DOI:
10.1039/C3RA46898D
(Paper)
RSC Adv., 2014,
4, 10123-10132
CuO nanoellipsoids for superior physicochemical response of biodegradable PVA
Received
21st November 2013
, Accepted 12th December 2013
First published on 18th December 2013
Abstract
PVA nanocomposites comprising of nanoparticles of CuO were prepared and their various physicochemical properties were evaluated. CuO with its impressive array of mechanical, optical, dielectric and hydrophilic properties as well as environmental friendliness is expected to augment the properties of the PVA matrix. The strong interaction between the polymer matrix and the nanofillers was confirmed via XRD and FT-IR investigations. The thermal stability and the mechanical strength of the polymer membranes indeed showed notable improvements in the composites. Thermal conductivity also increased to an extent beyond the theoretical prediction. The dielectric constant of the nanocomposite film was about 2 times higher than that of the pure PVA membrane. The high optical transparency of the PVA membrane also was not compromised with the inclusion of nanosized CuO particles.
1. Introduction
Given the rapid expansion of the polymer industry, green polymers or biodegradable polymers with balanced properties for industrial applications are a matter of much interest amongst material scientists and engineers.1–3 Although most of these biodegradable polymers have excellent properties comparable to many petroleum-based plastics and are potentially excellent alternatives for commercial commodity plastics, low mechanical strength, thermal stability etc. continue to be bottlenecks towards their commercialization. To counter this critical challenge researchers have been contemplating the idea of designing composite materials of polymers incorporating nanoscale objects to realize the transfer of some of the exceptional properties of the nanoparticles to the bulk polymer matrix.4,5
Poly vinyl alcohol (PVA) is a semi-crystalline polymer that is water soluble and completely biodegradable, and has attractive traits such as hydrophilicity, chemical resistance, emulsifying, adhesivity and excellent film forming capability.6,7 It is, currently the largest volume synthetic resin produced in the world. The number of existing and potential applications of PVA in fields such as biomedical applications,8 drug delivery,9 bioseparation, carriers for cell immobilization,10 barrier films for food and biomedical packaging11 explain why the properties of PVA are being widely examined across the research fraternity all over the world.
Besides, the high optical transparency of PVA enables it to be used in optically clear soft contact lenses.12 To further improve the performance level of PVA regarding these applications, its properties such as thermal stability, moisture resistance and mechanical strength are being tuned by introducing nanosized fillers within the polymer matrix. Carbon derivative materials such as graphene, carbon nanotubes (CNTs) and diamond7,13–19, minerals like clay and macromolecules such as cellulose20,21 have all had varying degrees of success in composite materials. The carbon derivatives, especially the nanotubes, have a tendency to agglomerate which can impede their potential influence on the polymer’s properties to some extent.
But given how good a host material PVA can be for metal22,23 or semiconductor materials,24,25 it is a great surprise that so few literature reports exist on their exploitation as filler nanomaterials. Especially, semiconductor metal oxides with their wide range of excellent properties have the potential to be suitable nanofillers if explored.26–31 Besides, the oxide materials have the possibility to strike a strong host–filler relationship with the hydroxy-functionalized PVA chains via hydrogen bonding. The basic aim is to improve some of the properties without compromising the polymers’ other advantageous properties.
In this article, we report the utilization of CuO nanoparticles to fabricate PVA nanocomposite materials with augmented thermal and mechanical stability and modified thermal conductivity and optical property. The reason behind selecting CuO as a filler material lies in its inexpensive and simple synthesis procedures, environmental benignity and impressive optical and mechanical properties.32,33 Additionally, the hydrophilic nature of CuO should enable a facile dispersion within the hydrophilic PVA matrix in an aqueous medium leading to strong interaction between them.34
Recently, scientists have shown keen interest in studying the electrical properties of PVA with its potential suitability as a dielectric material in microchips, suspension insulators, components of transformers, switch boards etc. and a good number of articles have been published focussing on the dielectric behaviour of PVA and its composites.28,35,36 On the other hand CuO nanoparticles have been reported to behave as a giant dielectric material.37,38 Taking the above facts into consideration we have also explored the influence of CuO nanoparticles on the dielectric properties of PVA.
2. Experimental
2.1 Materials
Cu(NO3)2·3H2O (cupric nitrate trihydrate, 99.5%, Alfa Aesar) and NaOH (sodium hydroxide) were of analytical grade and were used without any further purification. Poly vinyl alcohol (PVA, Mw ≈ 125
000) was purchased from CDH Ltd.
2.2 Synthesis of CuO nanoparticles
CuO nanoparticles were prepared via a simple wet chemical solution method. In this method 1 wt% aqueous solution of copper nitrate was treated with 1 M NaOH solution until the pH of the solution reached 10.0 and a dense blue-green precipitate of Cu(OH)2 appeared. This precipitate was calcined at a temperature of 130 °C for the duration of 10 hours to obtain black CuO powder. Details of the synthesis procedure and related growth mechanism, and the effect of pH have been discussed in a previous publication.31
2.3 Casting of the nanocomposite films
The CuO–PVA nanocomposite films were prepared via a simple solution casting method. 5 g of PVA was dissolved in 50 ml of distilled water under constant stirring at room temperature.
The requisite amount of CuO nanoparticles was added to 10 ml of distilled water followed by ultrasonication for 10 minutes to prepare uniform aqueous dispersions. The amount of CuO was chosen according to the aimed weight ratio of the nanocomposite components. Next, the aqueous dispersion was added dropwise to the PVA solution under vigorous stirring to obtain a uniform distribution of the nanoparticles in the polymer matrix. This solution was spread uniformly on glass plates and kept inside a micro-oven for 12 hours maintaining a temperature of 55 °C. The resultant films were peeled off and used for further property evaluations. In the current work, we prepared 3 different nanocomposite films with one being pure PVA film (S0) and the other two maintaining a PVA to CuO weight ratio of 500
:
1 (S1) and 50
:
1 (S2).
