Sr3GdNa(PO4)3F:Eu2+,Mn2+: a potential color tunable phosphor for white LEDs†
Received
17th September 2013
, Accepted 22nd October 2013
First published on 23rd October 2013
Abstract
A series of Eu2+ and Mn2+ activated novel Sr3GdNa(PO4)3F phosphors have been prepared through a high temperature solid state reaction. The investigation revealed that Sr3GdNa(PO4)3F crystallized in a hexagonal crystal system with the space group P
(no. 147). The Eu2+ activated phosphors can be efficiently excited in the range of 250 to 420 nm, which matches well with the commercial n-UV LED chips, and give intense blue emission centering at 470 nm. By codoping the Eu2+ and Mn2+ ions into the SGNPF host and singly varying the doping content of the Mn2+ ion, tunable colors from blue to white and then to yellow are obtained in SGNPF:Eu2+,Mn2+ phosphors under the irradiation of 390 nm. The energy transfer from the Eu2+ to Mn2+ ions is demonstrated to be a dipole–quadrupole mechanism in terms of the experimental results and analysis of photoluminescence spectra and decay curves of the phosphors. The critical distance between the Eu2+ and Mn2+ ions in SGNPF:Eu2+,Mn2+ was determined by the spectral overlap method. The investigation indicates that our prepared samples might have potential application in WLEDs.
1. Introduction
Compared with the traditional incandescent and fluorescent lamps, white light emitting diodes (WLEDs) possess merits of high efficiency, long lifetime, and environmental friendliness and have become another trend for general illumination.1–4 The phosphor converted WLEDs (pc-WLEDs) which are comprised of blue or near ultraviolet chips and down converting phosphors are common in use due to advantages of good color stability and reproducibility.5,6 Until recently, the commercial pc-WLED was dominated by the combination of an InGaN chip with a Y3Al5O12:Ce3+ (YAG:Ce) phosphor. However, this kind of WLED suffers from problems of low color rendering index and high correlated color temperature (CCT) due to the deficiency of the red component.7,8 To improve the properties of WLEDs, several methods have been adopted. One strategy is to explore novel phosphors with high efficiency, high rendering index, and low CCT. Recently, Daicho et al. reported a yellow emitting (Ca1−x−y,Srx,Euy)7(SiO3)6Cl2 phosphor with high quantum efficiency.9 Li et al. have synthesized the yellow emitting Ba0.93Eu0.07Al2O4 phosphor with low CCT.10 The method of blending tricolor (blue, green, red) phosphors upon the UV chips was also employed to obtain warm-white LEDs. This combination can produce warm-white light with excellent color rendering index; however the luminescent efficiency is a trade-off and color aberration occurs easily due to emission reabsorption and different degradation rates of the three-primary phosphors, respectively.11–13 In recent years, single component phosphors realized by energy transfer from the sensitizer to the activator in a proper host have attracted significant interest due to their good luminescence efficiency, color reproducibility and low manufacturing costs. For example, Xia et al. reported the blue-green Ca2Al3O6F:Ce3+,Tb3+ phosphor;14 Lv et al. have synthesized the BaMg2Al6Si9O30:Eu2+, Tb3+, Mn2+ phosphor;15 Liu et al. have reported the Eu2+ and Mn2+ codoped KCaY(PO4)2 phosphor.16
Apatite is a structural type for compounds with the general formula M10(XO4)6L2 (M = Ca, Sr, Ba; X = P, As, V, Mn, Cr, Si and Ge; L = F, Cl, OH). It has been known since Naray-Szabo determined the structure of Ca10(PO4)6F2 in 1930 and was widely studied due to its biological and industrial importance.17–20 Strontium is one of the common substituents in apatite and its presence has a great value in geology and materials science. The luminescence properties of Sr3GdNa(PO4)3F containing rare earth ions with a similar apatite structure have not been studied. In this paper, we synthesized the novel Sr3GdNa(PO4)3F:Eu2+,Mn2+ phosphor by a solid state reaction and studied its luminescence properties and the energy transfer from the Eu2+ to Mn2+ ions. Tunable colors including white were obtained by codoping the Eu2+ and Mn2+ ions into Sr3GdNa(PO4)3F.
