DOI:
10.1039/C4RA07825J
(Paper)
RSC Adv., 2014,
4, 60920-60928
Click synthesis of graphene/poly(N-(2-hydroxypropyl) methacrylamide) nanocomposite via “grafting-onto” strategy at ambient temperature
Received
30th July 2014
, Accepted 23rd October 2014
First published on 29th October 2014
Abstract
So far, much work has been performed to modify graphene by physical or chemical methods, aiming to improve its solubility in solvents for its further application. Herein, we reported a new graphene/polymer nanocomposite, a graphene/poly(N-(2-hydroxypropyl) methacrylamide) (PHPMA) nanocomposite (G-PHPMA), by combining reversible addition–fragmentation chain transfer (RAFT) polymerization and click chemistry under mild conditions via the “grafting-onto” strategy. The as-prepared azido-terminated PHPMA homopolymer via RAFT polymerization was grafted onto yne-containing graphene through click chemistry at room temperature using CuBr/PMDETA as the catalytic system. FT-IR, XRD, Raman, element analysis, TGA, AFM, and TEM measurements were used to confirm the covalent linkage between PHPMA chains and graphene. The resulting G-PHPMA nanocomposite showed excellent dispersibility in organic solvents and aqueous media and it could quickly enter into SMMC-7721 and SH-SY5Y cells.
Introduction
Graphene, a single-atom-thick sheet of sp2 bonded carbon atoms densely packed in a honeycomb crystal lattice, has been considered as one of the most promising materials of the future,1 because it possesses unique thermal, electric, and mechanical properties originating from its structure.2–4 Thus, it has showed great potential applications in transistors, supercapacitors, energy storage devices, electrodes, drug delivery, and polymer composites.5–7 Moreover, graphene-based polymeric materials also triggered enormous interest for their extremely high thermal conductivity and strong mechanical properties.8
Graphene, however, tends to aggregate in an almost irreversible manner due to its strong tendency for van der Waals interactions and high specific surface area, which limits its further process or applications.9 Numerous methods,10–13 mainly including covalent modifications and non-covalent interactions, have been developed to control the dispersion of graphene, prevent re-aggregation, and enhance compatibility with receiving host matrix. Because of the inherent instability of the resulting supramolecular systems, it is difficult to control the structure of graphene modified by non-covalent method.14,15 On the other hand, covalent modifications are more dominant of achieving permanent stabilization of graphene sheets and also endowing graphene with superior performance.16,17
Recently, covalent modification of graphene with polymers has attracted great attentions because graphene/polymer composites can improve the solubility of graphene and the versatility of polymers allows for control over the final properties of functionalized graphene.7,18 In general, graphene/polymer composites can be achieved by two different strategies of “grafting-onto”19 and “grafting-from”.20 The “grafting-onto” approach has been proved to be an effective strategy for the covalent modification of graphene with as-prepared polymer with certain molecular weight and polydispersity. For example, Sun et al. covalently grafted azido-terminated polystyrene prepared by ATRP onto graphene oxide (GO) sheets using Cu(I)-catalyzed 1,3-dipolar azide/alkyne cycloaddition reaction in DMF at room temperature.21 Also, Pan et al. used a very similar approach to prepare GO functionalized with poly(N-isopropylacrylamide) (PNIPAM) also replying on the synthesis of azido-terminated polymer (initially prepared by ATRP).22 Additionally, our group functionalized GO sheets by using the “grafting-onto” strategy of PNIPAM prepared by SET-LRP.23 The grafting-onto step was based on atom transfer nitroxide radical coupling reaction (ATNRC) and the obtained graphene/PNIPAM composites showed excellent dispersibility in a wide range of polar and nonpolar solvents. However, ATRP or SET-LRP can only be applied to polymerize a few portion of monomers, and can not be employed to prepare polymers with carboxyls, and even has bad control of chain growth for certain polymers; furthermore, reaction conditions of ATRP or SET-LRP are relatively strict and reagents, like CuX and ligands, are usually expensive.24,25 Thus, it is meaningful to develop a general and economical method with good distribution of molecular weight and efficient coupling efficiency to covalently modify graphene via the “grafting-onto” strategy.
Reversible addition–fragmentation chain transfer (RAFT) polymerization has been evidenced as one of the most effective living/controlled radical polymerization methods.26,27 In comparison with other living/controlled radical polymerization methods, such as ATRP and SET-LRP, metal-free RAFT polymerization has amazing versatility towards good tolerance for a wide range of functionalities and excellent control over polymer topology and architectures. Because of the versatility of RAFT reagents, lots of modifications can be made for RAFT reagents by introducing functionalities for the synthesis of certain polymers with reactive groups on chain end. So far, RAFT polymerization has been widely used for designing block copolymers, graft copolymers, branched and dendrimic topologies.28,29 Additionally, click chemistry, especially Cu(I)-catalyzed 1,3-dipolar azide/alkyne cycloaddition reaction, has gained lots of attention because of excellent functionality tolerance, high specificity, and nearly quantitative yield under mild experimental conditions;30 and a variety of functional polymers and materials with different structures has been prepared on the basis of click chemistry.31,32
Herein, we tried to employ RAFT polymerization and click chemistry to offer an efficient and universal method for the synthesis of graphene/polymer composites. In this article, we firstly utilized diazonium addition to introduce yne-containing groups onto graphene sheets to provide yne-containing graphene (G-yne). Meanwhile, azido-terminated poly(N-(2-hydroxypropyl) methacrylamide) (PHPMA–N3) was synthesized through RAFT homopolymerization using an azido-based chain transfer agent (CTA-N3). Finally, the linkage between PHPMA and graphene was achieved by azide/alkyne click chemistry with CuBr/PMDETA as catalytic system at ambient temperature as shown in Scheme 1. Obviously, this thought has paved a new path to prepare graphene/polymer composites.
