DOI:
10.1039/C3RA44345K
(Paper)
RSC Adv., 2014,
4, 8421-8430
Silica supported ceria nanoparticles: a hybrid nanostructure to increase stability and surface reactivity of nano-crystalline ceria†
Received
14th August 2013
, Accepted 18th November 2013
First published on 21st November 2013
Abstract
The mixed oxidation state (3+/4+) of ceria nanoparticles of smaller sizes make them attractive materials for their catalytic antioxidant biological properties. However the unmodified smaller ceria nanoparticles are limited in their use due to particles agglomeration and reduced surface chemical reactivity in the solutions used to disperse the nanoparticles. This work describes an effort to stabilize small ceria nanoparticles, retaining their desired activity, on a larger stable silica support. The ceria nanoparticles attached to silica was synthesized by a solution synthesis technique in which the surface functional groups of silica nanoparticles were found to be essential for the formation of smaller ceria nanoparticles. The surface chemical and vibrational spectroscopy analysis revealed cerium–silicate (Ce–O–Si) covalent bond linkage between silica and cerium oxide nanoparticles. The colloidal properties (agglomerate particle size and suspension stability) of ceria attached to silica was significantly improved due to inherent physico–chemical characteristics of silica against random collision and gravitation settling as opposed to unmodified ceria nanoparticles in solution. The bio-catalytic activity of ceria nanoparticles in the 3+ oxidation state was not found to be limited by attachment to the silica support as measured by free radical scavenging activity in different biological media conditions.
1. Introduction
Within the category of oxides, cerium oxide nanoparticles are found to be useful for their redox active properties. For some applications the useful properties of ceria appear when nanoparticles are partially reduced (mixed Ce 3+/Ce 4+ valence state). The redox active properties (reversible oxidation state between Ce 3+ and Ce 4+) appear to allow the ceria nanoparticles to act as free radical scavengers to prevent oxidative stress related cellular damage and this antioxidant property has made them attractive to several research groups studying structure–property relationships related to biomedical applications.1–4 Several synthesis approaches have been used to produce such particles and these include producing particles with grain sizes less than 5 nm (which stabilizes more Ce 3+), adding various dopants, or by synthesis conditions.5–7
Many different factors including the synthesis approach, environmental exposure, storage conditions, and processing or testing (e.g. aggregation to form larger crystals) exposure have been demonstrated to influence the physico–chemical properties of ceria nanoparticles.8,9 An intrinsic instability of the nanoparticles in aqueous media conditions over a longer ageing period has been demonstrated to have a direct influence on the fate and impact of nanoparticles used for biological applications.10–12 For example, ceria nanoparticles can remain as single particles in aqueous media or aggregate to bigger sizes, thereby reducing the desirable size dependent properties and decreasing their stability in suspension, which are important properties for biomedical applications. In addition to the particle size, the chemical state of ceria particles in solution has been observed to vary with time and environmental conditions.13 Hence long term stability of particles in different environmental conditions (pH, ionic strength, presence of biomolecules, etc.) can be a barrier in the production of functional particles. Therefore it is of importance to develop stabilization methods by which the desired physical and chemical properties of cerium oxide nanoparticles, often with sizes below 10 nm, can be maintained for longer periods of time.
Stabilization of cerium oxide nanoparticles in aqueous media conditions has been often achieved by surface functionalization or grafting with polymer, surfactants or surface ligands. Surface functionalization with organic moieties can increase the surface charge density to provide enough steric or electrostatic repulsive forces for particles to remain non-aggregated and stable in the colloidal state.14 Surface modification of nanoparticles with polymers or surfactants often requires elaborate synthetic schemes and can be a cumbersome process. Moreover surface modification with organic chemical groups can also alter the nanoparticle surface interactions by changing surface properties and limit their application for a desired biological end use.15,16 The long term stability of surfactant or polymer surface functionalized nanoparticles may be limited due to instability of surface ligands under harsh environmental conditions like highly acidic conditions, or enzymatic degradation.17,18 Nanoparticles stabilized by electrostatic repulsive functional groups can agglomerate to bigger sizes due to surface charge neutralization upon contact with highly ionic biological media conditions. The extent of particles agglomeration can affect the biological endpoints where cells uptake and behavior or translocation within different organs can be different. Hence the need to develop a ceria nanostructure with excellent stability, controlled size, and re-dispersibility in biological relevant media conditions.
