DOI:
10.1039/C4SC01629G
(Edge Article)
Chem. Sci., 2014,
5, 3617-3626
Thiosquaramides: pH switchable anion transporters†
Received
2nd June 2014
, Accepted 17th June 2014
First published on 1st July 2014
Abstract
The transport of anions across cellular membranes is an important biological function governed by specialised proteins. In recent years, many small molecules have emerged that mimick the anion transport behaviour of these proteins, but only a few of these synthetic molecules also display the gating/switching behaviour seen in biological systems. A small series of thiosquaramides was synthesised and their pH-dependent chloride binding and anion transport behaviour was investigated using 1H NMR titrations, single crystal X-ray diffraction and a variety of vesicle-based techniques. Spectrophotometric titrations and DFT calculations revealed that the thiosquaramides are significantly more acidic than their oxosquaramide analogues, with pKa values between 4.0 and 9.0. This led to the observation that at pH 7.2 the anion transport ability of the thiosquaramides is fully switched OFF due to deprotonation of the receptor, but is completely switched ON at lower pH.
Introduction
Recent interest in the development of small molecule lipid bilayer anion transporters has been driven by the potential application of these compounds to treat diseases caused by faulty anion transport across cell membranes.1–5 Cystic fibrosis is the most well-known example of this type of disease, that are known collectively as “channelopathies” and are characterised by faulty chloride transport channels.6 Compounds that can restore or disrupt such chemical processes in cells also have the potential to possess anti-cancer properties, where they can depolarize acidic compartments within cells and trigger apoptosis.7–10 Therefore one of the goals of our research recently has been to develop new classes of anion transporters and to learn what features of these molecules enable them to effectively mediate the flux of anions through lipid bilayers. Previously, we discovered that thiourea groups, particularly those with a single aromatic group pendant to the thiourea, are significantly more effective anion transporters than analogous ureas.11 A subsequent QSAR analysis of a series of similar thioureas showed that for this class of molecule, lipophilicity, molecular size and the Hammett constant of the substituents present on the transporter are the key molecular parameters upon which the transport efficiency of the molecule depends.12 We have also been interested in squaramide based receptors, as squaramides have been suggested as potent (thio)urea isosteres13–19 with potential therapeutic benefits against a variety of diseases (e.g. Chagas disease).20,21 We found that transporters containing squaramide groups (such as compounds 1–3) could mediate higher fluxes of anions than analogous ureas or thioureas despite having lower lipophilicities.22 In this case the significantly higher anion affinities of squaramides (as compared to urea and thiourea analogues) may be an important factor. As we had observed with other classes of transporter,23–25 increasing the degree of fluorination of the squaramides resulted in significantly enhanced transport properties.22
We envisaged that thiosquaramides should have both enhanced acidity compared to squaramides and higher lipophilicity. To test this hypothesis we have conducted the first systematic supramolecular study of thiosquaramides. Specifically, we synthesised thiosquaramides 5–8 and compared their chloride complexation and transport properties to squaramides 1–4. We find that the enhanced acidity of the NH groups results in thiosquaramide deprotonation under physiological conditions, with little chloride transport across lipid bilayers. However, under acidic conditions some thiosquaramides become active transporters. This unusual pH-switch mechanism has potential to become a new way to activate chloride transport within acidic environments in biological systems.
Results and discussion
Synthesis and characterisation
Oxosquaramides 1–4 were prepared using the zinc triflate mediated reaction of diethyl squarate with the appropriately substituted aniline, developed by Taylor and co-workers.26 Initial attempts to form the thiosquaramide derivatives 5–8 using Lawesson's reagent proved unsuccessful, while reaction with tetraphosphorus decasulfide (P4S10) gave thiosquaramide products in low yield as part of a complex mixture of products. However we found that the zwitterionic P4S10·pyridine complex, formed by reaction of P4S10 in refluxing pyridine,27 allowed the conversion of oxosquaramides 1–4 into thiosquaramides 5–8 in 66%, 48%, 37% and 60% yields respectively (Scheme 1). This strategy afforded each of the thiosquaramides in reasonable yields and 6–8 were easily purified by flash chromatography, while thiosquaramide 5 needed no further purification. Crystals suitable for single crystal X-ray analysis were obtained for thiosquaramides 5 and 8 by recrystallization from a concentrated DMSO solution allowing the elucidation of their solid state structure (see Fig. 1b for 8·DMSO). Tables of hydrogen bonds, data collection and refinement details can be found in the ESI.† Both 5 and 8 were found to form a 1
:
1 complex with DMSO in the solid state. In both cases the thiosquaramide moiety acts as a hydrogen bond donor to a single DMSO molecule with two hydrogen bonds formed between both available thiosquaramide NH groups and the oxygen atom of DMSO (N⋯O distances of 2.774–2.815 Å for 5·DMSO and 2.755–2.776 Å for 8·DMSO and N–H⋯O angles of 166–170° for 5·DMSO and 160–164° for 8·DMSO). These structures are similar to the crystal structures of the DMSO solvates of analogous squaramides 1 (previously published)22 and 4 (Fig. 1a) and also to those reported recently in a related study of luminescent squaramide based receptors.28 However, unlike the structures of oxosquaramides 1 and 4 the thiosquaramide structures are not fully planar, with the aromatic group being twisted out of the plane of the cyclobutene ring (see Fig. 1, torsion angles between the planes defined by the aromatic rings and the cyclobutene ring ranging between 2–6° and 15–19° for oxosquaramides 1·DMSO22 and 4·DMSO respectively and between 26–32° and 32–42° for analogous thiosquaramides 5·DMSO and 8·DMSO respectively). This twisted conformation likely results in a diminished contribution from the aromatic C–H hydrogen bonds that are often observed in complexes of oxosquaramides.22,29–31 The crystal structures reveal that steric hindrance between the large sulfur atoms and the ortho-CH hydrogen atoms is the cause of the non-planar geometry of the thiosquaramides and it is reasonable to assume that a fully planar anti/anti conformation can not be adopted in solution. In order to examine whether there is a difference in conformation between the oxosquaramides and thiosquaramides in solution, dynamic 1H NMR studies at various temperatures were performed on the most soluble compounds 4 and 8 (details can be found in the ESI†). It was found that in 1
:
1 CDCl3
:
DMSO-d6 oxosquaramide 4 is locked in the anti/anti conformation at temperatures between 273–333 K, whilst thiosquaramide 8 appears to be present in both syn/anti and anti/anti conformations with fast inter-conversion between each conformation. The difference in conformational equilibria is attributed to the larger steric size of the sulfur atoms in 8 which destabilize the anti/anti conformation.