2. Experimental section
2.1. Materials and synthesis
A series of Sr3(1−m−n)Eu3mMn3nGdNa(PO4)3F (SGNPF:mEu2+,nMn2+, m = 0.00–0.05, n = 0.01–0.06) phosphors were synthesized by a conventional high temperature solid-state reaction. SrCO3 (A.R.), SrF2 (A.R.), Gd2O3 (A.R.), Na2CO3 (A.R.), (NH4)2HPO4 (A.R.), Eu2O3 (99.99%), and MnCO3 (A.R.) were used as starting materials and were weighed in a proper stoichiometric ratio with 20% excess of SrF2 for the loss of fluorine. The raw materials were mixed thoroughly by grinding in an agate mortar for 15 min. The powder mixtures were first heated at 600 °C for 2 h and then at 1000 °C for 3 h under a reductive atmosphere (20% H2 + 80% N2). Finally, the prepared phosphors were cooled to room temperature and reground for further measurements.
2.2. Measurements and characterization
The powder X-ray diffraction (XRD) measurements were performed on a D8 Focus diffractometer (Bruker) operating at 40 kV and 40 mA with Cu Kα radiation (λ = 1.5418 Å). The scanning rate was fixed at 10° min−1 with 2θ ranging from 15° to 80°. The surface morphology of the prepared phosphor was inspected with a field emission scanning electron microscope equipped with an energy-dispersive spectrometer (EDS) (FE-SEM, S-4800, Hitachi, Japan). The photoluminescence excitation (PLE) and photoluminescence (PL) spectra were recorded on a Hitachi F-4500 spectrophotometer equipped with a 150 W Xenon lamp as the excitation source. The diffuse-reflectance spectra were obtained using a Hitachi U-4100 spectrophotometer with the reflection of black felt (reflection 3%) and white Al2O3 (reflection 100%). The luminescence decay curves were obtained from a Lecroy Wave Runner6100 Digital Oscilloscope (1 GHz) using a tunable laser (pulse width = 4 ns, gate = 50 ns) as the excitation source. The above measurements were performed at room temperature. The study of thermal stability was performed on an Edinburgh Instrument FLS 920 spectrophotometer with the advanced research system InC as the temperature controlling system.
3. Results and discussion
3.1 Phase identification and crystal structure
The powder X-ray diffraction of our prepared samples has been performed to verify their phase purity. Fig. 1(a) depicts the representative XRD patterns of our prepared phosphors together with the standard data of Sr3(La,Ce)Na(PO4)3(F,OH) (JCPDS 50-1595) for comparison. The patterns of the obtained samples cannot be indexed to any gadolinium compound reported in the database of the Joint Committee on Powder Diffraction Standards (JCPDS). However, we found that all the diffraction peaks of the sample can be well indexed to the standard data of JCPDS 50-1595 except for a small shift to the higher 2θ angle, indicating that the prepared phosphor is isostructural with Sr3(La,Ce)Na(PO4)3(F,OH). The energy dispersive spectrum of SGNPF shown in Fig. 1(c) reveals the presence of Sr, Gd, Na, P, O, and F in the host. Their proportion was determined to be Sr
:
Gd
:
Na
:
P
:
O
:
F = 3.01
:
1
:
1.07
:
2.98
:
10.82
:
0.83. This result is basically in agreement with the theoretical value of Sr3GdNa(PO4)3F, confirming the formation of the molecular formula. In addition, considering the stoichiometric ratio of the raw material we used during the preparation, it can be deduced that the obtained samples have the formula of Sr3GdNa(PO4)3F. The small shift of the diffraction angle should be ascribed to the smaller Gd3+ ions substituting for the larger La3+/Ce3+ ions. Sr3GdNa(PO4)3F crystallized in a hexagonal crystal system with the space group P
(no. 147). From Fig. 1(a), we can also see that the doped Eu2+ and Mn2+ ions do not cause any significant change to the structure and all the obtained samples are of phase purity. Considering the ionic radii and valence state of the cations,21 we suggest that the Eu2+ and Mn2+ ions will prefer to substitute for the Sr2+ ions. The morphology of the prepared Sr3GdNa(PO4)3F has been studied by FE-SEM. From the micrograph shown in Fig. 1(c), we can see that the prepared samples consist of particles with size ranging from 1 to 3 μm, which can meet the requirement of WLED phosphors.
