. Berrocal, A. Cruz, I. H. A. Badr and L. G. Bachas, Anal. Chem., 2000, 72, 5295–5299 CrossRef CAS.
- Z.-Q. Li, G.-D. Liu, L.-M. Duan, G.-L. Shen and R.-Q. Yu, Anal. Chim. Acta, 1999, 382, 165 CrossRef CAS.
- S. Nishizawa, P. Bühlmann, K. P. Xiao and Y. Umezawa, Anal. Chim. Acta, 1998, 358, 35 CrossRef CAS.
- E. Lindner and Y. Umezawa, Pure Appl. Chem., 2008, 80, 85–104 CrossRef CAS.
Footnotes |
† Electronic supplementary information (ESI) available: 1H-NMR spectra, 13C-NMR spectra, titration isotherms and Job plots of 2, and fit plots for the NMR titrations. See DOI: 10.1039/c4an01346h |
‡ Current address: Department of Inorganic, Analytical, and Applied Chemistry, University of Geneva, 1211, Geneva 4, Switzerland. |
|
This journal is © The Royal Society of Chemistry 2015 |
Click here to see how this site uses Cookies. View our privacy policy here.