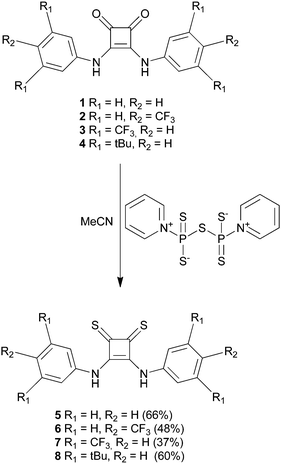 |
| Scheme 1 Synthesis of thiosquaramide receptors 5–8. Yields are given between brackets. | |
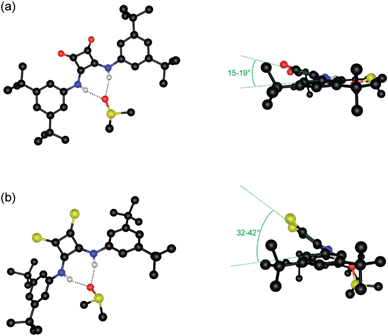 |
| Fig. 1 Front and side views of the X-ray crystal structure of 4·DMSO (a) and 8·DMSO (b). Ligands and DMSO molecules are shown in ball-and-stick. Non-interacting hydrogen atoms and non-interacting DMSO molecules are omitted for clarity. Hydrogen bonds are represented by dashed lines and atoms are colour coded as follows: C (black), H (white), F (green), N (blue), S (yellow) and O (red). Relevant torsion angles are given in green. | |
Anion binding
It has been observed that squaramides exhibit significantly higher anion affinities compared to their urea and thiourea analogues28–31 and that this results in superior anion transport abilities.22,32 A recent computational study concluded that thiosquaramides should have high acidity and hydrogen bond ability.33 Thus, we initially expected that thiosquaramides 5–8 would exhibit higher anion affinities than squaramide analogues 1–4. In order to evaluate the anion binding abilities of thiosquaramides 5–8, 1H NMR spectroscopic titrations at 298 K in 0.5% H2O in DMSO-d634 were performed by addition of tetrabutylammonium chloride (TBACl). Where possible, the data obtained from these titrations were plotted as the cumulative changes in chemical shift (Δδ) against the equivalents of anion added and the resulting plots were analysed using Hyperquad©.35 The best fit in all cases was to a 1
:
1 binding model (see ESI† for fitted data). The changes observed in the 1H NMR spectra of 5 and 8 upon the addition of TBACl showed that the NH signals associated with the thiosquaramide NHs were significantly shifted downfield. Unexpectedly however, the CF3 substituted receptors 6 and 7 did not exhibit such large changes and showed lower binding affinities for Cl− than both 5 and 8. Moreover, while 5 and 8 showed similar or higher affinities for Cl− than their squaramide counterparts 1 and 4, 6 and 7 showed considerably lower affinities for Cl− than 2 and 3. The association constants (Ka) of 1–8 in 0.5% H2O in DMSO-d6 with Cl− are summarised in Table 1 and demonstrate an unexpected trend whereby increasing the number of electron withdrawing substituents on the thiosquaramide unit results in lower affinities for Cl−. This is opposite to the trend seen for squaramides 1–4 and suggests that 6 and 7 may exist in deprotonated form in DMSO. Further support of this hypothesis is the recent report by Taylor et al. that a nitro-substituted squaramide exists in DMSO in a partially deprotonated form.26
Table 1 Summary of the chloride association constants Ka (M−1) of receptors 1–8 in 0.5% H2O in DMSO-d6 and CD3CN at 298 Ka
Receptor |
K
a (M−1) |
DMSO-d6 |
CD3CN |
Data fitted to a 1 : 1 model with errors <15%.
Value taken from ref. 22.
Receptor not sufficiently soluble to allow accurate Ka determination.
No measurable anion binding.
|
1
|
260b |
—c |
2
|
458b |
—c |
3
|
643b |
—c |
4
|
145 |
—c |
5
|
270 |
—c |
6
|
60 |
>104 |
7
|
—d |
114 |
8
|
402 |
—c |
To clarify this effect, 1H NMR titrations with 6 and 7 were also performed in CD3CN with TBACl. In this solvent the 1H NMR spectrum of 6 undergoes significant changes whereby the NH signals attributed to the thiosquaramide are shifted downfield upon the addition of TBACl (Fig. 2). The addition of Cl− initially resulted in peak broadening of all signals but when more than 1 equivalent of anion was added, well resolved signals were observed that were shifted significantly downfield from their original positions. This observation along with the considerable shifts exhibited by the aromatic protons strongly suggests the formation of a tightly bound host–guest complex in solution. Subsequent additions induced no further changes to the spectra. Interestingly, under the same conditions, the stability constant obtained for Cl− with 7 was found to be significantly lower than the value obtained with 6, indicating that this receptor is also partially deprotonated in CD3CN (Table 1). However, while considerations of NH acidity and deprotonation can explain the behaviour observed for thiosquaramides 6 and 7, they do not explain why the chloride affinities of 1 and 5 are almost identical in DMSO with 0.5% water, while the chloride affinity of thiosquaramide 8 is twice as high than analogous squaramide 4. Due to their higher NH acidity it is expected that thiosquaramides will display higher anion binding affinity than squaramides, but it appears that the higher conformational freedom of thiosquaramides (see above) can require a structure-dependent penalty to be paid upon anion binding. The magnitude of this penalty is difficult to predict but is expected to be different for simple thiosquaramide 5 and sterically bulky thiosquaramide 8.
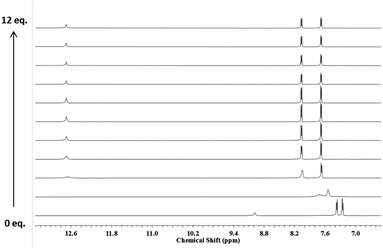 |
| Fig. 2 Stack plot of the 1H NMR spectrum of receptor 6 (2.5 × 10−3 M) upon titration with chloride (0–12 eq.) added as its tertabutylammonium salt in CD3CN at 25 °C. From bottom to top: 0, 0.5, 1.0, 1.5, 2, 3, 4, 6, 8, 10, 12 equiv. chloride. | |
Attempts were also made to investigate the anion binding behaviour of the thiosquaramides in the solid state. Previous X-ray crystal studies of oxosquaramides have shown that they form 1
:
1 complexes with tetrabutylammonium chloride in the solid state and that the squaramide adopts a fully planar anti/anti conformation in which the chloride anion is bound via hydrogen bonds from both NH hydrogen atoms and ortho-CH hydrogen atoms.22,26,30 As a comparison, single crystals of a chloride complex of thiosquaramide 6 were obtained by the slow evaporation of a 1
:
9 water–acetonitrile solution of 6 in the presence of excess tetramethylammonium (TMA) chloride (attempts to grow single crystals in the presence of TBACl failed). The X-ray crystal structure showed that thiosquaramide 6 can form a 2
:
1 receptor–chloride complex in the solid state, whereby a single chloride anion is coordinated by two thiosquaramide receptors via a total of four hydrogen bonds (N⋯Cl distances of 3.163–3.209 Å and N–H⋯Cl angles of 163–171°) (Fig. 3). Once again, a non-planar twisted conformation is observed that diminishes the potential contributions of the ortho-CH hydrogen atoms. Furthermore, the 2
:
1 stoichiometry observed here is different to the 1
:
1 stoichiometry seen in solution and in the crystal structures of analogous squaramides and it is not clear whether this is a packing effect resulting from the smaller TMA counter cation. Nevertheless, this crystal structure shows that a single chloride anion can accommodate multiple thiosquaramide receptors, which is relevant for the transmembrane anion transport ability of the thiosquaramides as the hydrophilic chloride anion needs to be screened from the apolar interior of the membrane in order to allow transport.