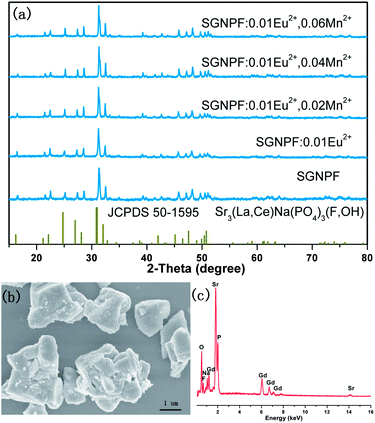 |
| Fig. 1 (a) XRD patterns of the typical prepared phosphors. The standard data for Sr3(La,Ce)Na(PO4)3(F,OH) (JCPDS 50-1595) are shown as references. (b) SEM image of the SGNPF host. (c) EDX spectrum of the SGNPF host. | |
To obtain the detailed crystal information of the prepared samples, Rietveld structure refinements of SGNPF and SGNPF:0.01Eu2+ samples were performed using the general structure analysis system (GSAS) program.22 The refinement patterns of SGNPF and SGNPF:0.01Eu2+ are depicted in Fig. 2(a) and (b), while their final structural parameters are shown in Table S1 and S2 (ESI†), respectively. The reliability factors χ2 = 3.009, Rwp = 4.24%, Rp = 3.22% for SGNPF and χ2 = 3.139, Rwp = 3.76%, Rp = 2.80% for SGNPF:0.01Eu2+ all indicate that atom positions, fraction factors, and temperature factors of the samples well satisfy the reflection conditions. The cell parameters of Sr3GdNa(PO4)3F were calculated to be a = b = 9.5728 Å, c = 7.1167 Å, and V = 564.795 Å3, similar to the cell parameters of the (Sr0.99Eu0.01)3GdNa(PO4)3F sample being a = b = 9.5749 Å, c = 7.1138 Å, and V = 564.804 Å3. This slight difference between the two samples in cell parameters can be explained by the small difference between Sr2+ and Eu2+ ions in ionic radii. The unit cell structure of SGNPF:0.01Eu2+ is shown in the inset of Fig. 2(b) together with the coordination environment of the Sr2+ ion. In the host, there is only one Sr site with six oxygen atoms and one fluorine atom coordinated. The bond length of Sr–F is 2.3704 Å and the average length of Sr–O is 2.6020 Å. Correspondingly, there will be one kind of Eu2+ emitting center generated by the substitution of Sr2+ ions in the SGNPF:0.01Eu2+ phosphor.
 |
| Fig. 2 The experimental (crosses) and calculated (red solid line) powder XRD patterns of the (a) SGNPF and (b) SGNPF:0.01Eu2+ samples. The blue solid lines represent the difference between experimental and calculated data and the green sticks mark the Bragg reflection positions. The inset of Fig. 2(b) shows the unit cell structure of SGNPF:0.01Eu2+ and the coordination environment of the Sr2+ ions. | |
3.2 Photoluminescence properties of the SGNPF:Eu2+ phosphor
Fig. 3 shows the normalized excitation and emission spectra of the SGNPF:0.01Eu2+ phosphor together with the reflection spectra of SGNPF and SGNPF:0.01Eu2+ samples. The excitation spectrum monitored at 470 nm shows a broad band ranging from 250 to 420 nm, which matches well with the commercial n-UV LED chips (360–410 nm). The broad excitation spectrum of SGNPF:0.01Eu2+ is caused by the electron transition from the 4f7 ground state to different excited states of the Eu2+ ions, which can be further validated by the diffuse reflection spectra of SGNPF and SGNPF:0.01Eu2+ samples. Compared with SGNPF, the SGNPF:0.01Eu2+ phosphor shows a strong absorption band ranging from 220 to 420 nm caused by the electron transition of Eu2+ ions. The SGNPF:0.01Eu2+ phosphor exhibits an intense blue emission which extends from 410 to 550 nm with the maximum at 470 nm due to the 4f65d1 → 4f7 transition of the Eu2+ ions. The shapes and peak wavelengths of the emission spectra are exactly the same under the excitation of 300, 350, and 390 nm, indicating that there is only one kind of Eu2+ emission center substituting for the Sr2+ ions in the phosphor. This phenomenon is consistent with the previous structure discussion of Sr3GdNa(PO4)3F which contains only one kind of Sr site. In order to investigate the effect of doping concentration of Eu2+ ions on the emission intensity of the phosphor, a series of Eu2+ doped phosphors have been prepared. Fig. 4 shows the emission spectra of SGNPF:mEu2+ (m = 0.002–0.05) phosphors under the excitation of 390 nm. With the increase of Eu2+ concentration, the emission intensity of the phosphor increases gradually and reaches a maximum at m = 0.01, and then decreases due to concentration quenching. The inset of Fig. 4 depicts the variation of the emission intensity as a function of doped Eu2+ content.