2.4 Characterization methods
The crystallographic characteristics of the samples were identified through X-ray diffraction (XRD) via a Rigaku benchtop X-ray diffractometer using monochromatized Cu-Kα radiation (λ = 1.54059 Å). The morphological investigations of the sample were carried out through scanning electron microscopy (SEM) images recorded on a Zeiss EVO MA-10 scanning electron microscope. Microstructural characterization at high magnifications was performed using STEM (Scanning Transmission Electron Microscopy) attached to a Field Emission Scanning Electron Microscope (FESEM, model-Zeiss Supra 40VP) and high resolution transmission electron microscopy (HR-TEM: FEI Tecnai G2 F30 STWIN at 300 keV). Thermal transitions and stability were probed applying by differential scanning calorimetry (DSC) and thermogravimetric analysis (TGA) through a Mettler Toledo TGA/DSC 1 high temperature (HT) Star system. The thermal conductivity of the samples was recorded using a hot disc thermal constant analyzer (TPS-500). The corresponding experimental error associated is ±2%. The UV-Vis spectra of the samples were recorded by a JASCO UV-VIS/NIR Spectrophotometer (model V-670) and the luminescence characteristics were investigated by photoluminescence spectroscopy using a Perkin-Elmer LS-55 luminescence spectrophotometer (Xe source). Dielectric property data of the samples were investigated using an iImpedance analyzer Wayne Kerr (6540A), UK within the frequency range 20–100 MHz at room temperature.
The mechanical properties of the films were evaluated using an Instron 4411 Universal testing machine under tensile measurements. The grip distance and the cross head speed were maintained respectively at 15 mm and 50 mm min−1. The samples were designed as rectangular stripes (60 mm × 10 mm) and the thickness of the three samples was measured to be 0.36 mm (S0), 0.50 mm (S1) and 0.68 mm (S2).
3. Results and discussions
3.1 Enhancement of polymer crystallinity
Fig. 1 exhibits the X-ray diffraction patterns of the pure PVA and the nanocomposite samples. The inset provides the XRD pattern for the synthesized CuO nanoparticles. The position of the diffraction peaks and the relative intensity of the peaks agree perfectly with the standard JCPDF card no. 05-0661, corresponding to the crystal system of monoclinic CuO (space group: C2/c; a: 0.4684 nm, b: 0.3425 nm, c: 0.5129 nm; β = 99.47°).
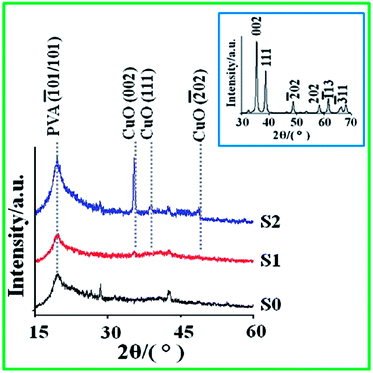 |
| Fig. 1 XRD patterns of the pure PVA membrane (S0) and the CuO–PVA nanocomposite membranes (S1 and S2). Increased intensity of the PVA crystalline peak in the nanocomposites is visibly apparent. Inset provides the XRD pattern for the CuO nanoparticles. | |
The X-ray diffraction profiles of pure and CuO nanoparticle incorporated PVA films, are all characterized by an intense peak at ∼19.5° which is typical of crystalline PVA. The small peaks observed at ∼28° and ∼41° for S0 and S1 are similar to the halos observed in the diffraction profile of pure water.6 Diffraction patterns for the composite films (S1 and S2) consist of peaks corresponding to CuO and these peaks gain in intensity from S1 to S2, which is in sync with the respective CuO nanofiller concentration of the two samples. More significantly, the corresponding diffraction peak for PVA also becomes more intense in the XRD profiles of the composites compared to the pure PVA sample, suggesting an increase in the crystallinity of the polymer. The calculated percent crystallinity of PVA from the XRD pattern was 32.3% for S0 and 35.2% and 41.5% for S1 and S2 respectively.
3.2 Strong interaction between the nanoparticles and the polymer chain
The increase in crystallinity of the polymer suggests strong chemical interactions between the CuO nanofillers and the PVA functional groups. This phenomenon can be corroborated with the FT-IR spectroscopic analysis of the samples.
Fig. 2a shows the vibrational spectra of the PVA membrane and its CuO incorporated composites in the IR region. The bands observed between 3000 and 3600 cm−1 are characteristic of the –OH functional groups present in PVA. The band at 2915 cm−1 corresponds to the stretching of –CH2– present in the PVA chain. The bands at 1380 and 1265 cm−1 occur due to the vibrational motion of the C–H groups, whereas, the band at 842 cm−1 arises due to the C–C stretching motion. Two other oxygen containing functional groups were detected at 1715 cm−1 (C
O functional groups from aliphatic acids or aldehydes) and at 1094 cm−1 due to the stretching mode of C–O bonds. The band at the right corner of the spectra at 530 cm−1 observed for S1 and S2, can be assigned to the Cu–O vibrational motion.31 The FT-IR troughs appear to be particularly intense in the composite S2 indicating that the insertion of CuO nanoparticles had significant effect on the bonding interactions within the PVA structural framework.
