DOI:
10.1039/C3RA47264G
(Paper)
RSC Adv., 2014,
4, 6391-6396
Green up-conversion luminescence of Yb3+-Er3+ co-doped CaLa2ZnO5 for optically temperature sensing†
Received
3rd December 2013
, Accepted 23rd December 2013
First published on 2nd January 2014
Abstract
A class of Yb3+/Er3+ ion co-doped CaLa2ZnO5 (CLZ) novel up-conversion (UC) phosphors were synthesized by a sol–gel method. The crystal structure and the phase composition of the samples were characterized by X-ray diffraction (XRD). Under 980 nm excitation, UC spectra are composed of three prominent emission bands centered at 524, 547 and 672 nm originating from 2H11/2 → 4I15/2, 4S3/2 → 4I15/2 and 4F9/2 → 4I15/2 electronic transitions of Er3+ ion in green and red regions, respectively. The UC spectra as a function of the concentration of the dopants were also investigated to determine the optimal composition. A possible UC mechanism in Er3+/Yb3+ co-doped CLZ is proposed in light of the dependence of emission intensities on pumping power. The photoluminescence lifetimes of the green band at 547 nm and the red band at 672 nm were also measured. The temperature dependence of fluorescence intensity ratio (FIR) of the two green UC emission bands peaked at 524 and 547 nm was studied in the range of 298–513 K under an excitation of 980 nm produced by a diode laser, and the maximum sensitivity was approximately 0.0059 K−1. Results suggest that the Yb3+/Er3+ co-doped CLZ phosphor is an efficient UC phosphor and a promising candidate for optical temperature sensors.
1. Introduction
Conventional temperature sensors are based on the principle of liquid and metal expansion, in which the measurement of temperature is achieved by heat flow to an invasive probe. These contact methods cannot be applied in many situations, such as intracellular temperature, oil refineries, coal mines and corrosive environments.1–3 In recent years, noncontact temperature measurement methods involving temperature dependent fluorescence intensity and optical temperature sensing devices, based on luminescent materials with rare earth (RE) ions as the activators, have attracted great interest.4 In particular, the fluorescence lifetime and fluorescence intensity ratio (FIR) schemes have received great attention and are regarded as very promising techniques for temperature sensing. The FIR technique based on the measurement of luminescence intensities from two thermally coupled levels of RE ion is independent of spectrum losses and fluctuations in the excitation intensity, so it can provide more accurate temperature measurements.5,6 For the coupled levels, the energy separation between them must be large enough to avoid strong overlapping of the two emissions (>200 cm−1) and short enough (>2000 cm−1) to allow the upper level to have a minimum population of optically active ions in the temperature range of interest.4,7 Some RE ions can meet this requirement to a greater extent, such as Pr3+ (3P1, 3P1 → 3H5), Nd3+ (4F3/2, 4F5/2 → 4I9/2), Sm3+ (4F3/2, 4G5/2 → 6H5/2), Eu3+ (5D0, 5D1 → 7F1), Tm3+ (3F3/2, 3H4 → 3H6), Dy3+ (4F9/2, 4I15/2 → 6H13/2) and Er3+ (4S3/2, 4F9/2 → 4I15/2).8–13 Among these ions, Er3+ ion has been investigated extensively due to its green UC emissions originating from two thermally coupled levels 2H11/2 and 4S3/2; the energy gap ΔE between them is about 800 cm−1 and locates in the range of 200 cm−1 < ΔE < 2000 cm−1,5,10 which matches well with the requirement for the thermally coupled levels.
The ternary oxides XRE2ZO5 (X = Ba, RE = rare-earth, Z = Cu, Zn) have also been receiving much attention because of their very interesting structural, physical and chemical properties and additionally they exhibit special magnetic, optical and superconducting properties.14–16 According to these studies, CaLa2ZnO5 is iso-structural with BaLa2ZnO5.17 Rare earth ions co-doped BaLn2ZnO5 (Ln = La, Gd, Y) have been proved to be efficient UC hosts, it is expected that CaLa2ZnO5 could be a suitable UC host.18,19 So far, only down-conversion luminescence of trivalent RE ion doped CaLa2ZnO5 has been investigated and no investigations on infrared-to-visible UC luminescence properties of rare earth doped CaLa2ZnO5 have been reported.17 In order to improve their efficiencies and absorptions at 980 nm, Yb3+ is often used as an efficient sensitizer in UC system since it possesses only one excited f-electron level that allows a strong and broad absorption band around 980 nm.20 As for as synthesis of phosphors is concerned, sol–gel method has been commonly used due to it requires lower sintering temperature and shorter heating time, and provides regular particle shape and narrow particle size distribution,21 which favor an the enhancement of photoluminescence intensity.