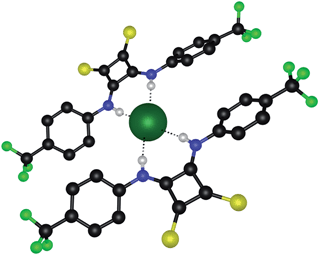 |
| Fig. 3 X-ray crystal structure of 6 with TMA chloride. Ligands are shown in ball-and-stick and bound chloride is shown in spacefill (0.6 times van der Waals radius). TMA counterions and non-interacting hydrogen atoms are omitted for clarity. Hydrogen bonds are represented by dashed lines and atoms are colour coded as follows: C (black), H (white), F (green), N (blue), S (yellow) and Cl (dark green). | |
pKa determination
In order to gain an insight into the acid–base behaviour of receptors 1–8 in aqueous solution pKa values were determined by theoretical and experimental means. Computational pKa values were obtained using density functional theory (DFT) at the M06-2X/6-311+G(3df,2p) level, in conjunction with the SMD continuum solvation model. Two water molecules were included in the model in order to take into account explicit interactions (details can be found in the ESI†). The results of the calculations are presented in Table 2. Experimental pKa values were obtained through pH-spectrophotometric titrations in a mixture of acetonitrile–water (9/1 v/v; in the presence of 0.1 M TBAPF6) as described by Fabbrizzi and co-workers.31 During the spectrophotometric measurements, each of the oxosquaramides was seen to undergo a single deprotonation event, characterised by a hypochromism at ∼370 nm and a concomitant hyperchromism at ∼340 nm. Similarly, the thiosquaramide deprotonation was signalled by a hypochromism at ∼405 nm and a concomitant hyperchromism at ∼335 nm and was accompanied by a change in the colour of the solution from bright yellow to dull orange. In Fig. 4, the titration profiles comparing squaramide 2 and the analogous thiosquaramide 6 are shown. A four parameter sigmoid curve was fitted through the data points using Sigma Plot (Systat Software Inc., Chicago, IL, USA) with the point of inflexion corresponding to the pKa. The obtained pKa values corresponding to a single deprotonation event are shown in Table 2 and range from 4.9–12.1. Encouragingly, pKa values for receptors 1 and 3 have also recently been determined in DMSO by Ni et al. using the Bordwell method and show values that are in close agreement with the values presented in this study (pKa(1) = 11.7 (found), 12.48 (literature); pKa(3) = 8.5 (found), 8.37(literature)).36 In general, there are reasonable agreements between the theoretically computed and the experimentally determined pKa values. As expected, both methods indicate that the thiosquaramides have lower pKa values than their analogous oxosquaramides and that the addition of electron withdrawing substituents results in a further lowering of the pKa values. The most acidic receptor was shown to be 7 (pKa = 4.9 (expt), 4.5 (DFT)). These values support the observation that electron deficient thiosquaramides exist in their deprotonated form at neutral pH and can explain some of the anion binding behaviour observed for thiosquaramides 6–7. Furthermore, pKa values below or around 7 indicate that thiosquaramides might be useful as pH-switchable anion transporters.
Table 2 Comparison of pKa values of receptors 1–8 (6 × 10−5 M) measured by spectrophotometric titrations in acetonitrile–water (9/1 v/v; in presence of 0.1 M TBAPF6) and calculated using DFT (M06-2X/6-311+G(3df,2p) with SMD continuum solvation)
Receptor |
pKa |
Experimental |
Computational |
Comparable to pKa obtained by Ni et al. in DMSO: pKa(1) = 12.48.
Comparable to pKa obtained by Ni et al. in DMSO: pKa(3) = 8.37.
|
1
|
11.7a |
11.3 |
2
|
9.8 |
9.1 |
3
|
8.5b |
7.9 |
4
|
12.1 |
12.0 |
5
|
7.3 |
9.0 |
6
|
5.3 |
6.6 |
7
|
4.9 |
4.5 |
8
|
7.7 |
9.6 |
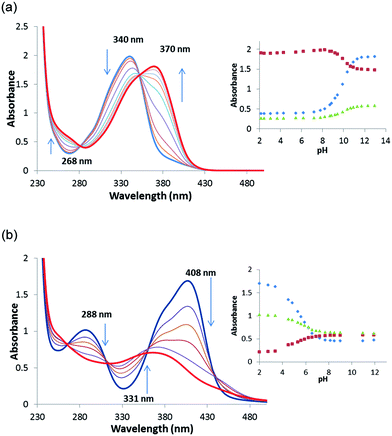 |
| Fig. 4 (a) Absorption spectra taken over the course of a pH-spectrophotometric titration of 2 (6 × 10−5 M) in an acetonitrile–water mixture (9/1 v/v; in presence of 0.1 M TBAPF6). Inset: Comparison plots of absorbance at 268 nm ( ), 340 nm ( ) and 370 nm ( ) vs. pH. (b) Absorption spectra taken over the course of a pH-spectrophotometric titration of 6 (5 × 10−5 M) in an acetonitrile–water mixture (9/1 v/v; in presence of 0.1 M TBAPF6). Inset: comparison plots of absorbance at 288 nm ( ), 331 nm ( ) and 408 nm ( ) vs. pH. | |
Anion transport
Previous studies have shown that squaramides are potent transmembrane anion transport agents that can outperform analogous ureas and thioureas.22,32 It has also been suggested that thioureas are better anion transporters than ureas due to their higher NH acidity and due to the higher lipophilicity of the sulfur atom.11,24 It was therefore envisaged that thiosquaramides have the potential to exceed any of the afore-mentioned functional groups in their intrinsic anion transport ability. To test this hypothesis vesicle-based experiments were performed according to standard literature procedures.37,38 Initially, unilamellar 1-palmitoyl-2-oleoyl-sn-glycero-3-phosphocholine (POPC) liposomes loaded with a NaCl solution (489 mM) buffered to pH 7.2 were prepared and suspended in an isotonic NaNO3 solution (489 mM) buffered to pH 7.2. A small volume of a DMSO solution34 of 1–8 was added to these liposomes and the resulting chloride efflux was monitored using an ion selective electrode (ISE). The results for thiosquaramides 5–8 are given in Fig. 5 (empty symbols) and show that the thiosquaramides possess only limited anion transport ability at this pH (the results for oxosquaramides 1–3 have been previously reported).22 Spectrophotometric pKa determinations, however, indicate that the pKa values of the thiosquaramides are in the range 4.0–8.0 (Table 2). This implies that at pH 7.2 a large proportion of the thiosquaramides will be present in their deprotonated, negatively charged form and will be unable to transport anions across lipid bilayers due to charge repulsion. The ion selective electrode experiments were therefore repeated with unilamellar POPC vesicles loaded with NaCl solution (489 mM) buffered to pH 4.0 and suspended in NaNO3 solution (489 mM) buffered to pH 4.0. At this pH the majority of the compounds should be present in their neutral form and anion transport should be ‘switched on’. The results shown in Fig. 5 (filled symbols) reveal that the anion transport ability of thiosquaramides 5 and 6 is indeed significantly enhanced at lower pH (the results for the oxo-squaramides can be found in the ESI†).