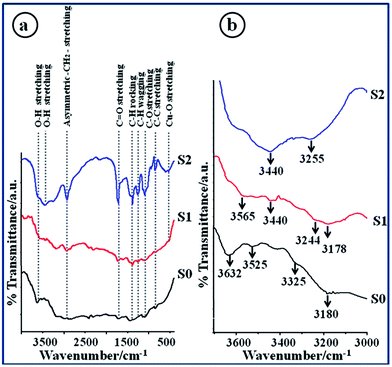 |
| Fig. 2 FT-IR spectra of pure PVA and the nanocomposites. (a) IR spectra across the entire region with the dotted lines indicating different vibrational modes of various chemical bonds. (b) IR spectra within the region 3000–3700 cm−1 to signify the effect of the CuO nanoparticles on the –OH functional group of PVA. | |
One particular mode of interaction between the metal oxide particles and the polymer can be the hydrogen bonding between the –OH functional groups of PVA and the oxygen in CuO. Fig. 2b focuses on the –OH functional group region of the FT-IR spectra (3000–3600 cm−1). The modification of the spectra from pure PVA membrane (S0) to the composites (S1 and S2) is apparent with the –OH defining positions becoming less delineated. Additionally, the positions appear to be blue shifted owing to the slight elongation of the O–H bond due to its hydrogen bond formation with the oxygen of CuO.
Further evidence of the strong interaction between the components of the CuO–PVA composites can be obtained from the photoluminescence (PL) spectra of the membranes (Fig. 3). PVA molecules exhibit photoluminescence emission in the visible region (within 400–500 nm region) due to a π* → n electronic transition in free –OH groups depending on their spatial distribution within oriented PVA molecules.39 In the case of our samples, two close prominent peaks observed for the pure PVA membrane at 420 nm and 434 nm can be attributed to transitions in syndiotactic (s) PVA and isotactic (i) PVA respectively. But, in the case of the two composite membranes the luminescence is almost quenched (shown as the inset of Fig. 3 with a magnified region for enhanced clarity) indicating that the presence of the CuO nanoparticles comprehensively reorders the delocalized n-electron system of the residual –OH groups of the PVA backbone.
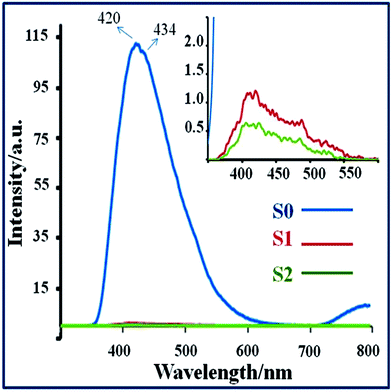 |
| Fig. 3 Photoluminescence (PL) spectra of pure PVA and the nanocomposites. The emission intensity for the CuO inclusive polymer membranes appears to be highly diminished. Inset provides the magnified PL spectra of the two CuO–PVA nanocomposites. | |
3.3 Microstructural features
Fig. 4 shows the microstructural features of the CuO nanoparticles, pure polymeric membranes and the composite films. The CuO particles were uniformly characterized by an elongated ellipsoidal morphology accompanied by a 100–200 nm average width and 600–800 nm average length (Fig. 4a and b). The edges of the particles appeared to be rather smooth as was suggested by the STEM image (Fig. 4b). The cross-sectional image of the particles revealed a papaya-like frame indicating the non-uniform accumulation of smaller CuO nanocrystals across the cross section (Fig. 4c). The pure PVA membrane consisted of beehive or mesh like structures dispersed within the PVA matrix (Fig. 4d).
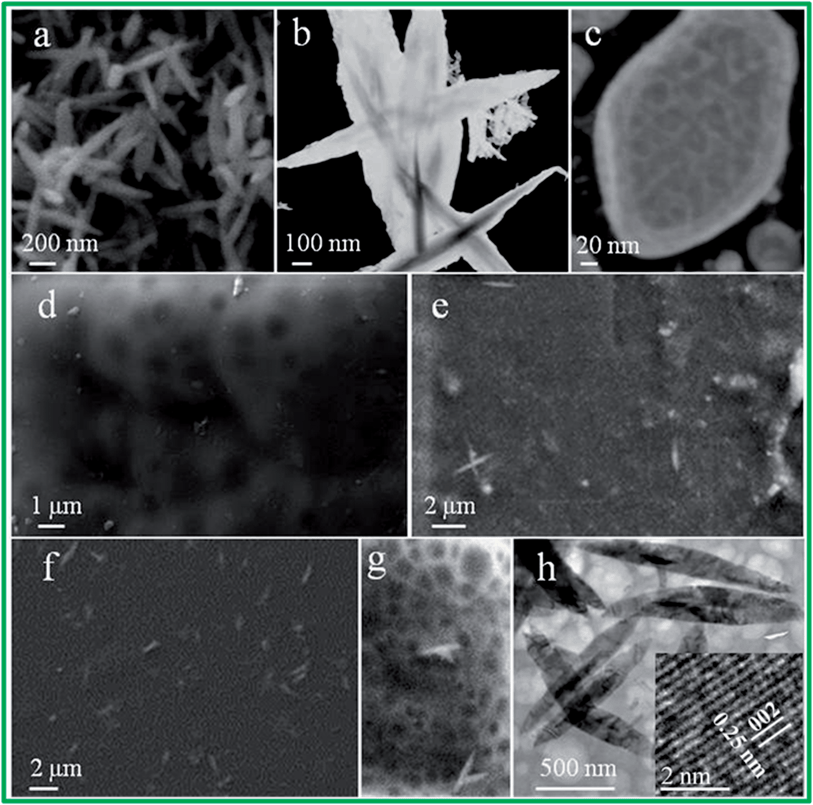 |
| Fig. 4 Micrographs of the CuO nanoparticles, PVA membranes and CuO–PVA composite membranes. (a) SEM image of the ellipsoidal shaped CuO nanoparticles, (b) STEM image of a few CuO nanoparticles, (c) STEM micrograph presenting the cross sectional image of a single CuO nanoellipsoid, (d) SEM micrograph of a pure PVA membrane showing mesh like microstructural characteristics, (e) SEM micrograph of CuO–PVA nanocomposite (S1) showing the presence of a few CuO nanoparticles on the polymer matrix, (f) SEM micrograph of CuO–PVA nanocomposite (S2) revealing a dense dispersion of CuO nanoparticles. (g) STEM image showing CuO nanoellipsoids attached to the mesh of PVA, (h) HRTEM image of S2 showing CuO nanoparticles dispersed without any agglomeration within the PVA matrix. Inset provides the lattice scale image of a dispersed CuO nanoparticle consisting of 002 lattice planes. | |
The microstructural images of the composites showed not only the presence but also the relative concentration of nanoparticles in the composite matrices. For the composite S1, a few CuO nanoellipsoids were found to be dispersed on the PVA matrix as revealed by the SEM micrograph of the sample (Fig. 4e). The corresponding SEM micrograph for S2 shows a higher density of CuO ellipsoids within the composite membranes (Fig. 4f), indicative of the higher CuO concentration. Fig. 4g shows the STEM image of S2 revealing the dispersion of CuO nanoellipsoids over the PVA mesh-like structure. CuO particles distributed over the PVA membrane. Fig. 4h shows the TEM image of the composite S2 revealing the random orientation of CuO nanoellipsoids while forming the composite membrane. The CuO–polymer interface was seemingly compact with no visible porosity or distortions observed. The lattice scale image of a dispersed CuO nanoellipsoid consisting of clear 002 lattice planes was provided in the inset. The microscopy images also refute the formation of CuO nanoparticle agglomerations within the polymer nanocomposites, thus denying the possibility of any significant physical or direct chemical interaction between the nanoparticles themselves within the polymer matrix.