In the current study, the UC phosphors CaLa2ZnO5: Er3+-Yb3+ were prepared by sol–gel method and their UC luminescence behavior was investigated. The possible mechanisms are elucidated through power dependence and lifetime measurements. The temperature sensing behavior by using the FIR technique on the synthesized phosphor has also been discussed in detail under a 980 nm laser diode excitation.
2. Experimental
2.1. Sample preparation
CaLa2−x−yZnO5: x Yb3+, y Er3+: (a) y = 0.02; x = 0, 0.05, 0.1, 0.15, 0.25, and (b) x = 0.05; y = 0.005, 0.01, 0.02, 0.03, 0.05 phosphors were prepared by using a modified sol–gel method. Typically in sol–gel method alkoxides are used as raw materials, which are often relatively expensive and may be toxic.22 In our experiments, rare earth oxides with high purity Ln2O3 (Ln = La, Yb, Er; 99.99%, Shanghai Yuelong Nonferrous Metals Ltd.), analytical grade CaCO3 and ZnO were used as raw materials. First, stoichiometric amounts of rare earth oxides, CaCO3 and ZnO were dissolved in dilute HNO3 (analytical grade, AR) under vigorous stirring, and the excess nitric acid was removed at high temperature. Then suitable amount of distilled water was added with constant stirring to form a homogeneous solution. Subsequently, the calculated quantity of citric acid (AR) is added to this solution, which acts as chelating agent of the metal ions. The molar ratio of the total chelated metal cations to citric acid was 1
:
2. A transparent aqueous solution was obtained after stirring for a few minutes. The obtained transparent aqueous solution was kept in an oven at 80 °C for 24 h to get colorless transparent resin, and the resin was then dried at 120 °C for 24 h to get yellowish dried gel. The dried gel was ground and preheated in air atmosphere at 500 °C for 2 h, and then sintered at required temperature at 1000 °C for 5 h to obtain final samples for testing.
2.2. Characterization
X-ray powder diffractions (XRD) patterns of the samples were obtained using a Rigaku-Dmax 3C powder diffractometer (Rigaku Corp, Tokyo, Japan) with Cu-Kα radiation (λ = 1.5406 Å). UC luminescence spectra of the phosphors at room temperature (RT) were recorded using a Hitachi F-4600 spectrophotometer (Hitachi high technologies corporation, Tokyo, Japan) with an external power-controllable 980 nm semiconductor laser (Hi-Tech Optoelectronics Company, Beijing, China) as excitation source. The lifetimes for 4S3/2 → 4I15/2 and 4F9/2 → 4I15/2 transitions of Er3+ at 547 and 672 nm at RT were carried out with a 980 nm pulsed laser of an optical parametric oscillator (OPO) as excitation source, and the signals were detected by a Tektronix digital oscilloscope (TDS 3052). To investigate the temperature dependence of the UC emission, the sample was placed in a temperature-controlled copper cylinder, and its temperature was increased from RT to 240 °C. The UC spectra of sample at various temperatures were obtained using a Jobin-Yvon HRD-1 double monochromator equipped with a Hamamatsu R928 Photomultiplier under the excitation of a 980 nm diode laser with 30 mW. The signal was analyzed by an EG&G 7265 DSP Lock-in Amplifier and stored into computer memories.
