DOI:
10.1039/C4RA14895A
(Paper)
RSC Adv., 2015,
5, 5598-5603
An autonomic and “off–on–off”-switchable polymer microreactor
Received
30th October 2014
, Accepted 2nd December 2014
First published on 2nd December 2014
Abstract
An originally designed autonomic and “off–on–off”-switchable polymer microreactor is reported. This polymer microreactor was made of nickel nanoparticles and a unique shape-memory polymer consisting of poly(acrylamide) and pendent dodecyl side chains that exhibited switchable domains. This reactor showed weak reactivity at relatively low temperatures due to the low mobility of molecular chains in the switchable domains, which inhibited the access of substrate to the encapsulated nickel nanoparticles (i.e., catalytic ‘off’ status). This reactor also showed weak reactivity at relatively high temperatures in response to the significantly increased hydrophobicity (viz., catalytic ‘off’ status). This reactor only demonstrated significant catalysis at modest temperatures, arising from the relative balance between the mobility of molecular chains and the hydrophobicity in the switchable domains (i.e., catalytic ‘on’ status). In this way, this polymer microreactor demonstrated the autonomic “off–on–off” catalytic ability. This novel design opens up the opportunity to develop smart polymer microreactors for controlled catalytic processes.
1. Introduction
There are tremendous implications in metal nanoparticles due to their importance in a broad range of applications, and particularly in catalytic applications. The recent advances in this field and synthetic polymers offer new opportunities to develop functional catalysts by incorporating both components into individual entitles.1,2 Prominent among these are the so-called polymer microreactors, which have gained traction due to the tantalizing prospect of controlling catalytic processes in chemical synthesis and in inaccessible environments. From the earliest endeavors, exemplified by poly(N-isopropylacrylamide) (PNIPAm)-encapsulated Ag nanoparticles,3,4 polymer microreactors have been shown to demonstrate tunable catalysis in water. This results from the temperature-dependent hydrophilic/hydrophobic transition of PNIPAm, which causes either impeded or unobstructed access into the encapsulated Ag nanoparticles. In this way, catalysis by the polymer microreactors demonstrates a tunable process in conjunction with increasing temperature. Despite the innovative properties, these polymer microreactors have not realized their full potentials, mainly because most of the controlled catalytic processes would require the microreactors that can work at a specified temperature in order to cater to an optimized reaction conditions.5 Unfortunately, it is not realistic to directly acquire such polymer microreactors based on currently available technology and methods. As such, new technology and methods are needed.
For centuries, mankind has been learning and acquiring knowledge from nature. In fact, a body of knowledge is already available. Shape-memory polymers (SMPs)6 appear to share a promising prospect with the dilemma of polymer microreactors. Although the SMPs and their mechanisms are diverse, the common ground lies in the conceptual combination of static netpoints and switchable domains, such as crosslinked poly(acrylic acid-co-stearyl acrylate),7 where the crosslinked netpoints stabilize the permanent shape and the switchable domains fix the temporary shape. The change in the mobility of aliphatic side chains in the switchable domains is the major driving force that transforms a temporary shape to a permanent shape (depending on the temperature whether it is below or above the transition temperature). Below the transition temperature, the “frozen” molecular chains in the switchable domains cause the polymer to behave like a hard polymer. Above the transition temperature, the increased mobility of the aliphatic side chains contributes to the flexibility of this polymer. The temporary shape may be maintained by applying a deforming force during the cooling process. Heating this polymer again above the transition temperature results in the recovery of the permanent shape. In this way, the molecular mobility of aliphatic side chains in the switchable domains acts as a molecular switch for the occurrence of the shape-memory effect. Although these works are not related to catalytic applications, the methods developed by scientists for SMPs provide a new insight into the dilemma of polymer microreactors, which makes the switchable domains feasible.
Inspired by this principle, herein, we reported an autonomic and “off–on–off”-switchable polymer microreactor (i.e., NiPR-S). This polymer microreactor was constructed from nickel nanoparticles and a unique shape-memory polymer consisting of crosslinked poly(acrylamide) (PAAm) and pendent dodecyl side chains that exhibited switchable domains. The mobility of molecular chains in the switchable domains acted as a molecular switch for providing access to the encapsulated metal nanoparticles. As outlined in Scheme 1, two opposing factors can be involved in the switchable domains. The increased mobility of molecular chains with increasing temperature intended to provide access to the encapsulated metal nanoparticles (cf. Route A). This access was, however, terminated by a further increase in the temperature, arising from the significantly increased hydrophobicity (cf. Route C). As a result, a compromise was reached under a modest temperature conditions (cf. Route B) in response to the relative balance between the mobility of molecular chains and the hydrophobicity in the switchable domains. In this way, this polymer microreactor demonstrated the autonomic “off–on–off” catalytic ability. The objective of this study is to demonstrate that the potential of polymer microreactors that work at a specified temperature can be realized by using this novel protocol, which opens up the opportunity to develop smart polymer reactors for controlled catalytic processes.