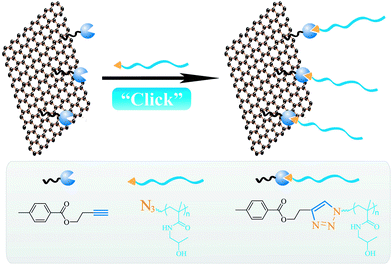 |
| Scheme 1 Preparation of G-PHPMA nanocomposite via click chemistry combining with the grafting-onto strategy. | |
Experimental
Materials
Copper(I) bromide (CuBr, Aldrich, 98%) was purified by stirring overnight over CH3CO2H at room temperature, followed by washing the solid with ethanol, diethyl ether, and acetone prior to drying at 40 °C in vacuo for 1 day. Graphite powder (Aldrich, 99.99+%), fluorescein isothiocyanate (FITC, Aldrich, 90%), sulfuric acid (H2SO4, Aldrich, 95–98%), potassium permanganate (KMnO4, Aldrich, 99%), 2-(4-aminophenyl)ethanol (Aldrich, 98%), hydrazine hydrate solution (Aldrich, 78–82%), N,N,N′,N′′,N′′-pentamethyldiethylenetriamine (PMDETA, Aldrich, 99%), isoamyl nitrite (Acros, 97%), and N,N-dimethylformamide (DMF, Aldrich, 99.8%) were used as received. S-1-Dodecyl-S′-(α,α′-dimethyl-α′′-3-azido-1-propyl acetate) trithiocarbonate22 was synthesized by esterification of S-1-dodecyl-S′-(α,α′-dimethyl-α′′-acetic acid) trithiocarbonate33 and 3-azido-1-propanol.34 But-3-ynyl 4-aminobenzoate35 and N-(2-hydroxypropyl) methacrylamide (HPMA)36 were prepared according to previous literatures.
Measurements
FT-IR spectra were recorded on a Nicolet AVATAR-360 FTIR spectrophotometer with a 4 cm−1 resolution. UV/vis spectra were taken using a Hitachi U-2910 spectrophotometer. X-ray diffraction (XRD) measurements were performed on a Philips X'Pert PRO X-ray powder diffractometer with CuKα (1.541 Å) radiation (40 kV, 40 mA) and the sample was exposed at a scan rate of 2θ = 0.0334° s−1 in the range between 3° and 85°. Unpolarized Raman scatterings were recorded performed on a Jobin Yvon LabRAM HR 800 UV micro-Raman spectrometer (514.5 nm line from an Ar+ laser) at room temperature. Elemental analysis was carried out on a CarloErba 1106 system. Relative molecular weight and molecular weight distribution were measured by conventional gel permeation chromatography (GPC) system equipped with a Waters 1515 Isocratic HPLC pump, a Waters 2414 refractive index detector, and a set of Waters Styragel columns (HR3 (500–30
000) and HR4 (5000–600
000), 7.8 × 300 mm, particle size: 5 μm). GPC measurements were carried out at 40 °C using LiBr-added DMF ([LiBr] = 50 mM) as eluent (flow rate: 1.0 mL min−1). The system was calibrated with linear poly(methyl methacrylate) standards. Thermogravimetric analysis (TGA) measurements were run on a TA Q500 system under N2 purge with a heating rate of 10 °C min−1. Atomic force microscopy (AFM) images were taken by a Veeco DI MultiMode SPM in the tapping mode of dropping the sample solution onto freshly exfoliated mica substrate. Transmission electron microscope (TEM) images were obtained by a JEOL JEM 2100F instrument operated at 200 kV. Zeta potential was measured by a Malvern Nano-ZS90 Zetasizer. Cellular uptake images were taken by a Nikon Eclipse TI fluorescence microscope.
Preparation of single-layer GO sheets
Exfoliated GO sheets were prepared by a modified Hummer's method37 as shown in Scheme 2. Graphite powder was oxidized by neat H2SO4 and KMnO4 with three different stages and followed by filtration and subsequent dialysis against deionized water for several days to remove residual salts and acids. Finally, the obtained GO dispersion (0.1 mg mL−1) was exfoliated by water-bath ultrasonication for 3 hours.