3. Results and discussion
3.1. Phase composition and crystal structure
The phase composition and crystal structure of all samples were identified by X-ray powder diffraction analysis. Fig. 1 shows the XRD patterns of CaLa1.93Yb0.05Er0.02ZnO5 phosphors as a function of sintering temperatures in the range of 800–1200 °C for 5 h. In addition, the XRD curve of blank CLZ annealed at 1100 °C for 5 h was also displayed in this figure, which is in agreement with what reported in ref. 17 and there was no diffraction peaks from any secondary phase observed. In case of the samples sintered at 800 °C, most diffraction peaks in XRD pattern could be indexed to CLZ, but there are a few weak diffraction peaks attributed to unidentified secondary phase(s). With further increase of the sintering temperature, the diffraction peaks from secondary phases disappeared completely in the range 900–1200 °C, which indicates that the ions Yb3+ and Er3+ are incorporated in the host lattice and do not alter the host structure significantly.
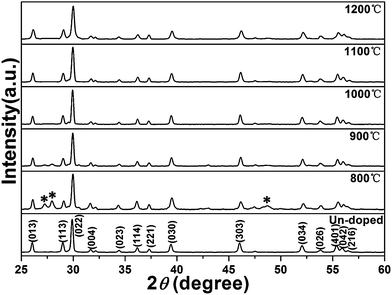 |
| Fig. 1 XRD patterns of CaLa2ZnO5:Yb3+, Er3+ phosphors sintered at different temperature and the un-doped CLZ at 1100 °C. | |
3.2. Dependence of UC spectra on dopant contents
The UC emission spectra for all prepared samples CaLa2−x−yZnO5: x Yb3+, yEr3+ were measured at sintered temperature for 1000 °C due to its highest UC intensity (in Fig. S1 of the ESI†), and results indicate that the UC emission intensity and spectral properties depend on dopant concentrations. UC spectra of the Er3+/Yb3+ co-doped CLZ consist of strong green emission peaks at 524 and 547 nm and a weak red emission peaked at 672 nm, which are assigned to 2H11/2 → 4I15/2, 4S3/2 → 4I15/2 and 4F9/2 → 4I15/2 transitions of Er3+, respectively. Fig. 2 presents the room temperature UC spectra of the selected Yb3+/Er3+ co-doped CaLa2ZnO5 families (a) with invariable Er3+ contents y = 0.02 and variable Yb3+ concentrations ranging from 0 to 0.25 and (b) with Er3+ concentrations y ranging from 0.005 to 0.05 and fixed Yb3+ content x at 0.05 under excitation at 980 nm. The normalized integrated intensities for green and red UC emissions were also calculated and displayed in the insets of Fig. 2(a) and (b) as function of Yb3+ and Er3+ concentrations for the two family samples, respectively. From inset of Fig. 2(a), it can be found that the UC emission intensities increase first and then decreases after reaching an optimum performance at around x = 0.05 with the increase of Yb3+ concentration. UC intensity in green and red region become stronger with the introduction of sensitizer Yb3+ in comparison with that of Er3+ singly doped CaLa2ZnO5. This is attributed to the more populated green and red emitting levels of Er3+, which due to an efficient energy transfer (ET) from Yb3+ to Er3+ for the larger absorption cross section of Yb3+ at 980 nm and larger energy overlap between Yb3+ and Er3+. As the Yb3+ concentration is increased above 0.05 at constant Er3+ concentration, the UC becomes less efficient, which may be mainly caused by the energy back transfer (EBT) Er3+ (4S3/2) + Yb3+ (2F7/2) → Er3+ (4I13/2) + Yb3+ (2F5/2).23,24 The higher Yb3+ concentration, the higher is the ratio between EBT from Er3+ to Yb3+ and energy transfer from Yb3+ to Er3+.25 For constant Yb3+ concentration at x = 0.05, similar trend was found and the optimum Er3+ concentration is about 0.02, as shown in the inset of Fig. 2(b). The UC intensity decreases with the concentration of Er3+ beyond 0.02, which may be due to the concentration quenching effect. The concentration quenching effect could be explained by the energy transfer between the nearest Er3+ and Yb3+/Er3+ ions. With increasing the contents of Er3+, the distance between Er3+ and Er3+/Yb3+ ions will decrease, which promotes non-radiative energy transfer and leads to the decrease of UC emission intensity.26 The digital photograph in the inset of Fig. 2 displays the brightest green light for the sample annealed at 1000 °C dispersing in alcohol under the excitation of 980 nm laser.