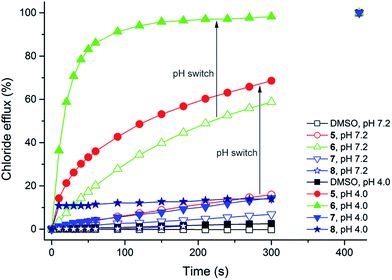 |
| Fig. 5 Chloride efflux from POPC vesicles at pH 7.2 (empty symbols) and pH 4.0 (filled symbols) mediated by thiosquaramides 5–8 (1 mol% with respect to lipid). POPC vesicles were loaded with a 489 mM NaCl solution buffered to pH 7.2 with 5 mM phosphate salts or to pH 4.0 with 5 mM citrate salts, and were suspended in a 489 mM NaNO3 solution buffered to pH 7.2 with 5 mM phosphate salts or to pH 4.0 with 5 mM citrate salts. At the end of the experiment (300 s), detergent was added to lyse the vesicles and calibrate the ISE to 100% chloride efflux. Each point represents the average of a minimum of 6 independent trials. DMSO was used as a control. | |
In order to quantify this effect, Hill analyses39 of the anion transport facilitated by 1–8 were performed at both pH 7.2 and pH 4.0, whereby the vesicle-based experiments were repeated at various concentrations of receptor to obtain EC50 values, i.e. the concentration of transporter (in mol% with respect to lipid) required to obtain 50% chloride efflux in 270 s (Table 3). It is clear from Table 3 that the oxosquaramides are powerful anion transporters at both pH 7.2 and pH 4.0, with little difference in activity between the two pHs. In contrast, all of the thiosquaramides are unable to effectively transport chloride out of POPC vesicles buffered to pH 7.2 at low carrier concentrations, but thiosquaramides 5 and 6 are significantly better transporters than their analogous oxosquaramides at pH 4.0. These Hill analyses further confirm the hypothesis that the transmembrane anion transport activity of the thiosquaramides can be switched on by lowering the pH. The fact that thiosquaramides 5 and 6 display higher anion transport activity at pH 4.0 than the analogous oxosquaramides 1 and 2 is presumably due to their higher NH acidity and higher lipophilicity. It must be noted, however, that thiosquaramides 7 and 8 (and oxosquaramide 4) do not show any significant anion transport at either pH 7.2 or pH 4.0. There could be several explanations for this behaviour. The experimental pKa value of receptor 7 (Table 2), suggests that even at pH 4.0 a significant amount of the receptor will be present in its inactive, deprotonated form (pure POPC vesicles were found to be unstable at pH < 4.0 and it was thus not possible to test whether this receptor could also be ‘switched on’ at even lower pH). While pKa considerations can explain the inactivity of compound 7, they cannot explain the behaviour seen for tert-butyl substituted receptors 4 and 8, which have much higher pKa values (Table 2). On the other hand, 4 and 8 are the most bulky receptors and this might cause a problem when aggregates or higher stoichiometries are needed for chloride transport (such as a 2
:
1 stoichiometry seen in the solid state, Fig. 3). This was corroborated by the high Hill coefficients (n-values) that were observed during the Hill analyses of 5 and 6 at pH 4.0 (see ESI†), indicating that higher order aggregates are indeed required for anion transport by these simple thiosquaramides.24,40 Another reason for the low anion transport activity of 4, 7 and 8 could be their lipophilicity. It has often been suggested that ion transport across lipid bilayers is diminished when receptors become too lipophilic to interact with the ions at the lipid-water interphase.12,24,41,42 In order to obtain an estimate of the lipophilicity of compounds 1–8, experimental retention times on reversed-phase HPLC43 were determined (Table 3). It is clear that the three inactive transporters are also the receptors with the highest lipophilicity (highest retention time), suggesting that lipophilicity may also contribute to the inactivity of 4, 7 and 8.
Table 3 Summary of the anion transport behaviour of receptors 1–8
|
EC50 at pH 7.2a (mol%) |
EC50 at pH 4.0a (mol%) |
Retention timeb (s) |
Apparent pKac |
Concentration of transporter (mol% with respect to lipid) needed to achieve 50% chloride efflux in 270 s from POPC vesicles filled with NaCl and buffered to pH 7.2 or pH 4.0.
Retention time of the compounds on a reversed-phase HPLC column.
pKa value obtained by performing anion transport studies at various pH (see main text for details).
Value taken from ref. 22.
Receptors are not active enough to allow EC50 or pKa determination.