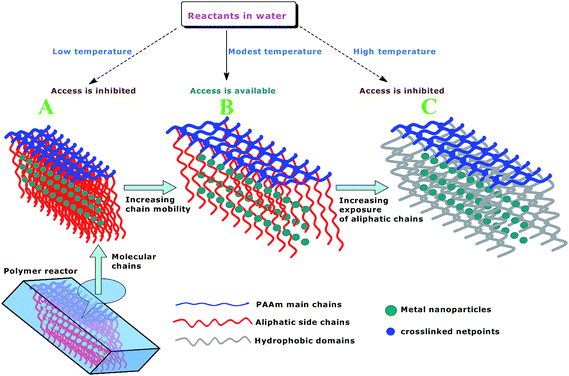 |
| Scheme 1 Proposed mechanism for the NiPR-S microreactor. | |
2. Experimental section
2.1. Preparation of polymer microreactors
Unless otherwise noted, the chemicals used were of analytic grade and used as received from Sigma-Aldrich. The preparation of the polymer microreactor, as outlined in Scheme 1, was based on the classic design of polymer microreactors8 and SMPs.9 In detail, N-dodecylacrylamide (0.24 g; 1 mmol), acrylamide (1.0 g; 14 mmol), N,N′-methylenebisacrylamide (0.12 g; 0.75 mmol), and AIBN (0.10 g), along with nickel nitrate hexahydrate (1.10 g; 3.7 mmol), were dissolved in dimethyl sulfoxide (6.5 mL). After being dispersed and deoxygenated with sonication and nitrogen, the mixture system was heated up to 65 °C and allowed to react for 2 h. The encapsulated ionic nickel was then reduced by an excess of sodium borohydride (tenfold, with regard to ionic nickel). The resulting polymer microreactor (i.e., NiPR-S) was thoroughly washed with ethanol and water, cut into pieces and then dried under flowing nitrogen.
For a comparative study, two controls, i.e., “NiPR-N” and “PR-S”, were also prepared under comparable conditions. NiPR-N was a non-responsive nickel microreactor prepared as NiPR-S but replacing N-dodecylacrylamide with acrylamide (herein the ‘N’ suffix in NiPR-N denotes a non-responsive polymer in contrast to the switchable polymer, denoted by ‘S’). PR-S was the polymeric carrier of NiPR-S and was prepared without using nickel. For the sake of convenient discussion, all of the prepared polymer reactors and carriers are mentioned henceforth as the conceptual microreactors (but not specified by microreactors or carrier).
2.2. Characterization
The TEM images of the as-prepared microreactors were obtained using a transmission electron microscope (TEM) (JEM-2100, Japan). The infrared spectra were recorded using a FTIR apparatus (Nicolet MX-1E, USA). The change in shape with temperature was recorded using a digital camera, where the slice of polymer microreactors were taken out from hot water and immediately photographed (25 °C, unless otherwise noted).
2.3. Thermal transition behavior
The thermal transition behavior in the switchable domains was studied as a function of temperature by using dynamic light scattering (DLS) (Bettersize-2000, China). In order to allow for equilibrium to be reached, all samples were kept at the specified temperatures for at least 10 min before acquiring the hydrodynamic radius (Rh). By comparing the switchable microreactors (i.e., NiPR-S and PR-S) with the non-responsive NiNR-N, the contribution of the thermal transition can be reflected by the change of Rc:10
where, Rd is the particle size of dried particles. ‘S’ represents the switchable microreactors and ‘N’ indicates the non-responsive microreactor.
2.4. Catalysis tests
The catalytic properties of the prepared microreactors were evaluated in a batch format, using the classic model reaction of reducing methylene blue (MB) with NaBH4.11 MB was added into a NaBH4 aqueous solution with an initial concentration of 0.05 mmol mL−1 (totally 4 mL; NaBH4, 20 fold with regard to MB). The solid content of microreactors in the reaction system was 2.5 mg mL−1. The reduction of MB was monitored spectrophotometrically. The catalytic activity of these microreactors was obtained from the average of three runs.