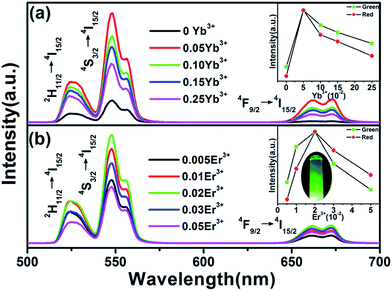 |
| Fig. 2 UC luminescence spectra of CaLa2−x−yZnO5: x Yb3+, y Er3+: (a) y = 0.02; x = 0, 0.05, 0.1, 0.15, 0.25, and (b) x = 0.05; y = 0.005, 0.01, 0.02, 0.03, 0.05. Insets show the normalized integrated intensities of the green and red emissions as a function of Er3+ and Yb3+ concentrations. | |
3.3. Mechanism of UC emission
For UC process, the intensity of the UC luminescence (I) is proportional to the nth power of the pump laser powder (P), i.e., I ∝ Pn, where n denotes the number of pumping photons absorbed per up-converted photon emitted.27 To determine the number of photons responsible for the UC mechanism of Yb3+/Er3+ co-doped CaLa2ZnO5, the dependences of the green (547 nm) and red (672 nm) emissions under 980 nm excitation of CaLa1.93Yb0.05Er0.02ZnO5 phosphors upon the pump power were investigated at room temperature. As shown in Fig. 3(a), the slopes of the both curves are 2.26 for green (4S3/2 → 4I15/2) and 2.30 for red (4F9/2 → 4I15/2), respectively, which are much larger than 2 and reveals that two and three IR photons are involved to produce one green or red in this UC process.28
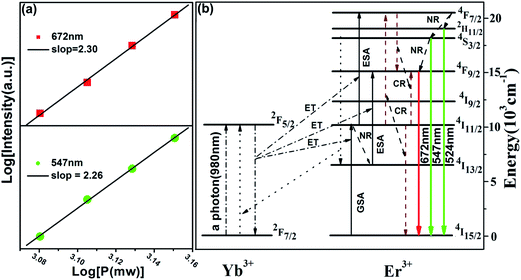 |
| Fig. 3 (a) Dependence of UC luminescence intensity of CaLa1.93Yb0.05Er0.02ZnO5 upon the pumping power; and (b) energy level diagram of the Yb3+, Er3+ ions and the proposed UC mechanisms in Er3+/Yb3+ co-doped CaLa2ZnO5 under 980 nm excitation. | |
To better comprehend the UC mechanism that populates the emitting state and radiative transitions of green and red UC luminescence, the possible mechanism and population processes in CaLa2ZnO5: Yb3+/Er3+ phosphor are schematically illuminated in Fig. 3(b) by the energy levels diagram and the energy matching. Under the 980 nm excitation, the population at the 4I11/2 level in Er3+ could be realized through the ground state absorption (GSA) of Er3+ or energy transfer (ET) from the 2F5/2 state of Yb3+ to Er3+ process 4I15/2 (Er3+) + 2F5/2 (Yb3+) → 4I11/2 (Er3+) + 2F7/2 (Yb3+). For above mentioned two processes, the energy transfer plays a dominant role because the larger absorption cross section of Yb3+ and the resonance between Yb3+ (2F5/2 → 2F7/2) and Er3+ (4I15/2 → 4I11/2) transition. For the green emissions those arise from the 2H11/2, 4S3/2 → 4I15/2 transitions, there are three possible processes for the ET, namely 4I11/2 to the 4F7/2 level of Er3+ as follows: Er3+ (4I11/2) + a photon (980 nm) → Er3+ (4F7/2) (excited state absorption: ESA), Er3+ (4I11/2) + Yb3+ (2F5/2) → Er3+ (4F7/2) + Yb3+ (2F7/2) (ET) and Er3+ (4I11/2) + Er3+ (4I11/2) → Er3+ (4F7/2) + Er3+ (4I15/2) (cross relaxation: CR).26,29 Followed by non-radiative relaxation (NR) from 4F7/2 level to the meta-stable 2H11/2 and 4S3/2 levels, the green emissions (524 and 547 nm) of 2H11/2 (4S3/2) → 4I15/2 occur. For the red emission resulting from 4F9/2 → 4I15/2 transition, the 4F9/2 level can be populated by further NR from 4S3/2, energy transfer from an excited Yb3+ to 4I13/2 state that from the NR of Er3+ ion in the 4I11/2 intermediate state, and the CR process 4F7/2 + 4I11/2 → 4F9/2 + 4F9/2 transition of Er3+. Finally, the 4F9/2 level relaxes radiatively to the ground state (4I15/2 level) of Er3+ with a red emission at 672 nm.