 |
| Fig. 3 Excitation and emission spectra of the SGNPF:0.01Eu2+ phosphor (solid lines). Diffuse reflection spectra of SGNPF and SGNPF:0.01Eu2+ (dotted lines). | |
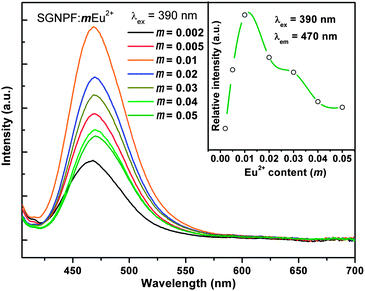 |
| Fig. 4 Emission spectra of SGNPF:mEu2+ (m = 0.002–0.05) phosphors. The inset shows the relationship between the emission intensity and doped Eu2+ content. | |
According to Dexter's energy transfer theory, concentration quenching is mainly caused by the non-radiative energy migration among the activators at high concentration due to the exchange interaction or multipole–multipole interaction.23,24 According to the report of Van Uiter, the emission intensity (I) per activator follows the equation25,26
| I/m = k[1 + β(m)θ/3]−1 | (1) |
where
I is the emission intensity of the activator,
m is the Eu
2+ concentration,
k and
β are constants for a given host in the same excitation condition,
θ = 3 stands for the energy transfer among the nearest neighbor ions while
θ = 6, 8, and 10 represent the dipole–dipole, dipole–quadrupole, and quadrupole–quadrupole interactions, respectively. By taking the logarithm of
eqn (1), the linear relationship between log(
I/
m) and log(
m) with a slope of (–
θ/3) was obtained. To get a correct
θ value, the dependence of log(
I/
m) on log(
m) was plotted and is shown in
Fig. 5 with the concentration of the Eu
2+ ions ranging from 0.02 to 0.05.
θ was calculated to be 4.593, which is located between 3 and 6, indicating that the dipole–dipole interaction and energy transfer among the nearest neighbor ions are both responsible for the concentration quenching of Eu
2+ ions.
 |
| Fig. 5 Dependence of log(I/m) on log(m) in SGNPF:mEu2+ phosphors. | |
3.3 Photoluminescence properties and energy transfer in the SGNPF:Eu2+,Mn2+ phosphor
It is well known that the Eu2+ ion is a good sensitizer for the Mn2+ ion and tunable colors can be obtained by codoping the two ions in a proper host.27–29 The Mn2+ singly doped phosphor has been synthesized and its PLE and PL spectra are shown in Fig. 6. The excitation spectrum of the Mn2+ ion singly doped phosphor shows several bands peaking at 314, 344, 408, and 501 nm due to the electron transition from the 6A1(6S) ground state to 4E (4D), 4T2(4D), [4A1(4G), 4E(4G)] and 4T1(4G) excited states, respectively.30,31 At the excitation of 410 nm, the SGNPFF:10Mn2+ phosphor shows a broad emission band centering at 580 nm due to the 4T1(4G) → 6A1(6S) transition. Since the d–d transition of the Mn2+ ions is spin and parity forbidden, the emission intensity of the SGNPF:Mn2+ phosphor is pretty low. Fig. S1† depicts the comparison between the PL spectrum of SGNPF:0.01Eu2+ and the PLE spectrum of the SGNPF:0.10Mn2+ phosphor. The broad spectral overlap in the range from 405 to 530 nm indicates that there is a great probability for the energy transfer from the Eu2+ to Mn2+ ions. Thus, the enhancement of the Mn2+ emission and tunable colors can be expected by codoping the Eu2+ and Mn2+ ions into the SGNPF host. Fig. 7 shows the photoluminescence excitation and emission spectra of the SGNPF:01Eu2+,0.02Mn2+ sample. At the excitation of 390 nm, the phosphor shows an emission spectrum including a blue emission band with the maximum at 470 and a yellow emission due to the electron transition of Eu2+ and Mn2+ ions, respectively. The excitation spectrum of SGNPF:0.01Eu2+,0.02Mn2+ monitored at 580 nm shows the excitation band of the Eu2+ ions, which validates the occurrence of energy transfer from the Eu2+ to Mn2+ ions. One can also see that there exists a difference between the excitation spectra monitored at 470 and 580 nm. This phenomenon may be ascribed to the effect of the Mn2+ ion on the crystal field around the Eu2+ ions. The doping of Mn2+ ions may change the environment of Eu2+ ions which are near the Mn2+ ions, resulting in the excitation spectrum monitored at 580 nm being different from that monitored at 470 nm.