Hill analysis revealed high n-values (n > 5), rendering the EC50 values inaccurate, however, loadings of 0.0125 mol% and higher consistently gave chloride effluxes greater than 50% in 270 s and EC50 values can thus be estimated as 0.0125 mol% or lower (lower loadings of transporters gave inconsistent results – see ESI†).
|
1
|
1.38d |
1.42 |
20.5 |
10.9 (±0.5) |
2
|
0.06d |
0.08 |
26.0 |
11.2 (±0.2) |
3
|
0.01d |
0.01 |
29.2 |
9.8 (±0.3) |
4
|
—e |
—e |
33.8 |
—e |
5
|
—e |
0.0125f |
22.7 |
6.3 (±0.1) |
6
|
0.68 |
0.0125f |
26.6 |
6.0 (±0.2) |
7
|
—e |
—e |
31.2 |
—e |
8
|
—e |
—e |
35.0 |
—e |
In principle, the chloride transport behaviour of the thiosquaramides could be due to either the formation of membrane-spanning channels or a mobile carrier mechanism. Oxosquaramides 1–3 have previously been shown to function primarily via a mobile carrier mechanism22 and it is likely that the same holds true for the thiosquaramides. Although the high Hill coefficients observed for 5 and 6 could indicate channel formation (see ESI†), they are more likely the result of aggregation (or precipitation) of the receptors, or multiple receptors complexing one chloride anion for optimal screening of the highly hydrophilic anion from the lipid environment.24,40 Evidence for a mobile carrier mechanism by thiosquaramides 58 was obtained by repeating the transport experiments with vesicles consisting of 3
:
7 cholesterol
:
POPC. It has often been reported that cholesterol increases the viscosity of a lipid bilayer and thereby exerts a negative effect on processes that depend on diffusion through the bilayer (such as a mobile carrier mechanism).44–47 A decrease in chloride transport mediated by 1–8 was observed at both pH 7.2 and pH 4.0 for cholesterol-containing liposomes, evidence in support of a mobile carrier mechanism (see ESI†). Furthermore, calcein leakage assays48–50 revealed that the large highly anionic dye calcein did not leak from POPC vesicles at pH 7.2 or pH 4.0 in the presence of 1–8 (see ESI†), excluding the formation of pores or channels with large internal diameter and vesicle disruption induced by the transporters. The most plausible anion transport mechanism displayed by thiosquaramides 1–8 is therefore a mobile carrier process.
The charge imbalance created by the transmembrane anion transport promoted by synthetic molecules can be compensated via either an antiport process, where two anions are transported across the bilayer in opposite directions, or a symport process, where both an anion and a cation are transported in the same direction. The chloride efflux shown in Fig. 5 is therefore most likely the result of either a pure ‘switch-on’ Cl−/NO3− antiport mechanism or the occurrence of a H+/Cl− symport process at lower pH (or a combination of both). To investigate which mechanism is dominant for the thiosquaramide based receptors, POPC liposomes containing a NaCl solution and suspended in a Na2SO4 solution were prepared at pH 7.2, pH 4.0 and in the presence of a pH gradient (pH 4.0 inside and pH 7.2 outside the vesicle). The double negatively charged sulfate anion is considerably more hydrophilic than nitrate (ΔGhydr(SO42−) = −1080 kJ mol−1; ΔGhydr(NO3−) = −300 kJ mol−1)51 and is therefore more challenging to transport across a lipid bilayer. It was found that in these circumstances only a minimal amount of chloride was transported out of the liposomes at both pH 7.2 and pH 4.0 (see ESI†). Furthermore, even in the presence of a pH gradient no significant chloride efflux occurred upon the addition of transporters 1–8 (see ESI†). While these results strongly suggest that an antiport mechanism is the dominant process for the chloride transport facilitated by 1–8, it must be noted that a pH gradient of pH 4.0 to pH 7.2 corresponds to a gradient in [H+] of ∼10−4 M. This is a very small fraction of the nearly 500 mM [Cl−] gradient that is applied to the system and HCl symport mechanisms are therefore extremely hard to detect using a chloride selective electrode.
In order to study the possibility of HCl symport in more detail, a series of vesicle-based experiments were conducted using the pH-dependent fluorescence properties of 8-hydroxy-1,3,6-pyrenetrisulfonate (HPTS) (Fig. 6).49,52,53 Initially, POPC liposomes were prepared containing NaCl (489 mM) and HPTS (1 mM) buffered to pH 7.2 or pH 6.0, and suspended in a NaNO3 (489 mM) solution buffered to pH 7.2 or pH 6.0. The results shown in Fig. 6 for 2 and 6 reveal that in the absence of a pH gradient the intravesicular pH does not change upon the addition of thiosquaramide based transporters (results for the other receptors can be found in the ESI†), indicating that the chloride effluxes shown in Fig. 5 are mainly the result of Cl−/NO3− antiport processes that occur at all pHs. However, when a pH gradient is applied across the phospholipid bilayer, a fast dissipation of the gradient is observed upon the addition of both oxosquaramides and thiosquaramides 1–8 (Fig. 6), suggesting that H+/Cl− symport (or OH−/Cl− antiport) can occur under these conditions. Similar HPTS fluorescence based studies were also conducted on vesicles loaded with NaCl and suspended in Na2SO4 (see ESI†). As with the previous experiments with external nitrate, only a small increase in internal pH was observed when both the intravesicular and extravesicular solution were buffered to pH 7.2, while a large increase in internal pH was seen when a pH gradient was applied. However, unlike the nitrate tests, a large increase in internal pH was also observed when both intra- and extravesicular solution were buffered to pH 6.0, hinting that H+/Cl− symport (or OH−/Cl− antiport) can occur without a pH gradient providing that sulfate is the external anion. In conclusion, it appears that the chloride transport ability of the thiosquaramides is mainly the result of a Cl−/NO3− antiport mechanism that can be switched on by lowering the pH, while H+/Cl− symport will occur only when a favourable [H+] gradient is applied or in the absence of an external anion suitable for antiport.