3.4. Lifetime measurements
In order to further investigate the influence of ET between Yb3+ and Er3+ on the UC luminescence, the emission decay curves of the green (4S3/2 → 4I15/2, 547 nm) and red (4F9/2 → 4I15/2, 672 nm) for the samples CaLa1.98−xZnO5: x Yb3+, 0.02 Er3+ (x = 0, 0.05, 0.10.) were recorded after 980 nm pulsed laser excitation. Fig. 4(a) and (b) shows the normalized decay profiles of the 4S3/2 → 4I15/2 (green) and 4F9/2 → 4I15/2 (red) transitions, respectively. The effective lifetimes are defined in equation:30 |
 | (1) |
here I(t) represents the luminescence intensity at time t. The decay curves can be well fitted with double exponential equation. And the average lifetime from decay curves can be calculated using the following equation:31 |
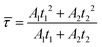 | (2) |
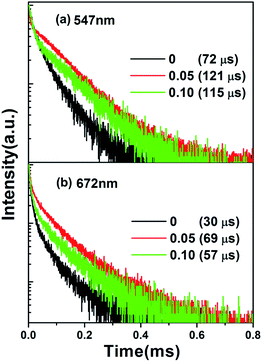 |
| Fig. 4 The temporal evolution of the green 4S3/2 → 4I15/2 (a) and red 4F9/2 → 4I15/2 (b) emissions in CaLa1.98−xZnO5: x Yb3+, 0.02 Er3+ (x = 0, 0.05, 0.10.). | |
The corresponding lifetimes are listed in Fig. 4, respectively. It is found that the lifetimes of green and red have been prolonged after the Yb3+ co-doping and the similar trend of the enhancement of UC luminescence intensity was confirmed, which confirms the presence of ET from Yb3+ to Er3+ in present system. Usually, the excited state is depopulated by the radiative and non-radiative transition processes, and the non-radiative processes include phonon-assisted non-radiative process and energy transfer process. With the increase of Yb3+ concentration, closer ionic distance could be obtained and more efficient energy transfer from Yb3+ to Er3+ could be expected and results in the green level lifetime reaching the maximum 121 μs at 0.05. However, the green emission lifetime decreases as the Yb3+ concentration is over 0.05 due to the energy back transfer (EBT) from Er3+ to Yb3+: Yb3+ (2F7/2) + Er3+ (4S3/2) → Yb3+ (2F5/2) + Er3+ (4I13/2) also become convenient in much higher Yb3+ concentration, which accelerates the depopulation of 4S3/2 level and results in the reduction of the green emission lifetime. As shown in Fig. 5(b), the variation trend of the red emission lifetime agrees with that of the green emission lifetime, and the red emitting level lifetime decreases from 69 to 57 μs with the increase of Yb3+ from 0.05 to 0.1. For the red emission, the 4F9/2 level from the further NR from 4S3/2 relaxes radiatively to the ground state (4I15/2) level. However, the depopulation of 4S3/2 level due to the energy back transfer (EBT) from Er3+ to Yb3+, resulting in the red emission lifetime decreases with the increase of Yb3+ concentration.