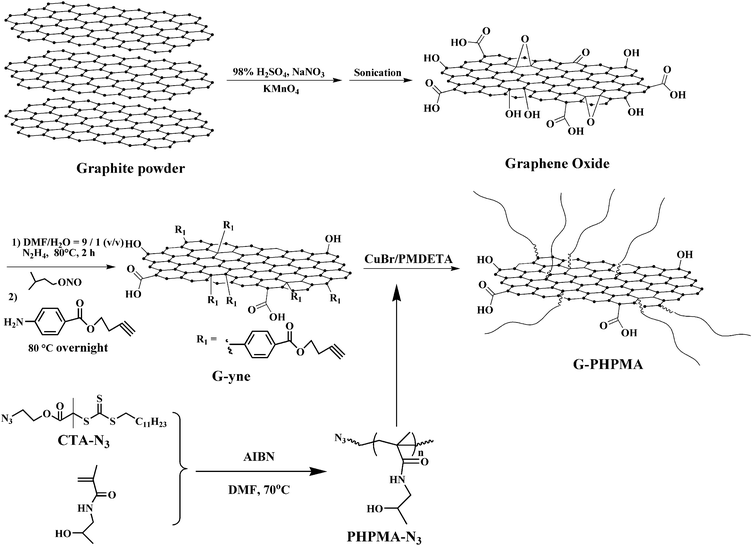 |
| Scheme 2 Synthetic route of G-PHPMA nanocomposite. | |
Preparation of yne-containing graphene
Diazonium addition: GO (200 mg) was firstly dispersed in deionized water followed by adding hydrazine hydrate (0.5 mL) solution gradually for stirring at 100 °C for 4 hours. Next, but-3-ynyl 4-aminobenzoate (2.00 g) and isoamyl nitrite (4.0 mL) were introduced under N2 and the solution was kept stirring vigorously at 80 °C overnight. The mixture was filtrated by a 0.22 μm filter and the residual solid was washed with deionized water and DMF. The remaining black solid, G-yne, was dried in vacuo at 40 °C overnight.
RAFT homopolymerization of N-(2-hydroxypropyl) methacrylamide
HPMA (3.00 g, 21 mmol), AIBN (10.0 mg, 0.061 mmol), S-1-dodecyl-S′-(α,α′-dimethyl-α′′-3-azido-1-propyl acetate) trithiocarbonate (123 mg, 0.275 mmol), and dry DMF (5.0 mL) were added to a 100 mL flask (flame-dried under vacuum prior to use) sealed with a rubber septum under N2. The system was degassed by three cycles of freezing–pumping–thawing followed by stirring at room temperature for 10 min. Finally, the flask was immersed into an oil bath set at 70 °C to start the polymerization. RAFT homopolymerization of HPMA lasted 12 h and was terminated by putting the flask into liquid N2. The slightly yellow product, PHPMA–N3, was collected by precipitating into diethyl ether for three times and dried in vacuo overnight. GPC: Mn = 15
500 g mol−1, Mw/Mn = 1.19. FT-IR: ν (cm−1): 3384, 2968, 2929, 2110 (–N3), 1637 (amide), 1538, 1460, 1267, 1205, 1091, 956, 806, 736, 697.
1,3-Dipolar azide/alkyne click chemistry between G-yne and PHPMA–N3
PHPMA-functionalized graphene was prepared by linking alkyne-functionalized graphene sheets and azido-terminated PHPMA via click chemistry. To a 100 mL flask (flame-dried under vacuum prior to use) sealed with a rubber septum, PHPMA–N3 (1.00 g), G-yne (42 mg), CuBr (8.8 mg, 0.06 mmol), and dry DMF (20 mL) were added under N2, and the system was then sonicated for 1 h. PMDETA (12 μL, 0.06 mmol) was introduced via a gas tight syringe followed by immersing the flash into an oil bath set at 25 °C. The coupling reaction lasted one day and the crude product was diluted with excess DMSO followed by filtering through a 0.22 μm filter and washing exhaustively with deionized water and DMF. The obtained black solid, G-PHPMA, was dried in vacuo at 40 °C overnight.
Cell culture
The human hepatoma cell line SMMC-7721 and neuroblastoma cell line SH-SY5Y were supplied by Shanghai Institute of Cell Biology, Chinese Academy of Sciences. They were cultured at 37 °C under a humidified 5% CO2 atmosphere in RPMI-1640 or DMEM medium (GIBCO/Invitrogen, USA) supplemented with 10% fetal bovine serum (FBS, BI Biological Industries Ltd., Israel) and 1% penicillin–streptomycin (10
000 U mL−1 penicillin and 10 mg mL−1 streptomycin, Solarbio Life Science, China).
Cellular uptake of G-PHPMA
G-PHPMA was labeled by FITC by mixing 1 mL of FITC aqueous solution (0.3 mg mL−1) with 10 mL of G-PHPMA aqueous suspension (0.5 mg mL−1). Free FITC was removed by dialysis against double-distilled water for 48 h. The resulting G-PHPMA/FITC nano-complex was stored at 4 °C. SMMC-7721 and SH-SY5Y cells were plated on a 20 mm glass round cover slip in 6-well plates, and allowed to adhere overnight. The cells were incubated with G-PHPMA/FITC nano-complex for 6 h followed by washing with phosphate buffered saline (PBS) three times. The cells were then imaged under an inverted Nikon Eclipse TI fluorescence microscope.