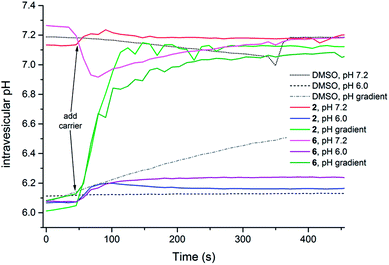 |
| Fig. 6 Investigation of HCl transport facilitated by 1–8 using the fluorescent dye HPTS to estimate the intravesicular pH. For the experiments without pH gradient, POPC vesicles were loaded with 489 mM NaCl, 1 mM HPTS, buffered to pH 7.2 with phosphate buffer or to pH 6.0 with citrate buffer, and suspended in a solution of 489 mM NaNO3 buffered to pH 7.2 with phosphate buffer or to pH 6.0 with citrate buffer. For the experiments with pH gradient, POPC vesicles were loaded with 489 mM NaCl, 1 mM HPTS, buffered to pH 6.0 with citrate buffer, and suspended in a solution of 489 mM NaNO3 buffered to pH 6.0 with citrate buffer, and at time t = 0 s a NaOH solution was added to achieve an external pH of 7.0. At time t = 50 s a DMSO solution of the transporter was added (1 mol% with respect to lipid) and at time t = 350 s detergent was added. Each line represents the average of 3 independent trials and DMSO was used as a control. | |
The pH-switchable transport behaviour displayed by the thiosquaramides renders this class of compound interesting for potential biological activity, as the careful control of ion transport processes is of utmost importance in a biological setting. For example, many of the naturally occurring chloride transporting proteins exhibit a gating or switching mechanism where the ion transport is activated by a specific biological trigger (e.g. pH, Ca2+, voltage or ligand gating).54–56 Further-more, it has often been suggested that HCl symport can result in anti-cancer activity7–10 and as the internal and external pH of cancer cells is thought to be different to that of healthy cells,57 pH switchable chloride transporters could become promising leads for anti-cancer drugs. In order to be biologically relevant, this pH-switch should occur around the physiological pH of 7.4 (in the range pH 6–8). To probe whether the pH-switch of the thiosquaramides falls into this pH range, the Cl−/NO3− experiments depicted in Fig. 5 were repeated at a range of pHs (see ESI† for details on the different pHs and buffers used). The results for 2 and 6 are shown in Fig. 7 and clearly show that the chloride transport ability of thiosquaramide 6 can be switched on at pH < 7, with the major switch occurring in the region pH 5.5–7.0. Fig. 7 also confirms that chloride transport by the analogous oxosquaramide 2 is relatively pH-independent and that thiosquaramide 6 displays more potent transmembrane anion transport activity than oxosquaramide 2 at pH < 6.0. In addition, the exact position of the switch should correspond to the pKa value of the receptor in the conditions of the anion transport experiments (but should be more accurately referred to as an ‘apparent pKa’ as it is a combination of the NH pKa and the transport ability of the receptor). In order to calculate the apparent pKa values it is theoretically possible to plot the chloride efflux (%) after a certain amount of time versus pH and fit the data to a sigmoidal function. However, this single-point approach can artificially raise the value of the calculated pKa, especially in the case of highly active anion transporters (see ESI†). A more accurate approach is therefore to use the normalised initial rate of chloride efflux to calculate apparent pKa values. The values obtained using this method are summarised in Table 3 and largely agree with the pKa values obtained through spectrophotometric titrations and DFT calculations (Table 2). The apparent pKa values of the oxosquaramides are in the region pH 10–11, while the apparent pKa thiosquaramides 5 and 6 is in the region pH 6–7. The major discrepancy between the spectrophotometric pKa values and the apparent pKa values is that a less pronounced effect of the addition of electron withdrawing substituents is observed for the apparent (transport) pKa compared to the spectrophoto-metric pKa. It is possible that, in the case of apparent pKa values, higher anion transport activity compensates for the stronger NH acidity of receptors containing electron with-drawing substituents.
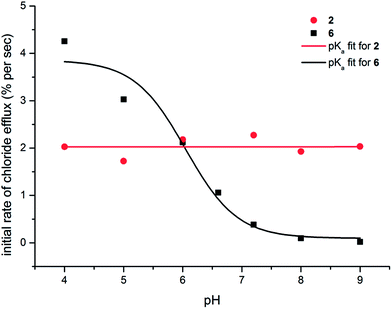 |
| Fig. 7 pH dependence of the chloride transport ability of compounds 2 and 6 (1 mol% with respect to lipid). Chloride transport experiments were conducted as described in Fig. 5 but at various different pHs. The obtained data was fitted to an asymptotic function and the initial rate of chloride efflux was calculated (see ESI† for details) and plotted as a function of pH. Apparent pKa values could subsequently be estimated by fitting the obtained plot to the following sigmoidal function: y = max + ((min − max)/(1 + 10(pKa−x))). | |
In summary, it was found that thiosquaramides 5 and 6 are able to transport chloride across phospholipid bilayers in a pH-dependent manner via an acidic ‘switch-on’ antiport mechanism. At high pH (pH > 7), the thiosquaramides are present as deprotonated, negatively charged species that are unable to complex and transport anions, while at lower pH (pH < 7) they are present as neutral species capable of anion binding. This is a significantly different mechanism than the behaviour of other pH-dependent anion transporters or HCl symporters, such as the prodigiosins.58–64 In these cases the anion transport of a neutral compound is enhanced by protonation of a basic functional group to form a positively charged species that can coordinate and subsequently transport anions. However, the neutral species is usually still an active transporter and so the anion transport cannot be fully ‘switched off’ using this approach. The thiosquaramides on the other hand can alternate between an inactive negatively charged species and an active neutral species and consequently provide a true ON–OFF switch for anion transport. So far, there have only been two other reports concerning switching-off anion transport by deprotonation, but these involve deprotonation of phenolic OH groups which have high pKa values and the switch consequently occurs at pHs that are not physiologically relevant (pH > 7.4).65,66 On the other hand, the ON–OFF switch in the region pH 6–7 gives the thiosquaramide based receptors more potential in the biological arena and an investigation of the biological activity of thiosquaramides is currently ongoing in our laboratories.
Conclusions
In this paper we have reported the first use of thiosquaramides as anion receptors and pH-switchable anion transporters. Proton NMR titrations in DMSO-d6 and CD3CN, as well as single crystal X-ray crystallography, revealed that thiosquaramides are able to form 1
:
1 and 2
:
1 complexes with chloride anions. It was also found that thiosquaramides containing electron with-drawing substituents are present in their deprotonated forms in DMSO and water. Vesicle studies using ion-selective electrodes and pH sensitive fluorescent dyes indicated that the trans-membrane anion transport abilities of the thiosquaramides is switched OFF at pH > 7 and switched ON at pH < 7. It was also shown that in the ON mode, the thiosquaramides function as mobile carriers that can promote chloride efflux mainly via a chloride/nitrate antiport process, although HCl symport also occurs in the presence of a pH gradient. This paper provides one of the few examples of truly controllable and switchable anion transport by synthetic molecules and this finding renders thiosquaramide based receptors interesting targets for developing future biologically active anion transporters.
Acknowledgements
We thank the EPSRC (EP/J009687/1) and the ARC (DP140100227) for funding and the EPSRC for access to the crystallographic facilities at the University of Southampton. XW thanks the China Scholarship Council and the University of Southampton for financial support. ILK thanks the University of Southampton for a teaching assistantship. BC acknowledges the provision of computing time from the NCI. BDS thanks the NSF (USA). PAG thanks the Royal Society and the Wolfson Foundation for a Royal Society Wolfson Research Merit Award. PAG and KAJ thank the European Cooperation in Science and Technology (COST) action CM1005 “Supramolecular Chemistry in Water” for support.
Notes and references
- J. T. Davis, O. Okunola and R. Quesada, Chem. Soc. Rev., 2010, 39, 3843–3862 RSC.