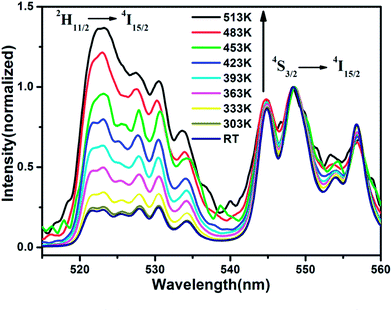 |
| Fig. 5 Temperature dependence of the green UC luminescence spectra of CaLa2ZnO: 0.05 Yb3+, 0.02 Er3+ under 980 nm excitation (the spectra are normalized to the most intense emission peak at 548 nm). | |
3.5. Temperature sensing behavior
Because of the small energy gap (≈750 cm−1) between the levels 2H11/2 and 4S3/2 of Er3+, the state of 2H11/2 may also be populated from 4S3/2 by thermal excitation and a quasithermal equilibrium occurs between the two coupled levels. The fluorescence intensity ratio (FIR) of the UC emission from 2H11/2 → 4I15/2 (524 nm) and 4S3/2 → 4I15/2 (548 nm) transitions of Er3+ could change with variation of external temperature. To investigate the temperature sensing behaviour of synthesized phosphors, the green UC emission spectra of CaLa2ZnO5: 0.05 Yb3+, 0.02 Er3+ under various temperatures from room temperature (RT) 293 to 513 K were shown in Fig. 5 using an unfocused laser beam of typically 30 mW at 980 nm, in which the spectra are normalized to the most intense emission peak at 548 nm. In the present case, the low exciting power was utilized (30 mW), which is in favor of the reduction of the heating effect caused by the excitation. It is found that the positions of the two UC emission bands in green region barely change with the increase of temperature, whereas the FIR of the two emission bands obviously varies with temperature. The relative population of the two thermally coupled multiplets follows the Boltzmann distribution. With thermalization of populations at the 2H11/2 and 4S3/2 levels, the FIR of green UC emissions from 2H11/2 and 4S3/2 to ground state 4I15/2 transitions can be written as:3,32 |
 | (3) |
where IH and IS are the integrated intensities of 2H11/2 → 4I15/2 (integrated from 515 to 540 nm) and 4S3/2 → 4I15/2 (integrated from 540 to 562 nm) transitions, respectively. N, g, σ, ω are the number of ions, the degeneracy, the emission cross-section, the angular frequency of fluorescence transitions from the 2H11/2 and 4S3/2 levels to the 4I15/2 level, respectively. ΔE is the energy gap between the 2H11/2 and 4S3/2 levels, K is the Boltzmann constant, T is absolute temperature, and the preexponential constant is given by C = gHσHωH/gSσSωS.
The monolog plot of the FIR of green UC emissions for the 2H11/2, 4S3/2 → 4I15/2 transitions as a function of inverse absolute temperature in the range of 298–513 K is shown in Fig. S2 of the ESI.† The experimental data could be fitted by straight line with a slop of about 1068.8. The dependence of FIR of green UC emissions at 524 and 547 nm on the absolute temperature was shown in Fig. 6(a). The FIR increased from 0.32 to 1.40 with increasing the temperature from room temperature 298 to 513 K. The coefficient C value in eqn (3) is 11.3 according to fitting curve of the experimental data. And the effective energy difference obtained by the fitting is 775 cm−1, which is close to the well-known splitting of 700–800 cm−1 between 2H11/2 and 4S3/2 multiplets. For sensing application, the relative sensitivity S is a very important parameter, which has been calculated using the formula:6
|
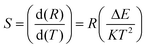 | (4) |
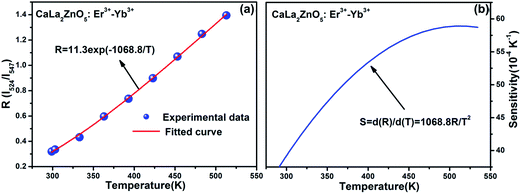 |
| Fig. 6 (a) Dependence of FIR of green UC emissions for the 2H11/2, 4S3/2 → 4I15/2 transitions on the absolute temperature and (b) sensitivity as a function of the temperature range of 298–513 K. | |
The corresponding resultant curve is shown in Fig. 6(b) as a function of the temperature. At the temperature of 483 K, the sensitivity of Er3+-Yb3+ co-doped CLZ reached its maximal value of about 0.0059 K−1. Moreover, in comparison with the other Er3+-Yb3+ co-doped oxide UC materials for temperature sensing,12,33–35 the temperature sensitivity have been improved. Hence, the present phosphor can be used as an excellent temperature sensor with high sensitivity.