Results and discussion
Preparation and characterization of azido-terminated PHPMA
Since the discovery of RAFT polymerization, this approach has been considered as one of the most effective methods for the preparation of polymers because of its wide range of monomers, simple polymerization operations, good tolerance of functional groups, and excellent control of polymer topology. The reaction mechanism of this method was continuously processed by activation and deactivation generated by thioesters as chain transfer agents, making the concentration of radical in the system maintain at a very low level so as to ensure living/controlled growth of polymer chains. In addition, RAFT reagent can be modified by introducing certain groups to synthesis of some polymers with functionalities on chain end. Here, we intended to prepare S-1-dodecyl-S′-(α,α′-dimethyl-α′′-3-azido-1-propyl acetate) trithiocarbonate, a specific RAFT reagent with azido functionality on one end, and followed by being employed for synthesis of azido-terminated PHPMA homopolymer, PHPMA–N3. This azido-terminated PHPMA was characterized by GPC and FT-IR. GPC measurement was performed at 40 °C using LiBr-added DMF as eluent. It can be seen from Fig. 1 that PHPMA–N3 was a well-defined polymer showing a unimodal and symmetrical retention peak with a high Mn of 15
500 g mol−1 and a narrow molecular weight distribution of 1.19. The sharp peak at 1637 cm−1 in FT-IR spectrum as shown in Fig. 2 was attributed to the stretching vibration of amide group while the peaks at 2968 and 2929 cm−1 were originated from the asymmetrical stretching vibration of CH3 and CH2 groups. respectively. In particular, the weak signal at 2110 cm−1 was attributed to the stretching vibration of azido group, which confirmed the existence of azido end group in PHPMA chain after RAFT homopolymerization. These evidences clearly suggested the successful preparation of azido-terminated well-defined PHPMA for providing premise for the succedent coupling reaction.
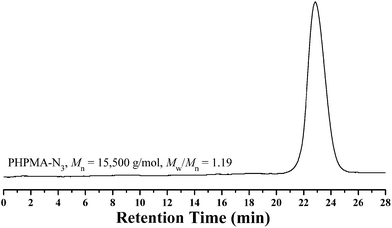 |
| Fig. 1 GPC trace of azido-terminated PHPMA homopolymer in LiBr-added DMF. | |
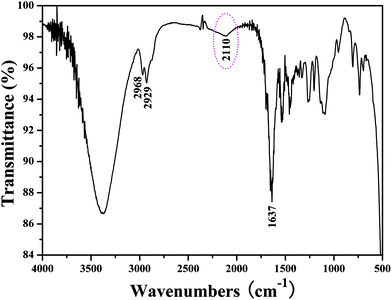 |
| Fig. 2 FT-IR spectrum of azido-terminated PHPMA homopolymer. | |
Introduction of yne-containing groups onto graphene sheets
Graphene oxide obtained by sonicating graphite oxide in water can be stable for several weeks without precipitation, for it contains three different kinds of oxygen-containing groups on its surface and edge including carboxyl, epoxy, and hydroxyl groups produced by oxidation process. Because of the existence of these oxygen-containing groups, GO usually was used as staring material for further modification. However, these oxygen-containing groups are labile and would easily decompose with heating; on the other hand, grafting of certain polymers requires the presence of non-oxygenated functional groups on graphene. Therefore, it is necessary to find alternative strategies with additional chemical reactions for the development of the modification of GO surfaces with desired functional groups before the grafting of polymers.
Considerable efforts have been done to pre-modify graphene with certain reactive groups before grafting polymers.12 Among these methods, diazonium addition reaction has been regarded as one of effective ways to introduce reactive groups on graphene sheets. For instance, Chen et al. utilized diazonium addition reaction to introduce Br-containing initiating groups onto the surface of graphene for the following in situ SET-LRP.38 Inspired by this example, we tried to employ this method to introduce yne-containing groups onto graphene in the current case (Scheme 2).
Fig. 3 exhibited XRD patterns of pristine graphite, graphene oxide, and G-yne. The interlayer distance can be easily calculated from the diffraction angle of the corresponding material according to Bragg's equation: nλ = 2d
sin
θ. Pristine graphite showed a sharp peak at 26.5° with an interlayer distance of 3.36 Å while GO showed a strong peak at 10.4° with an interlayer distance of 8.50 Å, indicating the expansion of interlayer space of graphene sheets. This result was mainly ascribed to covalent bonds and the repulsion of oxygen-containing groups on graphene sheets. However, after treating with the reductant of N2H4, G-yne showed a peak at about 23° with an interlayer distance of 3.86 Å, which was close to that of pristine graphite, suggesting the chemical reduction of GO; on the other hand, this difference between G-yne and pristine graphite illustrated that certain groups had been successfully grafted onto graphene sheets. Moreover, two characteristic peaks attributed to the presence of ester bond which were absent in FT-IR spectrum of GO (Fig. 4), were found to appear at 1653 and 1100 cm−1 in FT-IR spectrum after the diazonium addition reaction as shown in Fig. 4, which clearly witnessed the successful grafting of yne-containing groups onto graphene sheets because alkynyl was connected to graphene sheets via ester linkage.
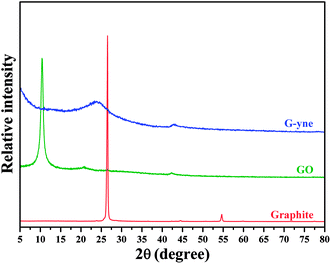 |
| Fig. 3 XRD patterns of pristine graphite, GO, and G-yne. | |
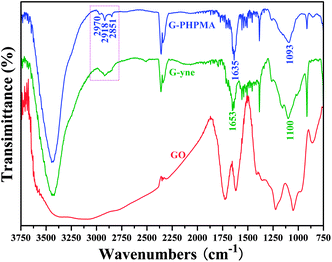 |
| Fig. 4 FT-IR spectra of GO, G-yne, and G-PHPMA. | |
Preparation of G-PHPMA nanocomposites via click chemistry
Click chemistry is mainly focused on highly selective and simple orthogonal reactions that do not yield side products, and it gives heteroatom-linked molecular systems with high efficiency under a variety of mild conditions. Among the diverse click approaches, Cu-catalyzed 1,3-dipolar azide/alkyne click reaction in particular has received the most attention, with applications extending to the synthesis of functional block copolymer, dendrimer preparation, synthesis of uniformly structured hydrogel, and many other polymers with different topologies.