The ceria attached to silica materials is attractive for chemical mechanical polishing in the semiconductor industry and such materials are utilized as abrasive elements due to their high polishing rates and selective removal.19 However attempts to directly coat ceria to the silica surface have proven to be a difficult task.20–22 Most studies have focused on fabricating silica–ceria composites and studied their properties as abrasives without any detailed analysis of silica–ceria interactions or suspension stability. Frequently the synthesis of ceria attached to silica nanoparticles involved post heat treatment which lead to hard aggregation and non-homogenous coatings.20 To the best of our knowledge there is no reported literature on ceria–silica nanostructure hybrids developed for biomedical applications with a focus on optimizing the dispersibility of unstabilized cerium oxide nanoparticles powder in biologically relevant media.
In this study novel hybrid nanostructures consisting of small ceria particles attached to larger silica particles have been designed and synthesized using a wet chemical synthesis technique. The larger silica particles of size ∼50–60 nm synthesized by sol–gel chemical process was selected to be used as a guest template to grow small ceria nanoparticles. The silica particles enable only limited diffusivity and interparticle interactions in solution by their larger size and high surface charge density which provides improved dispersibility and stability for small ceria nanoparticles which are highly unstable in solution. The particle size and structural characteristics of the silica–ceria hybrid nanostructure were analyzed by dynamic light scattering (DLS) X-ray diffraction (XRD), scanning electron microscopy (SEM), and transmission electron microscopy (TEM) imaging techniques including energy dispersive X-ray chemical analysis (EDX). The nature of bonding between silica and ceria nanoparticles were characterized by X-ray photoelectron spectroscopy (XPS) and Fourier transform infrared spectroscopy (FTIR) analysis. The silica–ceria hybrid nanostructure solution behavior was compared with unstabilized ceria by comparing the particle size changes and settling velocity in different biological media conditions. The bio-catalytic activity of the silica–ceria nanostructure was characterized by measuring the superoxide dismutase (SOD) mimetic activity in different biological media conditions in comparison to a control reagent.
2. Experimental section
2.1. Chemicals
The chemicals used in this work are tetraethylorthosilicate (TEOS, Sigma-Aldrich), cerium(III) nitrate hexahydrate (99.99%, Sigma-Aldrich), urea (≥98%, Sigma-Aldrich), ethanol (200 proof, Sigma-Aldrich), and ammonium hydroxide (28–30%). Unmodified ceria nanoparticles powders of mean size 8 nm were purchased commercially from Melorium Technologies (product # 09817). The deionized (DI) water used in our experiments has resistance greater than 18 Ohm at room temperature. All chemicals were used as received without any further modification.
2.2. Synthesis of the silica nanoparticles template
The silica nanoparticles of average size 50–60 nm were synthesized by a modified Stöber synthesis process.23 The initial reaction mixture consisted of 114 ml ethanol and 5.7 ml ammonium hydroxide in a 250 ml conical flask. The above mixture was stirred for 15 min followed by drop wise addition of 3.8 ml tetraethylorthosilicate; the final mixture was allowed to undergo vigorous stirring for 15 h at room temperature. The final silica nanoparticles sol obtained appeared milky white in color and was used as prepared without further modification.
2.3. Synthesis of the silica–ceria hybrid nanostructure
The silica–ceria nanostructure was synthesized by a solution chemical synthesis process previously reported for GaN/SiO2 nanocomposites.24 In common with this procedure a required amount of aqueous urea (0.6 gm/3 ml DI water) was added to 15 ml of prepared silica nanoparticles solution and the mixture was stirred (350 rpm) for 15 min at 85 °C. The ceria nanoparticles precursor was prepared by dissolving 0.0938 gm of cerium(III) nitrate hexahydrate in 1 ml DI water. This solution was then added to the urea–silica mixture and continued to stir for another 3 h at 85 °C. The ratio of Ce–Si in the final product was calculated as 5 wt%. The resulting yellowish colored solution was centrifuged to isolate the particles obtained as precipitates. The final product was washed 3 times with DI water and finally with anhydrous ethanol followed by drying at room temperature for 24 h.