4. Conclusions
A novel class of up-conversion phosphors CaLa2ZnO5 (CLZ) co-doped with Yb3+/Er3+ ions were synthesized by a sol–gel method. The UC intensity depends on the dopant concentrations, and the optimal UC emission was obtained in CLZ: 0.02 Er3+, 0.05 Yb3+ at 1000 °C. Under 980 nm excitation, the phosphors exhibit efficient visible UC emissions including strong green emission centered at 524
547 nm and weak red emission centered at around 672 nm, which were assigned to the 2H11/2, 4S3/2 → 4I15/2 and 4F9/2 → 4I15/2 transitions of Er3+, respectively. Two-photon processes are found to be responsible for these up-conversion emissions, and the possible UC mechanism is also proposed. The photoluminescence lifetime of green (4S3/2 → 4I15/2) and red (4F9/2 → 4I15/2) were also measured after 980 nm pulsed laser excitation, which confirms the existence of energy transfer. The optical thermometry in the temperature range of 298–513 K was presented using an FIR technique of the two green UC emissions in the Er3+-Yb3+: CaLa2ZnO5 and the high sensor sensitivity is around 0.0059 K−1 at 483 K. The results propose that the present phosphor material may be a good candidate for temperature sensors with high sensitivity.
Acknowledgements
This work was supported by the high-level talent project of Northwest University, National Natural Science Foundation of China (no. 11274251, 11274299), Natural Science Foundation of Hubei Province (2010CDB01607), Technology Foundation for Selected Overseas Chinese Scholar, Ministry of Personnel of China (excellent), Foundation of Shaanxi Educational Committee (11JK0528), Foundation of Key Laboratory of Photoelectric Technology in Shaanxi Province (12JS094), the Open Foundation of Key Laboratory of Photoelectric Technology and Functional Materials (Culture Base) in Shaanxi Province (ZS11010, ZS1202).
References
- P. R. N. Childs, J. R. Greenwood and C. A. Long, Rev. Sci. Instrum., 2000, 71, 2959–2978 CrossRef CAS PubMed.
- C. D. S. Brites, P. P. Lima, N. J. O. Silva, A. Mallán, V. Amaral, F. Palacio and L. d. Carlos, New J. Chem., 2011, 35, 1177–1183 RSC.
- S. S. Zhou, K. M. Deng, X. T. Wei, G. C. Jiang, C. K. Duan, Y. H. Chen and M. Yin, Opt. Commun., 2013, 291, 138–142 CrossRef CAS PubMed.
- W. Xu, Z. G. Zhang and W. W. Cao, Opt. Lett., 2012, 37, 4865–4867 CrossRef CAS PubMed.
- X. Wang, X. G. Kong, Y. Yu, Y. J. Sun and H. Zhang, J. Phys. Chem. C, 2007, 111, 15119–15124 CAS.
- V. K. Rai, A. Pandey and R. Dey, J. Appl. Phys., 2013, 113, 083104 CrossRef PubMed.
- S. F. León-Luis, U. R. Rodríguez-Mendoz, E. Lalla and E. L. Víctor Lavín, Sens. Actuators, B, 2011, 158, 208–213 CrossRef PubMed.
- V. K. Rai, D. K. Rai and S. B. Rai, Sens. Actuators, A, 2006, 128, 14–17 CrossRef CAS PubMed.
- V. K. Rai and S. B. Rai, Appl. Phys. B, 2007, 87, 323–325 CrossRef CAS.
- S. A. Wade, S. F. Collins and G. W. Baxter, J. Appl. Phys., 2003, 94, 4743–4756 CrossRef CAS PubMed.
- W. Xu, X. Y. Gao, L. J. Zheng, Z. G. Zhang and W. W. Cao, Sens. Actuators, B, 2012, 173, 250–253 CrossRef CAS PubMed.
- N. Rakov and G. S. Maciel, Sens. Actuators, B, 2012, 164, 96–100 CrossRef CAS PubMed.
- M. A. R. C. Alencar, G. S. Maciel, C. B. de Araújo and A. Patra, Appl. Phys. Lett., 2004, 84, 4753–4755 CrossRef CAS PubMed.
- C. Michel and B. Raveau, J. Solid State Chem., 1983, 49, 150–156 CrossRef CAS.
- C. F. Guo, X. Ding and Y. Xu, J. Am. Ceram. Soc., 2010, 93, 1708–1713 CrossRef CAS.