In the present work, we employed azide/alkyne click chemistry to graft the as-prepared azido-terminated PHPMA onto G-yne with CuBr/PMDETA catalytic system at room temperature to obtain PHPMA-modified graphene nanocomposite, G-PHPMA. The attached PHPMA polymeric chains on the graphene sheets were first verified by FT-IR (Fig. 4). The sharp peak at 1635 cm−1 was ascribed to the stretching vibration of amide group of PHPMA chains, which was obviously different from the signal of ester bond at 1653 cm−1 in FT-IR spectrum of G-yne. In addition, a new weak corresponding to the asymmetrical stretching vibration of CH3 group appeared at 2970 cm−1 after the click reaction, which was absent before the reaction. These evidences clearly illustrated that well-defined PHPMA chains had been successfully attached onto the graphene sheets by Cu-catalyzed 1,3-dipolar azide/alkyne click reaction.
Fig. 5 displayed Raman spectra of pristine graphite, GO, G-yne, and G-PHPMA, and each sample showed two prominent peaks corresponding to D and G bands in its own spectrum. Generally, G band around 1570 cm−1 is ascribed to sp2 hybridization in a graphitic two dimensional hexagonal lattice while D band around 1350 cm−1 arises from sp3 hybridization (C atoms of defects and disorder). It was found that G band peaks of GO, G-yne, and G-PHPMA (1580–1586 cm−1) had an obvious 12–18 cm−1 blue-shift compared to that of pristine graphite (1568 cm−1) because of the influence of defects and isolated double bonds produced by reduction process. On the other hand, we still found two distinct peaks existing in Raman spectrum of G-yne, that is to say, although GO was treated with reducing reagent, it was hard to completely restore GO into graphene only with simple peak of in Raman spectrum, which partly suggested that some oxygen-containing groups still remained on the graphene sheets after reduction for providing repulsive force to prevent the aggregation of graphene sheets. Furthermore, the intensity ratio of D and G band (ID/IG) was used to speculate the information about the disordered and ordered domains of graphene. The ID/IG ratio of G-yne was 1.25 while that of G-PHPMA was lowered to 1.14 because the grafting of PHPMA chains reduced the defects and isolated double bonds in-plane domains. This variation also partly illustrated the successful covalent bonding between graphene sheets and PHPMA chains for the preparation of graphene/PHPMA composite.
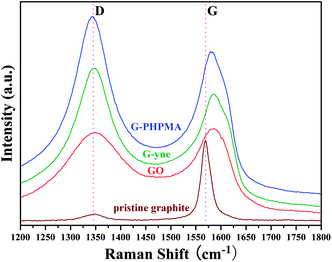 |
| Fig. 5 Raman spectra of pristine graphite, GO, G-yne, and G-PHPMA. | |
C, H, and N contents of G-yne and G-PHPMA were determined by element analysis as summarized in Table 1. It can be seen from the table that C/H molar ratio of G-yne was 2.24 while that of G-PHPMA was remarkably decreased to 0.99, which was mainly caused by the introduction of PHPMA chains onto the graphene sheets. Moreover, N content of G-PHPMA (7.28 wt%) was much higher than that of G-yne (4.90 wt%). Both differences clearly indicated that PHPMA polymer brushes had been grafted onto the graphene sheets.
Table 1 Element analysis of G-yne and G-PHPMA
Samples |
C (wt%) |
H (wt%) |
N (wt%) |
C/H molar ratio |
G-yne |
75.35 |
2.80 |
4.90 |
2.24 |
G-PHPMA |
63.60 |
5.34 |
7.28 |
0.99 |
The amount of grafted PHPMA polymer on graphene sheets was determined by TGA as shown in Fig. 6. The mass loss of GO below 200 °C was attributed to the pyrolysis of oxygen-containing groups on its plane. The thermal stability of G-yne obtained through diazonium addition reaction during chemical reduction was improved with slight decomposition in comparison with that of GO and the residual mass of G-yne at 750 °C was 62.51 wt%. After PHPMA chains were covalently grafted onto graphene sheets, the resulting G-PHPMA showed a significant thermolysis in the range of 300 °C to 450 °C, originating from the decomposition of PHPMA brushes. Assuming that the weight loss during the thermolysis of G-PHPMA was due entirely to the removal of the grafted PHPMA polymer chains, the grafting content of PHPMA, 24.23 wt% (=62.51–38.38 wt%), was estimated from the residual mass of G-PHPMA at 750 °C (38.38 wt%) after the decomposition.