The incorporation of CuO nanoellipsoids within the PVA matrix and the accompanying crosslinking through H bonding interactions between the oxygen atoms of CuO and the –OH functional groups from PVA have been depicted in Scheme 1.
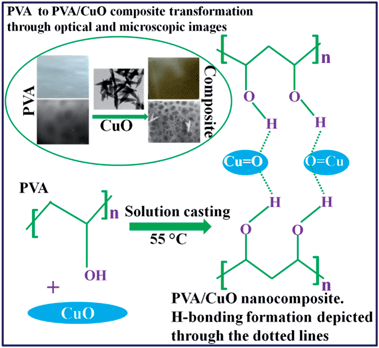 |
| Scheme 1 Transformation from pristine PVA to PVA–CuO composites, represented through optical and microscopic images and a schematic reaction diagram. | |
So, now that we know that the synthesized CuO nanoellipsoids were well dispersed within the PVA matrix and blended in via substantial interaction with the residual functional groups of the polymer, we shall focus on how they managed to improve the various important physicochemical properties of PVA.
3.4 Enhancement in thermal stability and conductivity
Thermal stability of the polymer nanocomposites was studied via analytical tools DSC and TGA. Both experiments were carried out under an argon atmosphere maintaining a heating rate of 10 °C min−1. Fig. 5a shows the DSC thermogram of the pristine polymer membrane and its composites emphasizing their melting and dissociating behaviors. The melting point (Tm) of the composites did not seem to have any shift in position relative to the pure polymer membrane, but the enthalpy of melting (ΔHm) attained a notable, even if little, improvement. This increase in melting enthalpy has a constructive effect on the mechanical properties of PVA due to its semi-crystalline nature. The enhanced crystallinity of the composites relative to the pure polymer as detected in the XRD spectra, possibly leads to enthalpy augmentation. Additionally the strong surface interaction between the nanoparticles and the polymer might have reduced the mobility of the PVA chain to some extent.
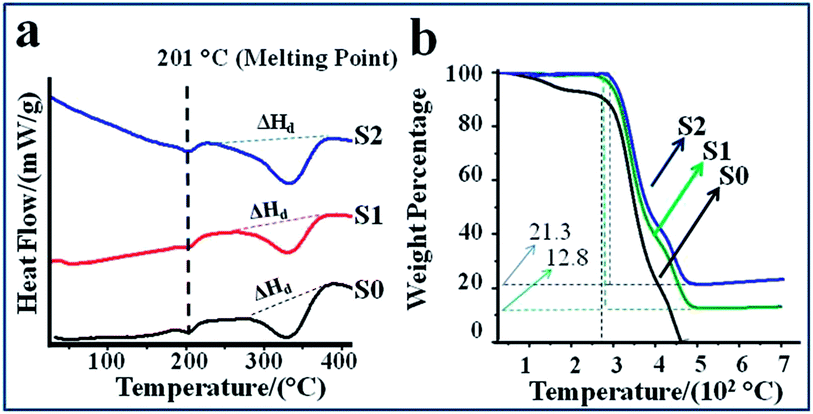 |
| Fig. 5 (a) DSC plot of pure PVA (S0) and the CuO–PVA nanocomposites (S1 & S2). (b) TGA curves of pure PVA (S0) and the CuO–PVA nanocomposites (S1 & S2). The residual weight after the completion of the degradation/pyrolysis reaction is indicated by the thin arrows. | |
However, the melting point of the polymer being unaffected indicates that the CuO nanoparticles had exerted their interaction mostly through the amorphous region of the polymer and the crystalline portion remained immune. Fig. 5b depicts the TGA thermogram of the samples which provides insight regarding the thermal decomposition behavior of the samples. Here, the initial weight loss (up to 260 °C) for S0 can be attributed to the vaporization of the adsorbed moisture and CO2 on the polymer membrane. Interestingly, the composites S1 and S2 barely show any initial weight loss within this temperature region indicating that, the addition of CuO nanoellipsoids has rendered the polymer membrane surface more resistant towards the adsorption of the aerial contaminants.