2.4. Preparation of the silica–ceria hybrid nanostructure suspension in biological relevant media
An aqueous solution of silica–ceria was prepared in DI water at a concentration of 40 mg ml−1. The prepared suspension was dispersed by sonication for 15 min in a water ultrasonicator (Model: Branson 2510). The prepared nanoparticle suspension was used as a stock solution for further dispersion in other aqueous media.
2.5. Characterization of the silica–ceria hybrid nanostructure
Dynamic light scattering (DLS) particle size measurements. The average median or effective diameters (Z-average) of the particles were measured by dynamic laser light scattering measurements. The instrument setup used for measurements was Brookhaven Zeta PALS particle size analyzer with a fixed He–Ne laser. All the measurements were performed at 90° scattering angle. The number average particle size and/or Z-average were derived from correlation function and cumulant size analysis using particle sizing software. The size analysis was conducted except unless noted by dispersing the dry powder particles at a concentration of not less than 10 μg ml−1. All sample preparation was made at room temperature. Before each measurement the nanoparticles dispersed solution was sonicated for a few minutes and vortexed for uniform dispersion. The final volume of particle dispersion used for size measurements was ∼1 ml.
X-ray diffraction (XRD) analysis. A portion of the particles was smeared uniformly over the double sided tape attached to metal sample holder for X-ray diffraction (XRD) measurements which were generated using a Cr Kα (2.2897 Å, 40 kV, 40 mA) radiation source. The measurements were performed using a 0.3 mm collimator under the following measurement conditions: Omega 25–45 deg., Phi 0–360 deg. The intensity data were collected over a 2θ range of 10–180°. The average crystallite size was calculated using the Debye–Scherer equation. The standard cubic indexation method was used to calculate the cell “a” parameter value for the prominent ceria base peak (111).
Scanning electron microscopy (SEM)/energy dispersive X-ray spectroscopy (EDS) measurements. The SEM and EDS measurement was done using FEI Quanta (Dual FIB/SEM). For image analysis, a portion of the particles was deposited uniformly over double sided tape mounted on a metal stub. Before imaging, a thin conducting layer of carbon film was deposited on the surface for better conductivity.
High resolution transmission electron microscopy (HRTEM) analysis. A JEOL JEM 2010 TEM was used to acquire the electron microscopy images at accelerating voltage of 200 keV. The sample preparation included dispersing the sample in DI water and sonication for 10 min followed by drop casting few drops of as prepared dispersion on a lacey copper grid (200 mesh) coated with carbon. The grids were allowed to dry at room temperature for ∼24 h before the actual analysis.
X-ray photoelectron spectroscopy (XPS) analysis. XPS was used to determine the chemical speciation and elemental composition of silica–ceria hybrid nanostructures. The XPS measurements were performed using a Phi 5000 Versa Probe. This system consisted of a monochromatic focused Al Kα X-ray (1486.7 eV) source and a hemispherical analyzer. A 25 W X-ray beam with 100 μm in diameter was incident normal to the sample surface and the photoelectrons were collected at 45° relative to the surface normal. Wide energy scan data were collected using pass energy of 117.4 eV. High-resolution scans were obtained using pass energy of 23.5 eV. Low energy electrons at ∼1 eV, 40 μA and low energy Ar+ ions (5 eV) were used to minimize the surface charging. The XPS spectra were calibrated to an energy scale with binding energies for Cu 2p3/2 at 932.67 ± 0.05 eV and Au 4f at 84.0 ± 0.05 eV.
Fourier transform infrared spectroscopy (FTIR) analysis. For FTIR measurements solid loose silica supported ceria powder was made into a pellet by mixing with dried KBr in ∼10
:
1 ratio. The measurements were performed using a Thermo Scientific FTIR spectrometer, model Nicolet iS10. All the measurements were an average of 50 scans and recorded at resolution of 1 cm−1.
2.6. Colloidal stability characteristics of the silica–ceria hybrid nanostructure in aqueous biological media conditions
Agglomeration kinetics of the silica–ceria hybrid nanostructure. The agglomeration kinetics of the unmodified and silica–ceria hybrid nanostructure was monitored by dynamic light scattering measurements over a period of 2 h. The nanoparticles were diluted from stock solution to 10 μg ml−1 concentration in the respective solutions. The effect of pH on the stability of the nanoparticles was tested at constant ionic strength solution of 0.02 M NaCl with the pH of the solution adjusted using either HCl or NaOH. The particles suspension was sonicated for 3 min and vortexed for 10 seconds before starting the first measurement and left undisturbed for subsequent measurements.