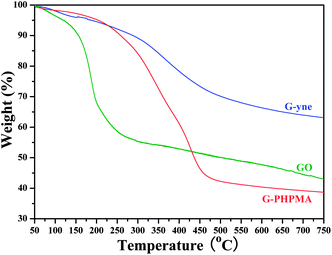 |
| Fig. 6 TGA (in N2) of GO, G-yne, and G-PHPMA with a heating rate of 10 °C min−1. | |
Surface morphology and dispersibility of G-PHPMA
The successful attaching of PHPMA chains was also examined by visualizing pattern features of the resulting nanocomposite using AFM and TEM. Fig. 7 show AFM images of GO and G-PHPMA, and it was found from Fig. 7A that the average height of GO sheets was 0.89 nm, which was obviously thicker than the well-known van der Waals thickness of pristine graphene sheet (0.34 nm) because of the presence of oxygen-containing groups above and below the graphene plane. The average thickness of G-PHPMA was 5.45 nm evaluated from Fig. 7B, which was much higher than that of GO. This elevated thickness probably confirmed the existence of PHPMA brushes across the surface of graphene sheets, which may be brought about by the swollen appearance and rough surface of polymeric chains on graphene plane or overlapped by a few layers. In view of horizon size, the value of G-PHPMA (430 nm) was much lower than that of as-prepared GO (850 nm) due to the consequential diazonium addition, chemical reduction, and Cu-catalyzed 1,3-dipolar azide/alkyne click reactions and continuous sonication.39 In addition, TEM measurements were performed to further investigate the surface morphologies of GO and G-PHPMA as shown in Fig. 8. GO was found to show a wrinkled topology (Fig. 8A) via distortions caused by the oxygen-containing groups while Fig. 8B showed black spots onto graphene sheets, this indicating PHPMA clusters dispersed on the whole sheet after click reaction. We also did not observe such kind of protuberances in GO sheets. Thus, it can be concluded from aforementioned results that PHPMA chains have been successfully attached onto graphene sheets.
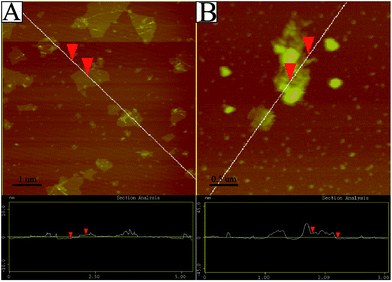 |
| Fig. 7 Tapping mode AFM images with section analysis of GO (A) and G-PHPMA (B). | |
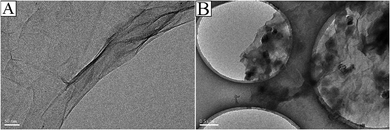 |
| Fig. 8 TEM images of GO (A) and G-PHPMA (B). | |
Generally, graphene possesses very poor solubility and dispersibility in solvents and graphene sheets usually tend to aggregate to form pristine graphite due to the hydrophobic nature of graphene and significant π–π interactions between graphene sheets. Therefore, it is meaningful to improve its solubility through some modification methods. Herein, graphene attached with polymeric clusters, G-PHPMA, was dispersed in regular solvents without surfactants and the dispersions were still very stable even after 30 days as shown in Fig. 9. It was found that G-PHPMA had good solubility or dispersibility in regular organic solvents (except some solvents with low polarity such as hexane) and water with the help of steric repulsive force originating from the long PHPMA chains on the surface of graphene sheets (Fig. 9), which may favor the possibility for its further application. Furthermore, zeta potential of G-PHPMA in aqueous media was found to be −17.7 mV, which was clearly higher than that of GO in aqueous media, −43.9 mV, due to the newly attached neutral PHPMA chains on the surface of graphene sheets. Indeed, there are two fundamental facts, i.e. steric repulsion and electrostatic stabilization, which can affect dispersion stability. The steric repulsion results from the polymers absorbed or attached on the surface of particles, which keep particles separated by steric repulsion between polymer layers. Electrical repulsion between particles carrying electrical charges also can make the particles resist flocculation and endow the particles with stability in solutions. Although the zeta potential decreased as the attachment of PHPMA chains, which made the electrical repulsion between G-PHPMA decreased; however, the attached PHPMA chains would also increase the steric repulsion between G-PHPMA. Thus, G-PHPMA had a high stability in regular organic solvents as shown in Fig. 9.
 |
| Fig. 9 Dispersion of G-PHPMA in various organic solvents with a concentration of about 0.5 mg mL−1, the images were taken after placing 30 days. | |
As it is well-known, PHPMA is a kind of water soluble, non-immunogenic, and non-toxic polymer, which has been frequently used in biomaterial area. In the current case, the covalent linking of PHPMA chains onto the surface of graphene obviously helped improve the dispersibilty in aqueous media. For the application in biomaterial area, water is the most important media. Therefore, we further measured UV/vis spectrum of G-PHPMA nanocomposite in aqueous media with different concentrations (1–100 mg L−1) and the absorption peak was found to be located at 275 nm as shown in Fig. 10. The absorbance at 275 nm increased linearly with the rising of the concentration of G-PHPMA and thus, the absorption coefficient was determined to be 17.49 L g−1 cm−1 from the slope of standard curve (inset of Fig. 10).