The beginning of the actual decomposition or thermal pyrolysis of the membranes is clearly observed in both DSC and TGA thermograms. The temperature at which this decomposition commences was determined to increase in the composites than in the pure PVA membrane (Table 1) and seems to move higher with the increment in CuO concentration. The degradation that is observed due to the chain stripping or condensation of –OH functional groups apparently becomes slower as we move from the pristine PVA to the composites as the slopes appear to be shifting towards higher temperature. The retardation of the decomposition can be further corroborated with the corresponding enthalpy (ΔHd) obtained from the DSC (Table 1), which clearly indicates that the degradation processes become increasingly more heat absorbing in the composites compared to the pristine PVA.
Table 1 Thermal parameters of pure PVA (S0) and CuO–PVA (S1 and S2) samples
Sample |
Melting point (Tm/°C) |
Melting enthalpy (ΔHm/J g−1) |
Beginning of pyrolysis (Td/°C) |
Enthalpy of degradation (ΔHd/J g−1) |
Residual weight (%) |
S0 |
201 |
22.1 |
264.2 |
219 |
— |
S1 |
201 |
24.3 |
274.7 |
242 |
12.8 |
S2 |
201 |
29.7 |
286.7 |
309 |
21.3 |
Another interesting observation via TGA was the presence of residual mass for the PVA–CuO composites, which was estimated to be 12.8% for S1 and 21.3% for S2. But for the pure PVA membrane no such mass was observed. The residual mass which could be a combination of organic olefins, alkenes and other aliphatic compounds and non-degraded polymer chain40 may be indicative of the fact that CuO could be acting as a catalyst surface for some of the side reactions leading to the organic chars or it could be that the interaction of CuO with the polymer chain simply made it partially prohibitive to condensation–degradation. At this moment, we cannot discard a cumulative effect of the two phenomena either.
Overall, the TG/DSC analysis suggests that the introduction of CuO nanofillers has significantly improved the polymer's thermal properties. The stability with respect to both melting and degradation in the composites seemed to be positively affected due to the strong interaction between the nanoparticles and PVA.
In fact, not only the thermal stability, but the thermal conductivity of the polymer too resulted in substantial enhancement as shown in Fig. 6. The thermal conductivity of the membranes was measured within the temperature range of 0–60 °C and it was found that the conductivity increased 50–60% over the entire temperature range in the nanocomposites compared to the pure polymeric membrane. Previously, an improvement of ∼30% in the thermal conductivity of nanodiamond incorporated PVA was reported.17 But spherical shape diamond nanoparticles, having a high thermal conductivity of their own (∼2000 W m−1 K−1), were found to be effective within the realm of theoretical predictions based on classical theories such as Maxwell's. In our case, however, the presence of ellipsoidal shaped CuO nanoparticles required a more specific theoretical model to predict the extent of enhancement. Lewis and Nielsen proposed such a theoretical model41 modifying Halpin–Tsai equations to incorporate various particle shapes. According to their model;
|
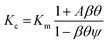 | (1) |
where,
Kc = thermal conductivity of the composite,
Km = thermal conductivity of the polymer matrix,
A = 2.8 (for aspect ratio 6),
33,43 θ = volume fraction of the nanofillers
|
 | (2) |
Kf = thermal conductivity of CuO = 77 W m
−1 K
−1 (
ref. 42)
|
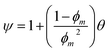 | (3) |
ϕm = packing fraction; assumed value 0.82 (for rod shaped randomly oriented particles, the closest approximation available).
43
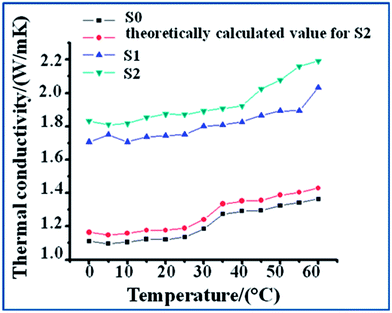 |
| Fig. 6 Thermal conductivity measurements of the pure PVA membrane (S0) and the CuO–PVA nanocomposites (S1 and S2) across a temperature range of 0–60 °C. Theoretically predicted values according to the Lewis–Nielsen equation for S2 have also been incorporated. | |
The calculated thermal conductivity values for composite S2 using the above model (Fig. 6; ●) accounted only for 5% of the observed enhancement. In fact any comprehensive explanation at the moment needs thorough experimental investigation coupled with theoretical base work. But here we must mention a couple of factors that might have augmented the thermal conductivity value beyond the theoretical prediction. The increased crystallinity is one such factor which would strongly enhance the phonon mode of vibration and thus increase the heat conduction. Besides, the effective medium theory for particles with high aspect ratios predicts very low interfacial resistance and thus high thermal conductivity for the nanocomposites.44
Not only the thermal properties, but also the introduced CuO nanoparticles with their inherent unique set of physical and chemical attributes and based on their strong interfacial interaction with PVA, could be assumed to significantly alter the polymer’s mechanical and electrical properties. First, we shall discuss the tensile properties.
3.5 Improved tensile characteristics
Fig. 7 presents the stress (σ)–strain (ε) curves for a PVA membrane and the CuO incorporated composites with different filler concentrations. The corresponding curve for PVA resembles a typical σ–ε curve for a polymer membrane with characteristic yielding behaviour. The curves for the composite membranes represent similar behaviour albeit with different magnitudes for the stress–strain values.
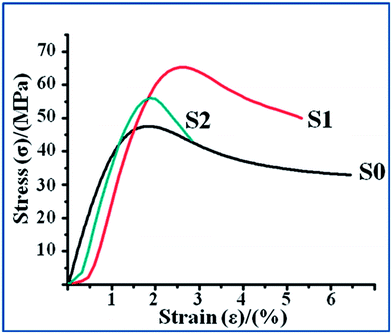 |
| Fig. 7 Stress–strain curves for pure PVA membrane (S0), CuO/PVA = 1/500 (S1), CuO/PVA = 1/50 (S2). | |
Table 2 lists these values for each of the samples. Following the addition of 0.2 wt% CuO nanoparticles (S1), the Young’s modulus for the polymer increased (from 4.28 GPa to 4.99 GPa) and the tensile strength increased 38% (from 47.56 MPa to 65.37 MPa). The elongation at break also increased indicating that the composite membrane is somewhat less brittle compared to pure PVA. This is confirmed by the calculated toughness values which suggest S1 to be slightly less brittle compared to S0.