- P. R. Brotherhood and A. P. Davis, Chem. Soc. Rev., 2010, 39, 3633–3647 RSC.
- A. P. Davis, D. N. Sheppard and B. D. Smith, Chem. Soc. Rev., 2007, 36, 348–357 RSC.
- N. Busschaert and P. A. Gale, Angew. Chem., Int. Ed., 2013, 52, 1374–1382 CrossRef CAS PubMed.
- P. A. Gale, R. Pérez-Tomás and R. Quesada, Acc. Chem. Res., 2013, 46, 2801–2813 CrossRef CAS PubMed.
-
F. M. Ashcroft, Ion Channels and Disease, Academic Press, San Diego and London, 2000 Search PubMed.
- S. Matsuyama, J. Llopis, Q. L. Deveraux, R. Y. Tsien and J. C. Reed, Nat. Cell Biol., 2000, 2, 318–325 CrossRef CAS PubMed.
- J. L. Sessler, L. R. Eller, W.-S. Cho, S. Nicolaou, A. Aguilar, J. T. Lee, V. M. Lynch and D. J. Magda, Angew. Chem., Int. Ed., 2005, 44, 5989–5992 CrossRef CAS PubMed.
- T. Sato, H. Konno, Y. Tanaka, T. Kataoka, K. Nagai, H. H. Wasserman and S. Ohkuma, J. Biol. Chem., 1998, 273, 21455–21462 CrossRef CAS PubMed.
- S. Ohkuma, T. Sato, M. Okamoto, H. Matsuya, K. Arai, T. Kataoka, K. Nagai and H. H. Wasserman, Biochem. J., 1998, 334, 731–741 CAS.
- N. J. Andrews, C. J. E. Haynes, M. E. Light, S. J. Moore, C. C. Tong, J. T. Davis, W. A. Harrell Jr and P. A. Gale, Chem. Sci., 2011, 2, 256–260 RSC.
- N. Busschaert, S. J. Bradberry, M. Wenzel, C. J. E. Haynes, J. R. Hiscock, I. L. Kirby, L. E. Karagiannidis, S. J. Moore, N. J. Wells, J. Herniman, G. J. Langley, P. N. Horton, M. E. Light, I. Marques, P. J. Costa, V. Felix, J. G. Frey and P. A. Gale, Chem. Sci., 2013, 4, 3036–3045 RSC.
- R. Prohens, S. Tomàs, J. Morey, P. M. Deyà, P. Ballester and A. Costa, Tetrahedron Lett., 1998, 39, 1063–1066 CrossRef CAS.
- D. Quiñonero, R. Prohens, C. Garau, A. Frontera, P. Ballester, A. Costa and P. M. Deyà, Chem. Phys. Lett., 2002, 351, 115–120 CrossRef.
- C. Garau, A. Frontera, P. Ballester, D. Quiñonero, A. Costa and P. M. Deyà, Eur. J. Org. Chem., 2005, 179–183 CrossRef CAS.
- A. Frontera, J. Morey, A. Oliver, M. N. Piña, D. Quiñonero, A. Costa, P. Ballester, P. M. Deyà and E. V. Anslyn, J. Org. Chem., 2006, 71, 7185–7195 CrossRef CAS PubMed.
- V. Ramalingam, M. E. Domaradzki, S. Jang and R. S. Muthyala, Org. Lett., 2008, 10, 3315–3318 CrossRef CAS PubMed.
- C. Jin, M. Zhang, L. Wu, Y. Guan, Y. Pan, J. Jiang, C. Lin and L. Wang, Chem. Commun., 2013, 49, 2025–2027 RSC.
- D. Quiñonero, A. Frontera, G. A. Suñer, J. Morey, A. Costa, P. Ballester and P. M. Deyà, Chem. Phys. Lett., 2000, 326, 247–254 CrossRef.
- R. Ian Storer, C. Aciro and L. H. Jones, Chem. Soc. Rev., 2011, 40, 2330–2346 RSC.
- F. Olmo, C. Rotger, I. Ramírez-Macías, L. Martínez, C. Marín, L. Carreras, K. Urbanová, M. Vega, G. Chaves-Lemaur, A. Sampedro, M. J. Rosales, M. Sánchez-Moreno and A. Costa, J. Med. Chem., 2014, 57, 987–999 CrossRef CAS PubMed.
- N. Busschaert, I. L. Kirby, S. Young, S. J. Coles, P. N. Horton, M. E. Light and P. A. Gale, Angew. Chem., Int. Ed., 2012, 51, 4426–4430 CrossRef CAS PubMed.
- N. Busschaert, P. A. Gale, C. J. E. Haynes, M. E. Light, S. J. Moore, C. C. Tong, J. T. Davis and J. W. A. Harrell, Chem. Commun., 2010, 46, 6252–6254 RSC.
- N. Busschaert, M. Wenzel, M. E. Light, P. Iglesias-Hernández, R. Pérez-Tomás and P. A. Gale, J. Am. Chem. Soc., 2011, 133, 14136–14148 CrossRef CAS PubMed.
- S. J. Moore, M. Wenzel, M. E. Light, R. Morley, S. J. Bradberry, P. Gomez-Iglesias, V. Soto-Cerrato, R. Perez-Tomas and P. A. Gale, Chem. Sci., 2012, 3, 2501–2509 RSC.
- A. Rostami, A. Colin, X. Y. Li, M. G. Chudzinski, A. J. Lough and M. S. Taylor, J. Org. Chem., 2010, 75, 3983–3992 CrossRef CAS PubMed.
- J. Bergman, B. Pettersson, V. Hasimbegovic and P. H. Svensson, J. Org. Chem., 2011, 76, 1546–1553 CrossRef CAS PubMed.
- R. B. P. Elmes, P. Turner and K. A. Jolliffe, Org. Lett., 2013, 15, 5638–5641 CrossRef CAS PubMed.
- R. B. P. Elmes, K. Yuen and K. A. Jolliffe, Chem.–Eur. J., 2014, 20, 7373–7380 CrossRef CAS PubMed.
- V. Amendola, G. Bergamaschi, M. Boiocchi, L. Fabbrizzi and M. Milani, Chem.–Eur. J., 2010, 16, 4368–4380 CrossRef CAS PubMed.
- V. Amendola, L. Fabbrizzi, L. Mosca and F.-P. Schmidtchen, Chem.–Eur. J., 2011, 17, 5972–5981 CrossRef CAS PubMed.
- L.-Q. Deng, Y.-M. Lu, C.-Q. Zhou, J.-X. Chen, B. Wang and W.-H. Chen, Bioorg. Med. Chem. Lett., 2014, 24, 2859–2862 CrossRef CAS PubMed.