2.5. Electrochemical tests
Electrochemical tests were further employed to obtain information on the switching behavior in the prepared polymer microreactors.12 Using an electrochemical workstation (CHI760E, China), a Pt wire encircled by a thin film of the polymer reactors (ca. 0.05 mm) was adopted as the working electrode in a three-electrode configuration (Au-plate counter electrode and Ag/AgCl ref. electrode). The redox process of HPO42−/H2PO4− (i.e., PBS; pH 7.0) was used as a probe to record the current change upon changing temperature.
3. Results and discussion
3.1. Analysis of composition and structure
The polymer microreactor NiPR-S, as aforementioned, was made of nickel nanoparticles and a unique shape-memory polymer consisting of poly(acrylamide) and pendent aliphatic side chains. FTIR was first used to characterize the composition of this polymer microreactor, as shown in Fig. 1. Three major bands (2900–3700, 1600–1750, and 1000–1500 cm−1) appeared in the spectrum of this polymer reactor. These bands were complex due to the complicated composition, which may be associated with the stretching of O–H/N–H, C
O, and C–N/C–C.13 For a comparative study, we also included the two controls described above in Fig. 1. PR-S exhibited almost the same spectrum as NiPR-S, whereas that of NiPR-N failed to exhibit a spectrum similar to NiPR-S. The same spectra between PR-S and NiPR-S can be ascribed to the comparable composition between PR-S and the polymer carrier of NiPR-S. The difference between NiPR-S and NiPR-N at 1000–1500 cm−1 may be ascribed to the presence of the aliphatic side chains within NiPR-S. Fig. 2 and 3 present the TEM images and a digital image of NiPR-S, respectively. Nickel nanoparticles with a size of ca. 50 nm were encapsulated in the polymeric building blocks. Thus, this novel polymer reactor was prepared in the desired form.
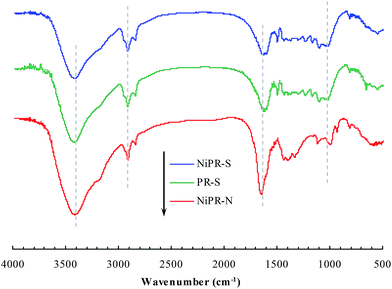 |
| Fig. 1 FTIR spectra of the prepared polymer microreactors. | |
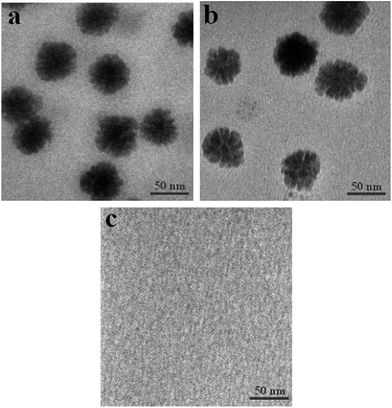 |
| Fig. 2 TEM images of metal nanoparticles present in the prepared polymer microreactors ((a): NiPR-S; (b): NiPR-N; (c): PR-S). | |
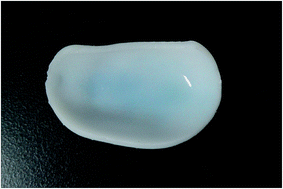 |
| Fig. 3 Digital image in the outward appearance of a slice of NiPR-S. | |
3.2. Thermal transition behavior and shape-memory effect
Using DLS, the thermal transition behavior in the switchable domains was studied as a function of temperature. As shown in Fig. 4, NiPR-S and PR-S revealed a significant dependence upon temperature in contrast to NiPR-N. The maximal value of Rc in NiPR-S appeared at ca. 43 °C. Below this temperature, NiPR-S revealed a low Rc associated with the low mobility of molecular chains in the switchable domains, which inhibited swelling of the polymer. Above this temperature, NiPR-S also revealed a low Rc, in response to the increased exposure of the hydrophobic side chains. A maximal value of Rc was reached at the transition temperature (i.e., 43 °C). This result suggests that the switchable properties have been incorporated into the prepared polymer microreactors.
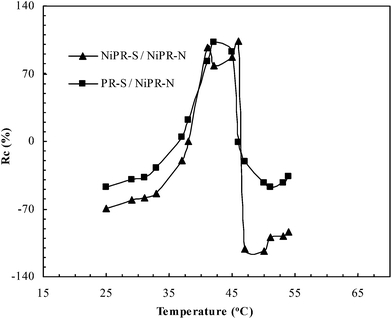 |
| Fig. 4 DLS curves of the prepared polymer microreactors. | |
To further address the switchable properties in NiPR-S, Fig. 5 displays the shape-memory effect. A rod-like slice (cf. a) was bent by loading above the transition temperature (i.e., 50 °C), followed by cooling to room temperature (i.e., ∼25 °C), which fixed the bent shape (cf. b). Heating this polymer again above the transition temperature resulted in the recovery of the rod-like shape (cf. c and d). The recovering behavior was repeatable and the system can be made to switch between the initial and the temporary shapes. In conjunction with the study in Fig. 4, this result further suggests the switchable properties have been incorporated into the prepared polymer microreactors.