Table 2 Tensile parameters of pure PVA (S0), and CuO–PVA nanocomposites (S1 and S2)a
Sample |
Elongation at break (%) |
Tensile strength (MPa) |
Young's modulus (GPa) |
Toughness (J g−1) |
The toughness value was calculated as the area enclosed by the stress–strain curve divided by density (g m−3) of the corresponding material. |
S0 |
1.81 |
47.56 |
4.28 |
196.6 |
S1 |
2.55 |
65.37 |
4.99 |
201.1 |
S2 |
1.82 |
56.09 |
4.79 |
88.2 |
The increase in elastic modulus can be predicted by applying the simple rule of mixing and utilizing the following equation as a first approximation
|
Ec = ECuOVCuO + EPVA(1 − VCuO)
| (4) |
where,
EC,
ECuO, and
EPVA are the elastic modulus of the composite, CuO and PVA respectively.
VCuO is the volume fraction of CuO nanoparticles present within the polymer matrix.
Assuming ECuO = 81.6 GPa,45 EPVA = 4.28 GPa and that 0.2 wt% CuO corresponds to VCuO = 0.0106, the calculated EC = 5.09 GPa, which is comparable to the value that we obtained (= 4.99).
From a more abstract perspective, the improvement observed can be largely attributed to the nanosize of the fillers resulting in a sufficiently high interfacial contact area with the polymers and thus providing strong interaction to prevent the stretching of amorphous chains or sheer yielding of crystallites. The improved crystallinity in the composite materials also can be one of the causes for the increased elastic modulus. However, such improvements do not get reciprocated for S2 which, apart from its tensile strength (56.09 MPa), shows properties which are comparable to PVA. The toughness value shows that S2 has become much more brittle compared to pure PVA. The reason behind this may be that the excess of CuO nanoparticles provides a number of entanglements within the polymer matrix. Besides, the large number of hydrogen bonds formed outweighs the interfacial interaction and makes the polymer susceptible towards deformation induced by weakening of the bonds within the polymer chain and thus rendering it easier to be stretched. So, the admixture of excess CuO nanoparticles is unfavourable for the promotion of the polymer’s tensile properties.
3.6 Modification of electrical properties
The dielectric properties of PVA and the CuO composite membranes were studied through capacitance measurement of rectangular strips cut off from the original membranes. Fig. 8a presents the plot depicting the variation of the real part of the dielectric constant (εr) with frequency of the alternating electric field (ω) applied. Notable observations from the plot are (i) CuO nanofillers enhanced the dielectric constant of PVA, the enhancement was around two fold in the lower frequency region but dropped off as we increased the frequency; (ii) the composites were more responsive towards the changing frequency than the pure polymeric membrane and (iii) the relative concentration of the CuO nanoparticles in the two composites did not induce a lot of difference in their respective dielectric constants. To rationalize these observations we have to deliberate over the various components of dielectric polarization (i) atomic, (ii) electronic, (iii) orientational and for heterogeneous systems such as our composite material there is the feasibility of another component (iv) interfacial polarization. The presence of the polymeric matrix causes some of the charge carriers of CuO to get trapped which in turn induces the interfacial polarization within the composite systems. In the low frequency region, this component, induced by the nanoellipsoids of CuO, elevates the dielectric constant of PVA. But the interfacial polarization and orientational polarization also happen to be the two slowest components in terms of attaining equilibrium with the alternating frequency of the current. And so, as we increase the frequency of the current the contribution from these two components becomes increasingly smaller and the εr value goes down rapidly. In the MHz region the corresponding εr values of the pure polymer and the CuO composites are almost comparable. The interfacial polarization effect was less pronounced in the dissipation factor or tan
δ spectra of the samples (Fig. 8b). Here too, the dissipation factor decreases with increasing frequency, illustrating the relaxation process. The low dielectric loss of the polymeric membrane which remained almost the same even after the inclusion of the CuO nanoparticles bodes well for the composites’ application as an insulating material. The relatively higher value of dielectric loss at a lower frequency could be due to the mobile charges in the polymer backbone.
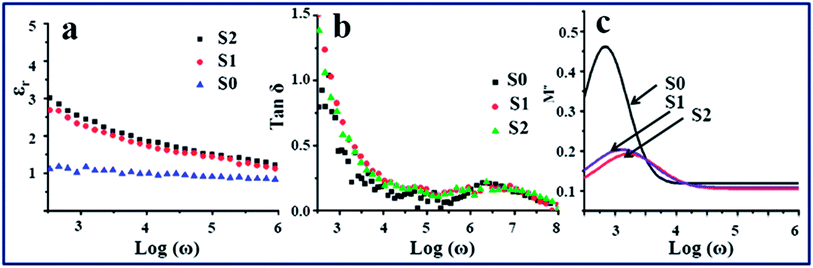 |
| Fig. 8 Representative plots for the dielectric properties of pure PVA membrane (S0) and the PVA–CuO nanocomposites membranes (S1 and S2); (a) real part of the dielectric permittivity (εr) against logarithm of the frequency (ω), (b) loss tangent (tan δ) against log(ω) and (c) electric loss modulus (M′′) against log(ω). | |
The presence of the bulk relaxation phenomenon for both the polymer and its composites was evident by the peak formation in the loss modulus spectra (Fig. 8c). Interestingly, the maxima of the peak decrease substantially in the composites and also shift towards higher frequency indicating diminished relaxation time. The calculated relaxation time was of the order of 10−3 in the pure PVA membranes and the order of 10−4 in the composites. The broadening of the peaks in the composites compared to the pure polymeric sample can be attributed to the interaction between the nanofillers of CuO.