- T. Lu and S. E. Wheeler, Chem.–Eur. J., 2013, 19, 15141–15147 CrossRef CAS PubMed.
- Thiosquaramides with electron withdrawing substituents (especially 7) were found to be unstable in DMSO and gradually degraded over time. Experiments conducted in DMSO were therefore always performed with a fresh solution that was used within 30 minutes to avoid errors. The thiosquaramides were found to be more stable in other solvents and where possible the results obtained in DMSO were verified by repeating them with acetonitrile solutions (however, not all of the receptors were soluble in acetonitrile and so this was not always possible). See ESI† for additional details about thiosquaramide stability.
- P. Gans, A. Sabatini and A. Vacca, Talanta, 1996, 43, 1739–1753 CrossRef CAS.
- X. Ni, X. Li, Z. Wang and J.-P. Cheng, Org. Lett., 2014, 16, 1786–1789 CrossRef CAS PubMed.
- B. D. Smith and T. N. Lambert, Chem. Commun., 2003, 2261–2268 RSC.
- A. V. Koulov, T. N. Lambert, R. Shukla, M. Jain, J. M. Boon, B. D. Smith, H. Li, D. N. Sheppard, J.-B. Joos, J. P. Clare and A. P. Davis, Angew. Chem., Int. Ed., 2003, 42, 4931–4933 CrossRef CAS PubMed.
- A. V. Hill, Biochem. J., 1913, 7, 471–480 CAS.
- S. Bhosale and S. Matile, Chirality, 2006, 18, 849–856 CrossRef CAS PubMed.
- V. Saggiomo, S. Otto, I. Marques, V. Felix, T. Torroba and R. Quesada, Chem. Commun., 2012, 48, 5274–5276 RSC.
- H. Valkenier, C. J. E. Haynes, J. Herniman, P. A. Gale and A. P. Davis, Chem. Sci., 2014, 5, 1128–1134 RSC.
- T. Braumann, J. Chromatogr., 1986, 373, 191–225 CrossRef CAS.
- J. Hjort Ipsen, G. Karlström, O. G. Mourtisen, H. Wennerström and M. J. Zuckermann, Biochim. Biophys. Acta, Biomembr., 1987, 905, 162–172 CrossRef.
- T. P. W. McMullen, R. N. A. H. Lewis and R. N. McElhaney, Curr. Opin. Colloid Interface Sci., 2004, 8, 459–468 CrossRef CAS PubMed.
- J. C. M. Holthuis, G. van Meer and K. Huitema, Mol. Membr. Biol., 2003, 20, 231–241 CrossRef CAS.
- W. F. D. Bennett, J. L. MacCallum and D. P. Tieleman, J. Am. Chem. Soc., 2009, 131, 1972–1978 CrossRef CAS PubMed.
-
V. P. Torchilin and V. Weissig, Liposomes: a practical approach, Oxford University Press, 2003 Search PubMed.
- V. Sidorov, F. W. Kotch, G. Abdrakhmanova, R. Mizani, J. C. Fettinger and J. T. Davis, J. Am. Chem. Soc., 2002, 124, 2267–2278 CrossRef CAS PubMed.
- T. N. Lambert, J. M. Boon, B. D. Smith, M. N. Pérez-Payán and A. P. Davis, J. Am. Chem. Soc., 2002, 124, 5276–5277 CrossRef CAS PubMed.
- Y. Marcus, J. Chem. Soc., Faraday Trans., 1991, 87, 2995–2999 RSC.
- N. R. Clement and J. M. Gould, Biochemistry, 1981, 20, 1534–1538 CrossRef CAS.
- K. Kano and J. H. Fendler, Biochim. Biophys. Acta, 1978, 509, 289–299 CrossRef CAS.
- T. J. Jentsch, V. Stein, F. Weinreich and A. A. Zdebik, Physiol. Rev., 2002, 82, 503–568 CAS.
- C. Duran, C. H. Thompson, Q. Xiao and H. C. Hartzell, Annu. Rev. Physiol., 2010, 72, 95–121 CrossRef CAS PubMed.
- L. Feng, E. B. Campbell, Y. Hsiung and R. MacKinnon, Science, 2010, 330, 635–641 CrossRef CAS PubMed.
- J. R. Griffiths, Br. J. Cancer, 1991, 64, 425–427 CrossRef CAS.
-
J. T. Davis, in Topics in Heterocyclic Chemistry, ed. P. A. Gale and W. Dehaen, Springer, New York, 2010, vol. 24, pp. 145–176 Search PubMed.
- J. L. Seganish and J. T. Davis, Chem. Commun., 2005, 5781–5783 RSC.
- J. T. Davis, P. A. Gale, O. A. Okunola, P. Prados, J. C. Iglesias-Sánchez, T. Torroba and R. Quesada, Nat. Chem., 2009, 1, 138–144 CrossRef CAS PubMed.
- P. A. Gale, M. E. Light, B. McNally, K. Navakhun, K. E. Sliwinski and B. D. Smith, Chem. Commun., 2005, 3773–3775 RSC.
- P. I. Hernandez, D. Moreno, A. A. Javier, T. Torroba, R. Perez-Tomas and R. Quesada, Chem. Commun., 2012, 48, 1556–1558 RSC.
- P. A. Gale, J. Garric, M. E. Light, B. A. McNally and B. D. Smith, Chem. Commun., 2007, 1736–1738 RSC.
- S. D. Whitmarsh, A. P. Redmond, V. Sgarlata and A. P. Davis, Chem. Commun., 2008, 3669–3671 RSC.
- O. A. Okunola, J. L. Seganish, K. J. Salimian, P. Y. Zavalij and J. T. Davis, Tetrahedron, 2007, 63, 10743–10750 CrossRef CAS PubMed.
- P. V. Santacroce, J. T. Davis, M. E. Light, P. A. Gale, J. C. Iglesias-Sánchez, P. Prados and R. Quesada, J. Am. Chem. Soc., 2007, 129, 1886–1887 CrossRef CAS PubMed.
Footnotes |
† Electronic supplementary information (ESI) available: Synthesis and characterisation of the receptors, details and figures about the X-ray crystal structures of the receptors and anion complexes, conformational studies, thiosquaramide stability studies, association constant determination, stack plots and fitplots of the 1H NMR titrations, pH-spectrophotometric titrations, DFT calculations of pKa values, various vesicle assays methods and Hill plots. CCDC 1005458–1005461. For ESI and crystallographic data in CIF or other electronic format see DOI: 10.1039/c4sc01629g |
‡ These authors contributed equally to this work. |
|
This journal is © The Royal Society of Chemistry 2014 |