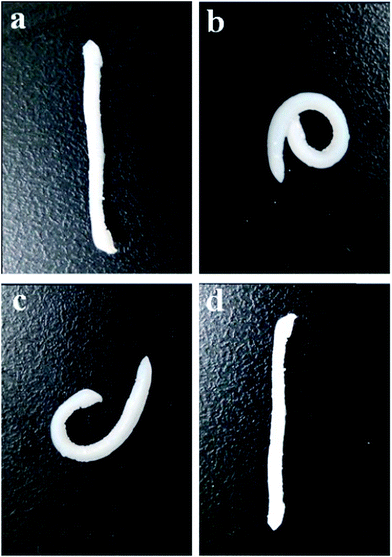 |
| Fig. 5 The shape-memory effect of NiPR-S ((a): initial shape; (b): fixed temporary shape (polymer was re-shaped at 50 °C, followed by quenching to 25 °C); (c): shape recovering (at 50 °C); (d): recovered permanent shape). | |
3.3. Switchable catalysis
The catalytic properties of these polymer microreactors are presented in Fig. 6. PR-S did not exhibit significant catalysis due to the lack of catalytic nickel nanoparticles. In contrast, NiPR-S and NiPR-N showed significant catalytic ability, where the conversion increased rapidly with time. To verify the switchable catalysis, three representative temperatures, i.e., 30, 43 and 50 °C (lower, equal to or higher than the transition temperature of NiPR-S, respectively), were selected for a comparative study. In the control microreactor NiPR-N, the catalytic activity increased with increasing temperature. In contrast, the catalytic activity of NiPR-S underwent an initial increase and then a decrease, reaching a maximal value at 43 °C. Specifically, NiPR-S at both 30 °C and 50 °C showed a lower catalytic activity than NiPR-N and nonetheless a higher activity than NiPR-N at the transition temperature (i.e., 43 °C). NiPR-S, therefore, demonstrated the switchable catalysis in an ‘off–on–off’ paradigm, as desired.
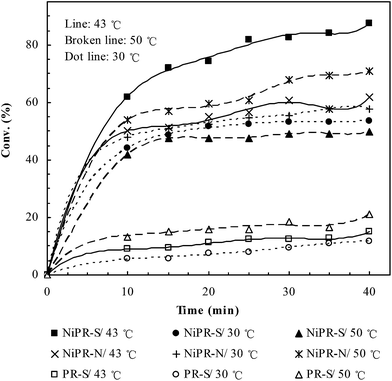 |
| Fig. 6 Catalytic activity of the prepared polymer microreactors. | |
3.4. Access-regulating behavior
Electrochemical tests were further employed to achieve information on the switching behavior in the prepared polymer microreactors.12 By a comparison between the switchable microreactors (i.e., NiPR-S and PR-S) and the non-responsive NiPR-N, the effect of the switchable properties on the access to the polymer inner was thus exposed. As shown in Fig. 7, NiPR-N revealed an increase in current with increasing temperature (cf. b). In contrast, the current in NiPR-S and PR-S underwent an initial increase and then a decrease, reaching a maximal value at the transition temperature, i.e., 43 °C (cf. a and b). Specifically, NiPR-S at both 30 °C and 50 °C revealed a smaller current value than that of NiPR-N and nonetheless a higher current value than that of NiPR-N at 43 °C. This result suggests that switching of the aliphatic domains regulated access to the polymer interior in NiPR-S and PR-S. In conjunction with the catalytic study (Fig. 6), this result further suggests that the switchable properties in these polymer microreactors allows fine tuning of the access of substrate to the encapsulated metal nanoparticles, which thereby makes switching of the catalytic activity feasible.