3.7 Optical transparency
Fig. 9 displays the UV-Vis spectra of the pure PVA membrane and the two CuO incorporated nanocomposites membranes. The PVA membrane was measured to have transparency well above 90% across the visible region. Such high transparency enables PVA films to be utilized as polarizer films in electrical devices. The observed trough at around 265 nm in the spectra arises due to a π → π* transition in the PVA molecule. With the introduction of CuO nanoellipsoids the transparency value became slightly lower in the two composites, but never the less maintained a very high value of more than 80%. The lowering can be attributed to the scattering of light caused by the nanosized CuO particles present within the matrix.
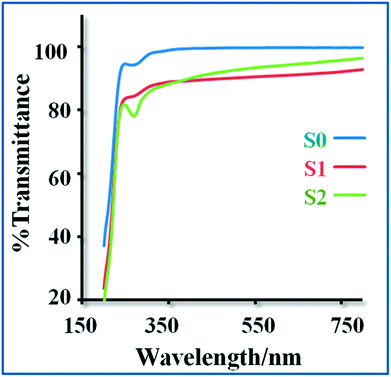 |
| Fig. 9 UV-Vis spectra of PVA and the composites. | |
4. Conclusions
We have prepared CuO nanoellipsoid incorporated nanocomposites of PVA with improved physicochemical properties. A simple solution casting method was applied during the synthesis. The nanocomposites possessed enhanced crystallinity compared to the pure PVA membrane. Strong host–filler interactions and the presence of H-bonding were established through the vibrational spectroscopy and photoluminescence studies. The thermal stability in terms of the energy required to melt the polymer membrane or the pyrolysis of the polymer chain was observed to increase in the composites. The degradation temperature was also enhanced slightly. Thermal conductivity of the composite membranes increased 50–60% across a temperature range of 0–60 °C. The increment was beyond the theoretical prediction of modified Halpin–Tsai equation, indicating strong interactions between the host polymer and the filler nanoparticles. The tensile strength and elastic modulus were both superior for the composites compared to the pure PVA and the toughness was comparable. The Young's modulus value was comparable to that of 0.2 wt% loading and thus ideal reinforcement was revealed. The nanoparticles also improved the dielectric constant nearly two-fold without compromising its dielectric loss properties. Optical transparency of the PVA films remained quite high even after the incorporation of nanoparticles. Overall, we have been able to prepare high quality PVA nanocomposite films by using economic and easily processable CuO nanoparticles and the improved properties indicated that CuO can be a suitable alternative to the conventional carbon based nano-fillers.
Acknowledgements
We thank the Director, NPL, New Delhi, India for providing the necessary experimental facilities. Dr A. Dhar, Dr S. N. Sharma, Mr K. N. Sood and Mr P. C. Mandal are gratefully acknowledged for providing the necessary instrumentation facilities for XRD, FT-IR, SEM and UV-Vis spectroscopy respectively. We thank Dr B. P. Singh for letting us carry out the experiments regarding mechanical properties. We also express our gratitude towards Dr A. M. Biradar and Dr J. P. Rana for the dielectric property measurements. Kajal Kumar Dey acknowledges the financial support from Council of Scientific and industrial research, India (Grant no. 31/001(0325/2009-EMR-I)).
References
- G. Swift, Acc. Chem. Res., 1993, 26, 105 CrossRef CAS.
- W. R. Gombotz and D. K. Pettit, Bioconjugate Chem., 1995, 6, 332 CrossRef CAS.
- R. Wang, W. Chen, F. Meng, R. Cheng, C. Deng, J. Feijen and Z. Zhong, Macromolecules, 2011, 44, 6009 CrossRef CAS.
- P. Podsiadlo, A. K. Kaushik, E. M. Arruda, A. M. Waas, B. S. Shim, J. Xu, H. Nandivada, B. G. Pumplin, J. Lahann, A. Ramamoorthy and N. A. Kotov, Science, 2007, 318, 80 CrossRef CAS PubMed.
- T. C. Merkel, B. D. Freeman, R. J. Spontak, Z. He, I. Pinnau, P. Meakin and A. J. Hill, Science, 2002, 296, 519 CrossRef CAS PubMed.
- R. Ricciardi, F. Auriemma, C. D. Rosa and F. Lauprȇtre, Macromolecules, 2004, 37, 1921 CrossRef CAS.
- J. Wang, X. Wang, C. Xu, M. Zhanga and X. Shang, Polym. Int., 2011, 60, 816 CrossRef CAS.
- C. M. Hassan and N. A. Peppas, Adv. Polym. Sci., 2000, 153, 37 CrossRef CAS.
- N. A. Peppas, P. Bures, W. Leobandung and H. Ichikawa, Eur. J. Pharm. Biopharm., 2000, 50, 27 CrossRef CAS.
- V. I. Lozinsky, Trends Biotechnol., 2003, 21, 445 CrossRef CAS PubMed.
- S. A. Paralikar, J. Simonsen and J. Lombardi, J. Membr. Sci., 2008, 320, 248 CrossRef CAS PubMed.
- D. M. Fernandes, A. A. W. Hechenleitnera, S. M. Limab, L. H. C. Andradeb, A. R. L. Cairesc and E. A. Gómez Pineda, Mater. Chem. Phys., 2011, 128, 371 CrossRef CAS PubMed.
- M. Cadek, J. N. Coleman, V. Barron, K. Hedicke and W. J. Blau, Appl. Phys. Lett., 2002, 81, 5123 CrossRef CAS PubMed.
- L. Liu, A. H. Barber, S. Nuriel and H. D. Wagner, Adv. Funct. Mater., 2005, 17, 975 CrossRef.