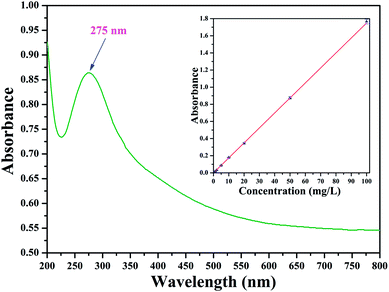 |
| Fig. 10 UV-vis absorbance spectrum of G-PHPMA in aqueous media with a concentration of 50 mg L−1 and UV-vis absorbance standard curve of G-PHPMA at 275 nm (inset). | |
In order to explore the potential application of G-PHPMA nanocomposite in biomaterial area, FITC was used as a fluorescent probe for intracellular imaging40 to check whether G-PHPMA could easily enter cells. FITC was mixed with G-PHPMA in aqueous media to form G-PHPMA/FITC complex.41 The cellular uptake of G-PHPMA/FITC was investigated by incubating SMMC-7721 and SH-SY5Y cells with G-PHPMA/FITC for fluorescence imaging. It can be seen from Fig. 11 that G-PHPMA penetrated cell membranes and entered cells. Thus, we can confirm the cellular uptake of G-PHPMA by SMMC-7721 and SH-SY5Y cells, implying potential application in biomaterial area. Further work of drug delivery using G-PHPMA as nanocarrier is being carried out in our group.
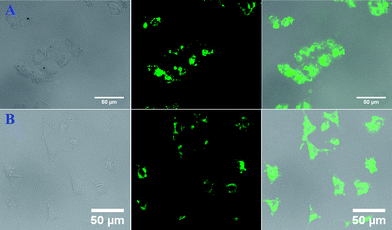 |
| Fig. 11 Differential interference contrast (left), fluorescence (center), and merge (right) images of SMMC-7721 (A) and SH-SY5Y (B) cells incubated with G-PHPMA/FITC for 6 h. Scale bar: 50 μm. | |
Conclusion
In summary, this is the first report of covalent modification of graphene with poly(N-(2-hydroxypropyl) methacrylamide) via combining RAFT polymerization and click chemistry through the “grafting-onto” strategy. The resulting polymer/modified graphene sheets (G-PHPMA) were characterized by FT-IR, Raman, element analysis, TGA, AFM, and TEM. The nanocomposite showed excellent dispersibility in common organic solvents and aqueous media. Moreover, the nanocomposite could quickly enter into SMMC-7721 and SH-SY5Y cells, which implied potential application in biomaterial area. Because of the versatility of RAFT and the efficiency of click chemistry, this thought was considered an effective and universal path to prepare polymer modified graphene composites.
Acknowledgements
The authors thank the financial supports from National Natural Science Foundation of China (21204098, 51172070, 11275050, and 51132009), Program for New Century Excellent Talents in University (NCET-10-0379), Shanghai Scientific and Technological Innovation Project (11nm0501100 and 11ZR1445900), and Shu Guang project (11SG30).
References
- A. K. Geim and K. S. Novoselov, Nat. Mater., 2007, 6, 183–191 CrossRef CAS PubMed.
- L. M. Malard and M. A. Pimenta, Phys. Rep., 2009, 473, 51–87 CrossRef CAS PubMed.
- C. Lee, X. Wei, J. W. Kysar and J. Hone, Science, 2008, 321, 385–388 CrossRef CAS PubMed.
- X. Du, I. Skachko, A. Barker and E. Y. Andrei, Nat. Nanotechnol., 2008, 3, 491–495 CrossRef CAS PubMed.
- C. X. Lim, H. Y. Hoh, P. K. Ang and K. P. Loh, Anal. Chem., 2010, 82, 7387–7393 CrossRef CAS PubMed.
- R. S. Dey and C. R. Raj, J. Phys. Chem. C, 2010, 114, 21427–21433 CAS.
- Z. Liu, J. T. Robinson, X. M. Sun and H. J. Dai, J. Am. Chem. Soc., 2008, 130, 10876–10877 CrossRef CAS PubMed.
- M. Fang, K. G. Wang, H. B. Lu, Y. L. Yang and S. Nutt, J. Mater. Chem., 2009, 19, 7098–7105 RSC.
- B. Q. Zhang, W. Ning, J. M. Zhang, X. Qiao, J. Zhang, J. S. He and C. Y. Liu, J. Mater. Chem., 2010, 20, 5401–5403 RSC.
- X. H. An, T. S. Simmons, R. Shah, C. Wolfe and K. M. Lewis, Nano Lett., 2010, 10, 4295–4301 CrossRef CAS PubMed.
- R. Hao, W. Qian, L. Zhang and Y. Hou, Chem. Commun., 2008, 6576–6578 RSC.
- K. P. Loh, Q. L. Bao, P. K. Ang and J. X. Yang, J. Mater. Chem., 2010, 20, 2277–2289 RSC.
- J. R. Lomeda, C. D. Doyle, D. V. Kosynkin, W. F. Hwang and J. M. Tour, J. Am. Chem. Soc., 2008, 130, 16201–16206 CrossRef CAS PubMed.
- T. Kuila, S. Bose, A. K. Mishra, P. Khanra, N. H. Kim and J. H. Lee, Prog. Mater. Sci., 2012, 57, 1061–1105 CrossRef CAS PubMed.
- W. D. Lee and S. S. Im, J. Polym. Sci., Part B: Polym. Phys., 2007, 45, 28–40 CrossRef CAS.
- B. D. Wang, D. Yang, J. Z. Zhang, C. B. Xi and J. H. Hu, J. Phys. Chem. C, 2011, 115, 24636–24641 CAS.