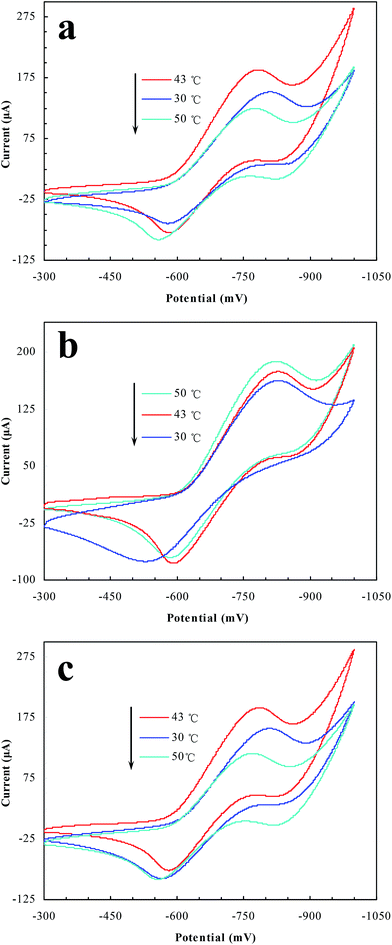 |
| Fig. 7 CV profiles with tunable access to the polymer interior of the prepared microreactors ((a): NiPR-S; (b): NiPR-N; (c): PR-S). | |
4. Conclusions
An originally designed autonomic and “off–on–off”-switchable polymer microreactor is reported in this study. This polymer microreactor was constructed from nickel nanoparticles and an unique shape-memory polymer consisting of poly(acrylamide) and pendent dodecyl side chains that behaved as thermally switchable domains. The mobility of molecular chains in the switchable domains acted as a molecular switch for tuning of the access of substrate to the encapsulated metal nanoparticles. Below the transition temperature (∼34 °C), this polymer microreactor revealed weak reactivity due to the low mobility of molecular chains in the switchable domains, which inhibited access to the encapsulated nickel nanoparticles. Above the transition temperature, this polymer microreactor revealed weak reactivity, due to the significantly increased hydrophobicity in the switchable domains. This polymer microreactor only provided significant catalysis under a modest temperature condition, resulting from the compromise reached at the transition temperature. In this way, this polymer microreactor demonstrated the autonomic “off–on–off” catalytic ability. It is therefore confirmed that polymer microreactors that can work at a specified temperature can be prepared using this novel protocol, which opens up the opportunity to develop smart polymer microreactors for controlled catalytic processes. Future development in this field will significantly increase the applications and will lead to the appearance of novel catalytic materials and functional catalysts.
Acknowledgements
The authors want to express their gratitude to the National Science Foundation of China (nos 51473070, 21403091). We also thank the Jiangsu Province and Jiangsu University for support under the distinguished professorship program (Sujiaoshi [2012]34, no. 12JDG001) and the innovation/entrepreneurship program (Suzutong [2012]19). The authors also appreciate the project support from the Science and Technology Agency of Jiangsu Province (BK20130486).
References
- M. Pérez-Lorenzo, B. Vaz, V. Salgueiriño and M. A. Correa, Chem.–Eur. J., 2013, 19, 12196–12211 CrossRef PubMed.
- Y. Zhou, M. Zhu and S. Li, J. Mater. Chem. A, 2014, 2, 6834–6839 CAS.
- C.-W. Chen, M.-Q. Chen, T. Serizawa and M. Akashi, Adv. Mater., 1998, 10, 1122–1126 CrossRef CAS.
- Y. Lu, Y. Mei, M. Drechsler and M. Ballauff, Angew. Chem., Int. Ed., 2006, 45, 813–816 CrossRef CAS PubMed.
- B. M. Nestl, S. C. Hammer, B. A. Nebel and B. Hauer, Angew. Chem., Int. Ed., 2014, 53, 3070–3095 CrossRef CAS PubMed.
- T. Xie, Nature, 2010, 464, 267–270 CrossRef CAS PubMed.
- A. Lendlein and S. Kelch, Angew. Chem., Int. Ed., 2002, 41, 2034–2057 CrossRef CAS.
- B. Peng, X. Yuan, M. Zhu and S. Li, Polym. Chem., 2014, 5, 562–566 RSC.
- J. Hu, Y. Zhu, H. Huang and J. Lu, Prog. Polym. Sci., 2012, 37, 1720–1763 CrossRef CAS PubMed.
- S. Li, Y. Ge, A. Tiwari and S. Cao, Small, 2010, 6, 2453–2459 CrossRef CAS PubMed.
- Y. Zheng and A. Wang, J. Mater. Chem., 2012, 22, 16552–16559 RSC.
- S. Li, Y. Ge and A. P. F. Turner, Adv. Funct. Mater., 2011, 21, 1194–1200 CrossRef CAS.
- W. Wang, X. Tian, Y. Feng, B. Cao, W. Yang and L. Zhang, Ind. Eng. Chem. Res., 2010, 49, 1684–1690 CrossRef CAS.
|
This journal is © The Royal Society of Chemistry 2015 |
Click here to see how this site uses Cookies. View our privacy policy here.