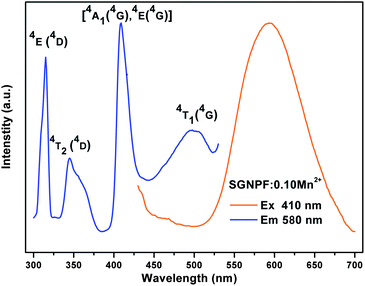 |
| Fig. 6 Excitation and emission spectra of the SGNPF:0.10Mn2+ phosphor. | |
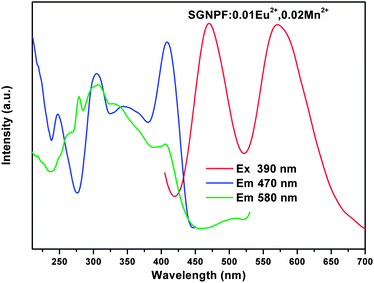 |
| Fig. 7 Excitation and emission spectra of SGNPF:0.01Eu2+,0.02Mn2+ phosphor. | |
In order to study the effect of doping concentration of Mn2+ ions on the luminescence properties of the phosphors, a series of SGNPF:0.01Eu2+,nMn2+ (n = 0.01–0.06) samples have been prepared. Fig. 8 demonstrates the emission spectra of the phosphors at the excitation of 390 nm. One can see that the emission intensity of the Eu2+ ions decreases monotonously with the increment of the Mn2+ content, while the emission intensity of the Mn2+ firstly increased to a maximum at n = 0.04 and then decreased due to concentration quenching. From Fig. 8, one can also see that the peak wavelengths of Mn2+ emission shift to longer wavelengths with an increase of the Mn2+ content from 0.01 to 0.06. This phenomenon can be ascribed to the enhancement of the crystal field surrounding the Mn2+ ions caused by the substitution of the Sr2+ ions by Mn2+ ions. Since the ionic radius of the Mn2+ ion is smaller than that of the Sr2+ ion, doping of Mn2+ will cause shrinkage of the unit cell volume and the length of the Mn–O will become shorter. This variation leads to the increment of the crystal field splitting and further results in the shifting of the Mn2+ emission to a longer wavelength.32,33
 |
| Fig. 8 Emission spectra of SGNPF:0.01Eu2+,nMn2+ phosphors with different Mn2+ contents. | |
To further investigate the energy transfer process, the photoluminescence decay curves of the Eu2+ ions in SGNPF:0.01Eu2+,nMn2+ phosphors were measured and depicted in a logarithmic intensity in Fig. 9. For the Eu2+ singly doped sample, the decay curves can be well fitted to the single exponential function which can be written as
| I(t) = I0 exp(−t/τ) | (2) |
where
I0 and
I(
t) are the emission intensities of Eu
2+ ions at time 0 and
t, respectively. On the basis of
eqn (2), the lifetime of the Eu
2+ ion in the SGNPF:0.01Eu
2+ phosphor was determined to be 497.1 ns. It is noticeable that the decay curves deviate from the single exponential function with the increment of the Mn
2+ content and this deviation becomes more and more obvious. That is to say the doping of the Mn
2+ ions has gradually modified the luminescence dynamics of the Eu
2+ ions due to the energy transfer from the Eu
2+ to Mn
2+ ions. The average lifetimes of the Eu
2+ ions can be defined as
|  | (3) |
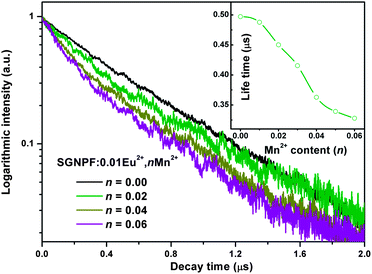 |
| Fig. 9 Decay curves for the Eu2+ emission in typical SGNPF:0.01Eu2+,nMn2+ phosphors (excited at 355 nm, monitored at 470 nm). The inset shows the relationship between the lifetime and doped Eu2+ content. | |
By using eqn (3), the decay lifetimes of the Eu2+ ions are calculated to be 487.8, 450.1, 415.4, 362.7, 339.2, and 327.8 ns for SGNPF:0.01Eu2+,nMn2+ phosphors with n = 0.01, 0.02, 0.03, 0.04, 0.05, and 0.06, respectively. The variation in life times for the Eu2+ ions is depicted in the inset of Fig. 9. The decrease of the decay time of the Eu2+ ion is caused by the non-radiative energy transfer from the Eu2+ to Mn2+ ions.