- G. J. Yu and P. Y. Wu, Polym. Chem., 2014, 5, 96–104 RSC.
- X. Sun, Z. Liu, K. Welsher, J. T. Robinson, A. Goodwin, S. Zaric and H. J. Dai, Nano Res., 2008, 1, 203–212 CrossRef CAS PubMed.
- B. Zdyrko and I. Luzinov, Macromol. Rapid Commun., 2011, 32, 859–869 CrossRef CAS PubMed.
- J. F. Shen, Y. Z. Hu, C. Li, C. Qin and M. X. Ye, Small, 2009, 5, 82–85 CrossRef CAS PubMed.
- S. Sun, Y. Cao, J. C. Feng and P. Y. Wu, J. Mater. Chem., 2010, 20, 5605–5607 RSC.
- Y. F. Yang, X. H. Song, L. Yuan, M. Li, J. C. Liu, R. Q. Ji and H. Y. Zhao, J. Polym. Sci., Part A: Polym. Chem., 2012, 50, 329–337 CrossRef CAS.
- Y. Deng, Y. J. Li, J. Dai, M. D. Lang and X. Y. Huang, J. Polym. Sci., Part A: Polym. Chem., 2011, 49, 1582–1590 CrossRef CAS.
- K. Matyjaszewski and J. H. Xia, Chem. Rev., 2001, 101, 2921–2990 CrossRef CAS PubMed.
- M. Horn and K. Matyjaszewski, Macromolecules, 2013, 46, 3350–3357 CrossRef CAS.
- E. Rizzardo, J. Chiefari, Y. K. Chong, F. Ercole, J. Krstina, J. Jeffery, T. P. T. Le, R. T. A. Mayadunne, G. F. Meijs, C. L. Moad and G. Moad, Macromol. Symp., 1999, 143, 291–307 CrossRef CAS.
- J. Chiefari, Y. K. Chong, F. Ercole, J. Krstina, J. Jeffery, T. P. T. Le, R. T. A. Mayadunne, C. L. Moad, G. Moad, E. Rizzardo and S. H. Thang, Macromolecules, 1998, 31, 5559–5562 CrossRef CAS.
- H. De-Brouwer, M. A. J. Schellekens, B. Klumperman, M. J. Monteiro and A. L. German, J. Polym. Sci., Part A: Polym. Chem., 2000, 38, 3596–3603 CrossRef CAS.
- A. Goto, K. Sato, Y. Tsujii, T. Fukuda, G. Moad, E. Rizzardo and S. H. Thang, Macromolecules, 2001, 34, 402–408 CrossRef CAS.
- H. C. Kolb, M. G. Finn and K. B. Sharpless, Angew. Chem., Int. Ed., 2001, 44, 2004–2021 CrossRef.
- C. R. Becer, R. Hoogenboom and U. S. Schubert, Angew. Chem., Int. Ed., 2009, 48, 4900–4908 CrossRef CAS PubMed.
- P. Wu, A. K. Feldman, A. K. Nugent, C. J. Hawker, A. Scheel, B. Voit, J. Pyun, J. M. J. Frechet, K. B. Sharpless and V. V. Fokin, Angew. Chem., Int. Ed., 2004, 43, 3928–3932 CrossRef CAS PubMed.
- J. T. Lai, D. Filla and R. Shea, Macromolecules, 2002, 35, 6754–6756 CrossRef CAS.
- C. Boyer, J. Q. Liu, V. Bulmus, T. P. Davis, C. Barner-Kowollik and M. H. Stenzel, Macromolecules, 2008, 41, 5641–5650 CrossRef CAS.
- K. Ulbrich, V. Subr, J. Strohalm, D. Plocova, M. Jelinkova and B. Rihova, J. Controlled Release, 2000, 64, 63–79 CrossRef CAS.
- J. Suksiriworapong, K. Sripha and V. B. S. Junyaprasert, Polymer, 2010, 51, 2286–2295 CrossRef CAS PubMed.
- J. Q. Liu, L. Tao, W. R. Yang, D. Li, C. Boyer, R. Wuhrer and T. P. Davis, Langmuir, 2010, 26, 10068–10075 CrossRef CAS PubMed.
- X. Y. Chen, L. Yuan, P. Y. Yang, J. H. Hu and D. Yang, J. Polym. Sci., Part A: Polym. Chem., 2011, 49, 4977–4986 CrossRef CAS.
- Y. Hernandez, V. Nicolosi, M. Lotya, F. M. Blighe, Z. Y. Sun, S. De, I. T. McGovern, B. Holland, M. Byrne, Y. K. Gunko, J. J. Boland, P. Niraj, G. Duesberg, S. Krishnamurthy, R. Goodhue, J. Hutchison, V. Scardaci, A. C. Ferrari and J. N. Coleman, Nat. Nanotechnol., 2008, 3, 563–568 CrossRef CAS PubMed.
- C. Peng, W. Hu, Y. Zhou, C. H. Fan and Q. Huang, Small, 2010, 6, 1686–1692 CrossRef CAS PubMed.
- V. Cauda, A. Schlossbauer, J. Kecht, A. Zurner and T. Bein, J. Am. Chem. Soc., 2009, 131, 11361–11370 CrossRef CAS PubMed.
|
This journal is © The Royal Society of Chemistry 2014 |