- H. K. F. Cheng, N. G. Sahoo, Y. P. Tan, Y. Pan, H. Bao, L. Li, S. H. Chan and J. Zhao, ACS Appl. Mater. Interfaces, 2012, 4, 2387 CAS.
- X. Zhao, Q. Zhang and D. Chen, Macromolecules, 2010, 43, 2357 CrossRef CAS.
- S. Morimune, M. Kotera, T. Nishino, K. Goto and K. Hata, Macromolecules, 2011, 44, 4415 CrossRef CAS.
- L. Deng, S. J. Eichhorm, C.-C. Kao and R. J. Young, ACS Appl. Mater. Interfaces, 2011, 3, 433 CAS.
- J.-H. Yang and Y.-D. Lee, J. Mater. Chem., 2012, 22, 8512 RSC.
- J. George, V. A. Sajeevkumar, K. V. Ramana, S. N. Sabapathy and Siddaramaiah, J. Mater. Chem., 2012, 22, 22433 RSC.
- J. George, K. K. R. Dutta, V. A. Sanjeevkumar, S. N. Sabapathy, A. S. Bawa and M. Eswaramoorthy, ACS Appl. Mater. Interfaces, 2009, 1, 2796 Search PubMed.
- T. Ung, L. M. Liz-Marzán and P. Mulvaney, J. Phys. Chem. B, 2001, 105, 3441 CrossRef CAS.
- I. Hussain, M. Brust, A. J. Papworth and A. I. Cooper, Langmuir, 2003, 19, 4831 CrossRef CAS.
- Y. Wang, A. Suna, W. Mahler and R. Kasowski, J. Chem. Phys., 1987, 87, 7315 CrossRef CAS PubMed.
- R. V. Kumar, Y. Koltypin, Y. S. Cohen, D. Aurbach, O. Palchik, I. Felner and A. Gedanken, J. Mater. Chem., 2000, 10, 1125 RSC.
- D. M. Fernandesa, A. A. W. Hechenleitnera, S. M. Limab, L. H. C. Andradeb, A. R. L. Cairesc and E. A. G. Pinedaa, Mater. Chem. Phys., 2011, 128, 371 CrossRef PubMed.
- Z. Guo, D. Zhang, S. Wei, Z. Wang, A. B. Karki, Y. Li, P. Bernazzani, D. P. Young, J. A. Gomes, D. L. Cocke and T. C. Ho, J. Nanopart. Res., 2010, 12, 2415 CrossRef CAS.
- E. Tuncer, I. Sauers, D. R. James, A. R. Ellis, M. P. Paranthaman, A. Goyal and K. L. More, Nanotechnology, 2007, 18, 352704 Search PubMed.
- S. Gupta, S. Sindhu, K. A. Varman, P. C. Ramamurthy and G. Madras, RSC Adv., 2012, 2, 11536 RSC.
- S. Gandhi, R. H. H. Subramani, T. Ramakrishnan, A. Sivabalan, V. Dhanalakshmi, M. R. Gopinathan Nair and R. Anbarasan, J. Mater. Sci., 2010, 45, 1688 CrossRef CAS.
- R. Vijaya Kumar, R. Elgamiel, Y. Diamant, A. Gedanken and J. Norwig, Langmuir, 2001, 17, 1406 CrossRef.
- B. Polyakov, L. M. Dorogin, S. Vlassov, M. Antsov, P. Kulis, I. Kink and R. Lohmus, Eur. Phys. J. B, 2012, 85, 366 CrossRef.
- K. K. Dey, A. Kumar, R. Shanker, A. Dhawan, M. Wan, R. R. Yadav and A. K. Srivastava, RSC Adv., 2012, 2, 1387 RSC.
- F. M. Chang, S. L. Cheng, S. J. Hong, Y. J. Sheng and H. K. Tsao, Appl. Phys. Lett., 2010, 96, 114101 CrossRef PubMed.
- S. Mitra, O. Mondal, D. R. Saha, A. Datta, S. Banerjee and D. Chakravorty, J. Phys. Chem. C, 2011, 115, 14285 CAS.
- N. Bouropoulos, G. C. Psarras, N. Moustakas, A. Chrissanthopoulos and S. Baskoutas, Phys. Status Solidi A, 2008, 205, 2033 CrossRef CAS.
- S. Sarkar, P. K. Jana, B. K. Chaudhuri and H. Sakata, Appl. Phys. Lett., 2006, 89, 212905 CrossRef PubMed.
- J. W. Chen and G. N. Rao, IEEE Trans. Magn., 2011, 47, 3772 CrossRef CAS.
- S. Ram and T. K. Mandal, Chem. Phys., 2004, 303, 128 CrossRef PubMed.
- J. W. Gilman, D. L. VanderHart and T. Kashiwag, ACS Symp. Ser., 1994, 11, 161 Search PubMed.
- T. Lewis and L. Nielsen, J. Appl. Polym. Sci., 1973, 29, 3819 Search PubMed.
- J. Gangwar, K. K. Dey, S. K. Tripathi, M. Wan, R. R. Yadav, R. K. Singh, Samta and A. K. Srivastava, Nanotechnology, 2013, 24, 415705 CrossRef PubMed.
- I. H. Tavman, Int. Commun. Heat Mass Transfer, 1998, 25, 723 CrossRef CAS.
- C. W. Nan, R. Birringer, D. R. Clarke and H. Gleiter, J. Appl. Phys., 1997, 81, 6692 CrossRef CAS PubMed.
- E. P. S. Tan, Y. Zhu, T. Yu, L. Dai, C. H. Sow, V. B. C. Tan and C. T. Lim, Appl. Phys. Lett., 2007, 90, 163112 CrossRef PubMed.
|
This journal is © The Royal Society of Chemistry 2014 |
Click here to see how this site uses Cookies. View our privacy policy here.