Non-radiative energy transfer between the Eu2+ and Mn2+ ions usually occurs as a result of exchange interaction or multipole–multipole interaction. According to Dexter's energy transfer theory, for the exchange interaction, the following relationship can be given:34,35
where
I and
I0 represent the luminescence intensities of the Eu
2+ ions in the presence and absence of the Mn
2+ ions.
C is the total doping concentration of the Eu
2+ and Mn
2+ ions. For the multipole–multipole interaction, the relationship between the luminescence intensity and the doping concentration can be approximately demonstrated as
31,34,35 |  | (5) |
In the above expression, α = 6, 8, and 10 corresponds to dipole–dipole, dipole–quadrupole, and quadrupole–quadrupole interactions, respectively. ln(I0/I) as a function of C and I0/I as a function of Cα/3 (α = 6, 8, 10) are presented in Fig. 10(a–d). It is obvious that a linear relationship can be observed only when α = 8, indicating that the electric dipole–quadrupole interaction is mainly responsible for the energy transfer from the Eu2+ to Mn2+ ions. For the dipole–quadrupole interaction mechanism, the critical distance (RC) for the energy transfer from the Eu2+ to Mn2+ ions can be calculated by the spectral overlap method:33
|  | (6) |
where
λS (in Å) is the wavelength peak of the Eu
2+ emission,
fq is the oscillator strength of the involved absorption transition of the acceptor (Mn
2+),
E (in eV) is the energy involved in the transfer, and
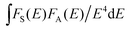
stands for the spectral overlap between the normalized photoluminescence spectra of the Eu
2+ emission
FS(
E) and the Mn
2+ excitation
FA(
E). For the Eu
2+ and Mn
2+ codoped SLNPF phosphor,
λs is 4700 Å,
fq is about 10
−10, and

was calculated to be 0.0254 eV. Correspondingly, the critical distance was determined to be 10.68 Å.
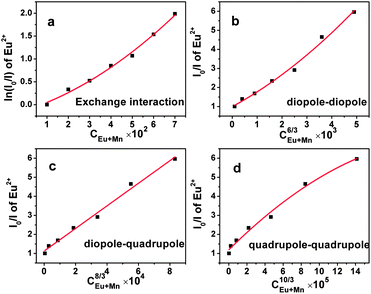 |
| Fig. 10 Dependence of (a) ln(I0/I) on C and I0/I on (b) C6/3, (c) C8/3, and (d) C10/3. | |
Thermal stability as an important character has also been investigated. Fig. 11 shows the normalized emission intensity of the SGNPF:0.01Eu2+,0.02Mn2+ phosphor at different temperatures. When the temperature reaches 423 K, the intensity decreases to 50.1% of the initial value at 298 K. The decrement of emission intensity with the increase of temperature can be ascribed to the temperature-dependent electron–phonon interaction, which can be explained by the schematic configuration coordinate diagram of Eu2+ ions as shown in the inset of Fig. 11. With the increase of temperature, the electron–phonon interaction increases, which further makes the electrons at the bottom of the 5d excited state shift to the junction of the excited and ground states and then relax to the 4f ground state in a nonradiative way.
 |
| Fig. 11 Normalized emission intensity of the SGNPF:0.01Eu2+,0.02Mn2+ phosphor at different temperatures. The inset shows the schematic configuration coordinate diagram of the Eu2+ ion. | |
The Commission Internationale de L'Eclairage (CIE) chromaticity coordinates of SGNPF:Eu2+,nMn2+ samples are calculated and summarized in Table 1. With the increase of the Mn2+ content, the CIE coordinates of the sample can vary from (0.143,0.160) to (0.465,0.428) due to the different emission components of the Eu2+ and Mn2+ ions resulting from the energy transfer from the Eu2+ to Mn2+ ions. The CIE coordinates of the phosphors together with the corresponding photographs are also depicted in Fig. 12. From this figure, we can clearly see that the color hue of the samples changes from blue to white and finally to yellow. The correlated color temperature (CCT) as one of the characteristics of the phosphor has also been calculated by using the approximate formula reported by McCamy:36T = −437n3 + 360n2 – 6861n + 5514.31, where n = (x − 0.3320)/(y − 0.1858). The results are also listed in Table 1, where one can clearly see that the CCT of the samples decreased greatly with the Mn2+ content varying from 0 to 0.06. At n = 0.03, the CCT of the phosphor decreases to 4140 K and a white color with a low correlated color temperature is obtained. The luminescence properties of Sr3GdNa(PO4)3F:Eu2+,Mn2+ phosphors indicate that the prepared samples may serve as single-component white emitting phosphors for UV excited WLEDs.