- C. F. Guo, J. Yu, J. H. Jeong, Z. Y. Ren and J. T. Bai, Phys. B, 2011, 406, 916–920 CrossRef CAS PubMed.
- V. R. Bandi, B. k. Grandhe, K. W. Jang, H. S. Lee, D. S. Shin, S. S. Yi and J. H. Jeong, J. Alloys Compd., 2012, 512, 264–269 CrossRef CAS PubMed.
- I. Etchart, I. Hernández, A. Huignard, M. Bérard, W. P. Gillin, R. J. Curry and A. K. Cheetham, J. Mater. Chem., 2011, 21, 1387–1394 RSC.
- I. Etchart, M. Bérard, M. Laroche, A. Huignard, I. Hernández, W. P. Gillin, R. J. Curry and A. K. Cheetham, Chem. Commun., 2011, 47, 6263–6265 RSC.
- K. Teshima, S. H. Lee, N. Shikine, T. Wakabayashi, K. Yubuta, T. Shishido and S. Oishi, Cryst. Growth Des., 2011, 11, 995–999 CAS.
- T. Li, C. F. Guo, Y. R. Wu and J. H. Jeong, J. Alloys Compd., 2012, 540, 107–112 CrossRef CAS PubMed.
- J. Lin, M. Yu, C. K. Lin and X. M. Liu, J. Phys. Chem. C, 2007, 111, 5835–5845 CAS.
- I. Etchart, A. Huignard, M. Bérard, M. N. Nordin, I. Hernández, R. J. Curry, W. P. Gillin and A. K. Cheetham, J. Mater. Chem., 2010, 20, 3989–3994 RSC.
- G. Y. Chen, G. Somesfalean, Y. Liu, Z. G. Zhang, Q. Sun and F. P. Wang, Phys. Rev. B: Condens. Matter Mater. Phys., 2007, 75, 195204 CrossRef.
- Y. F. Jiang, R. S. Shen, X. P. Li, J. S. Zhang, H. Zhong, Y. Tian, J. S. Sun, L. H. Cheng, H. Y. Zhong and B. J. Chen, Ceram. Int., 2012, 38, 5045–5051 CrossRef CAS PubMed.
- J. H. Chung, J. H. Ryu, J. W. Eun, J. H. Lee, S. Y. Lee, T. H. Hes, B. G. Choi and K. B. Shim, J. Alloys Compd., 2012, 522, 30–34 CrossRef CAS PubMed.
- J. H. Boyer, F. Vetrone, L. A. Cuccia and J. A. Capobianco, J. Am. Chem. Soc., 2006, 128, 7444–7445 CrossRef CAS PubMed.
- T. Li, C. F. Guo and L. Li, Opt. Express, 2013, 21, 18281–18289 CrossRef CAS PubMed.
- L. Li, C. F. Guo, Y. Q. Chen, T. Li and J. H. Jeong, Mater. Lett., 2013, 95, 52–54 CrossRef CAS PubMed.
- H. Jing, C. F. Guo, G. G. Zhang, X. Y. Su, Z. Yang and J. H. Jeong, J. Mater. Chem., 2012, 22, 13612–13618 RSC.
- T. Fujii, K. Kodaira, O. Kawauchi, N. Tanaka, H. Yamashita and M. Anpo, J. Phys. Chem. B, 1997, 101, 10631–10637 CrossRef CAS.
- B. S. Cao, Y. Y. He, Z. Q. Feng, Y. S. Li and B. Dong, Sens. Actuators, B, 2011, 159, 8–11 CrossRef CAS PubMed.
- S. K. Singh, K. Kumar and S. B. Rai, Sens. Actuators, A, 2009, 149, 16–20 CrossRef CAS PubMed.
- B. Dong, D. P. Liu, X. J. Wang, T. Yang and S. M. Miao, Appl. Phys. Lett., 2007, 90, 181117 CrossRef PubMed.
- B. Dong, B. S. Cao, Y. Y. Zhuang, Z. Liu, Z. P. Li and Z. Q. Feng, Adv. Mater., 2012, 24, 1987–1993 CrossRef CAS PubMed.
Footnote |
† Electronic supplementary information (ESI) available. See DOI: 10.1039/c3ra47264g |
|
This journal is © The Royal Society of Chemistry 2014 |