Table 1 CIE coordinates and correlated color temperatures of SGNPF:Eu2+,nMn2+ samples
Sample composition |
CIE coordinates (x,y) |
CCT (K) |
SGNPF:0.01Eu2+ |
(0.143,0.160) |
1 368 573 |
SGNPF:0.01Eu2+,0.01Mn2+ |
(0.262,0.283) |
12 630 |
SGNPF:0.01Eu2+,0.02Mn2+ |
(0.340,0.352) |
5197 |
SGNPF:0.01Eu2+,0.03Mn2+ |
(0.376,0.382) |
4140 |
SGNPF:0.01Eu2+,0.04Mn2+ |
(0.431,0.417) |
3146 |
SGNPF:0.01Eu2+,0.05Mn2+ |
(0.449,0.422) |
2871 |
SGNPF:0.01Eu2+,0.06Mn2+ |
(0.465,0.428) |
2673 |
 |
| Fig. 12 CIE coordinates and luminescent digital photographs of the prepared SGNPF:0.01Eu2+,nMn2+ samples. The inset shows the photographs of the samples under a 365 nm UV lamp. | |
4. Conclusions
In summary, Sr3GdNa(PO4)3F:Eu2+,Mn2+ phosphors have been synthesized and their structure and luminescence properties have been investigated in detail for the first time. The Sr3GdNa(PO4)3F host crystallizes in the P
space group with cell parameters a = b = 9.5728 Å, c = 7.1167 Å, V = 564.795 Å3, and Z = 2. The SGdNPF:Eu2+ phosphors can be efficiently excited in the wavelength range from 250 to 420 nm and have a strong blue emission ranging from 405 to 550 nm under an irradiation of 390 nm. By codoping the Eu2+ and Mn2+ ions into the host and utilizing the energy transfer of Eu2+ → Mn2+, CIE coordinates of the phosphors changed from (0.143,0.160) to (0.465,0.428) with corresponding color hues varying from blue to white then to yellow. In SGNPF:Eu2+,Mn2+ samples, energy transfer from the Eu2+ to Mn2+ ions is mainly resonant type via a dipole–quadrupole mechanism and the obtained critical distance for the doped ions is 10.68 Å by the spectral overlap method. Moreover, with the increase of Mn2+ content, the correlated color temperatures of the samples decreased significantly. Preliminary studies on the properties of the phosphor indicate that our prepared SGNPF:Eu2+,Mn2+ may have a potential value for WLEDs.
Acknowledgements
This work is financially supported by the National Natural Science Foundation of China (Grant no. 21271167) and the Fund for Creative Research Groups (Grant no. 21221061).
Notes and references
- T. Suehiro, N. Hirosaki and R.-J. Xie, ACS Appl. Mater. Interfaces, 2011, 3, 811 CAS.
- S.-K. Kim, J. W. Lee, H.-S. Ee, Y.-T. Moon, S.-H. Kwon, H. Kwon and H.-G. Park, Opt. Express, 2010, 18, 11025 CrossRef CAS PubMed.
- T.-C. Liu, B.-M. Cheng, S.-F. Hu and R.-S. Liu, Chem. Mater., 2011, 23, 3698 CrossRef CAS.
- Z.-y. Mao and D.-j. Wang, Inorg. Chem., 2010, 49, 4922 CrossRef CAS PubMed.
- L. Chen, C.-I. Chu and R.-S. Liu, Microelectron. Reliab., 2012, 52, 900 CrossRef CAS PubMed.
- S. Zhang, H. Liang and C. Liu, J. Phys. Chem. C, 2013, 117, 2216 CAS.
- C. Hecht, F. Stadler, P. J. Schmidt, J. r. S. auf der Günne, V. Baumann and W. Schnick, Chem. Mater., 2009, 21, 1595 CrossRef CAS.
- K. Y. Jung, H. W. Lee and H.-K. Jung, Chem. Mater., 2006, 18, 2249 CrossRef CAS.
- H. Daicho, T. Iwasaki, K. Enomoto, Y. Sasaki, Y. Maeno, Y. Shinomiya, S. Aoyagi, E. Nishibori, M. Sakata and H. Sawa, Nat. Commun., 2012, 3, 1132 CrossRef PubMed.
- X. Li, J. D. Budai, F. Liu, J. Y. Howe, J. Zhang, X.-J. Wang, Z. Gu, C. Sun, R. S. Meltzer and Z. Pan, Light: Sci. Appl., 2013, 2, e50 CrossRef.
- L. Chen, A. Luo, Y. Zhang, F. Liu, Y. Jiang, Q. Xu, X. Chen, Q. Hu, S.-F. Chen and K.-J. Chen, ACS Comb. Sci., 2012, 14, 636 CrossRef CAS PubMed.
- N. Guo, H. You, Y. Song, M. Yang, K. Liu, Y. Zheng, Y. Huang and H. Zhang, J. Mater. Chem., 2010, 20, 9061 RSC.
- W. Lü, N. Guo, Y. Jia, Q. Zhao, W. Lv, M. Jiao, B. Shao and H. You, Inorg. Chem., 2013, 52, 3007 CrossRef PubMed.
- Z. Xia, R.-S. Liu, K.-W. Huang and V. Drozd, J. Mater. Chem., 2012, 22, 15183 RSC.
- W. Lü, Z. Hao, X. Zhang, Y. Luo, X. Wang and J. Zhang, Inorg. Chem., 2011, 50, 7846 CrossRef PubMed.
- W.-R. Liu, C.-H. Huang, C.-W. Yeh, J.-C. Tsai, Y.-C. Chiu, Y.-T. Yeh and R.-S. Liu, Inorg. Chem., 2012, 51, 9636 CrossRef CAS PubMed.
- I. Mayer, R. Roth and W. Brown, J. Solid State Chem., 1974, 11, 33 CrossRef CAS.
- J. F. Rakovan and J. M. Hughes, Can. Mineral., 2000, 38, 839 CrossRef CAS.
- M. Xie and R. Pan, Opt. Mater., 2013, 35, 1162 CrossRef CAS PubMed.
- C. Zhang, S. Huang, D. Yang, X. Kang, M. Shang, C. Peng and J. Lin, J. Mater. Chem., 2010, 20, 6674 RSC.
- R. D. Shannon, Acta Crystallogr., Sect. A: Cryst. Phys., Diffr., Theor. Gen. Cryst., 1976, 32, 751 CrossRef.
- A. C. Larson and R. B. Von Dreele, Los Alamos Natl. Lab., 1994, 1–221 Search PubMed.
- Y. Jia, H. Qiao, Y. Zheng, N. Guo and H. You, J. Phys. Chem. C, 2012, 14, 3537 CAS.
- D. L. Dexter, J. Chem. Phys., 1953, 21, 836 CrossRef CAS.
- W. Lü, Z. Hao, X. Zhang, X. Wang and J. Zhang, Opt. Mater., 2011, 34, 261 CrossRef PubMed.
- L. Van Uitert, J. Lumin., 1971, 4, 1 CrossRef CAS.
- N. Guo, Y. Huang, H. You, M. Yang, Y. Song, K. Liu and Y. Zheng, Inorg. Chem., 2010, 49, 10907 CrossRef CAS PubMed.
- J. S. Kim, P. E. Jeon, J. C. Choi, H. L. Park and S. I. Mho, Appl. Phys. Lett., 2004, 84, 2931 CrossRef CAS.
- Y.-H. Won, H. S. Jang, W. B. Im, D. Y. Jeon and J. S. Lee, Appl. Phys. Lett., 2006, 89, 231909 CrossRef.
- T.-S. Chan, R.-S. Liu and I. Baginskiy, Chem. Mater., 2008, 20, 1215 CrossRef CAS.
- C.-H. Huang, T.-W. Kuo and T.-M. Chen, ACS Appl. Mater. Interfaces, 2010, 2, 1395 CAS.
- L. Shi, Y. Huang and H. J. Seo, J. Phys. Chem. A, 2010, 114, 6927 CrossRef CAS PubMed.
- H. You, J. Zhang, G. Hong and H. Zhang, J. Phys. Chem. C, 2007, 111, 10657 CAS.
- D. L. Dexter and J. A. Schulman, J. Chem. Phys., 1954, 22, 1063 CrossRef CAS.
- G. Blasse, Philips Res. Rep., 1969, 24, 131 CAS.
- C. S. McCamy, Color Res. Appl., 1992, 17, 142 CrossRef.
Footnote |
† Electronic supplementary information (ESI) available. See DOI: 10.1039/c3tc31837k |
|
This journal is © The Royal Society of Chemistry 2014 |
Click here to see how this site uses Cookies. View our privacy policy here.