Characterization of the suspension stability by sedimentation kinetics. The unmodified ceria and silica–ceria hybrid nanostructure settling velocity was evaluated by characterizing time dependent suspension stability analysis. The sedimentation of the nanostructure was monitored by in situ absorbance measurements over a period of 12 h with 30 min time interval between each measurement. The stock nanoparticles suspension (40 mg ml−1) was diluted to a concentration of 25 μg ml−1 in the required testing media, DI water, phosphate buffer saline (PBS) or complete cell culture media (CCM), RPMI (Roswell Park Memorial Institute Medium) with 10 vol% fetal bovine serum (FBS). The diluted nanoparticle suspension was vortex mixed in a vortexer (Fisher Vortex, Gene 2) for 15–20 seconds. The changes in maximum absorbance (λmax ∼ 300–320 nm) intensity of ceria nanoparticles in different aqueous media was recorded as a function of time in a 10 mm path length cuvette using a UV-Vis spectrometer (Shimadzu UV-2501 PC). The suspension stability index was calculated as % of initial absorbency at t = 0 and other subsequent time points up to 12 h.
Analysis of SOD activity of silica–ceria hybrid nanostructures. The bio-catalytic activity of the silica–ceria hybrid nanostructure was analyzed by SOD mimetic assay. The procedure for SOD mimetic activity was followed as prescribed in the Trevigen's HT Superoxide Dismutase Assay Kit (Cat# 7501-500-K). In general the superoxide radical generated from the conversion of xanthine by xanthine oxidase converts WST-1 to WST-1 formazan, a colored reagent, which absorbs light at 450 nm. In our experiment silica supported ceria nanoparticles dispersed in different biological relevant media conditions was assessed for reduction of superoxide ion concentrations in competition to the SOD control reagent. The measure of silica supported ceria nanoparticles SOD activity was represented by the extent of reduction in the appearance of WST-1 formazan measured using an absorbance plate reader.
3. Results and discussion
3.1. Particle characterization and structural evolution
A schematic illustration of the steps followed in the synthesis of the silica–ceria hybrid nanostructure is shown in Scheme 1. The nano-crystalline ceria nanoparticles were synthesized using sol–gel derived silica nanoparticles as a guest matrix. The surface functional groups of silica mediated the growth of the nano-crystalline ceria nanoparticles. The as synthesized silica–ceria hybrid was isolated and washed by centrifugation, dried at room temperature and processed as a loose powder for further characterization. The formation of cerium oxide was indicated by color change of the reaction solution from colorless to pale yellow (oxide). The particle size, crystallinity, and morphology of the silica supported ceria nanoparticles was characterized DLS particle size measurements, XRD and SEM/EDX analysis as described in the later sections.
 |
| Scheme 1 Schematic representation of the synthesis of the silica–ceria hybrid nanostructure by a solution chemical synthesis process. The larger silica nanoparticles (∼50–60 nm) were used as a support matrix to grow and stabilize small cerium oxide nanoparticles. | |
3.2. Particle size characterization
Fig. 1 shows the particle size characterization for the silica–ceria hybrid nanostructure by DLS size analysis. The silica particles size was measured as an average median size of 68.7 nm and after the attachment of ceria showed broad size distribution with an increase in the average median size to 71.1 nm. However the control unmodified ceria nanoparticles agglomerated to average median size of 300 nm (Fig. 1). The small increase in average median particle size (∼2 to 3 nm) and broader size distribution can be ascribed to a change in hydrodynamic size of the silica particles due to the attachment of ceria. This observation is supported by the polydispersity index (PDI) values derived from cumulant analysis based on particle size distribution width. The PDI for bare silica particles was measured as 0.018 representing the monodisperse silica particles. After ceria attachment the PDI for silica particles increased to 0.31 consistent with broad particle size distribution where homogenous particle size is disturbed by ceria particles attachment and may be due to agglomeration of non-attached ceria particles as seen by the size distribution of silica tailing on the lower side. Similarly the PDI for bare ceria particles was measured as 0.384 due to the intense agglomeration of small ceria particles present in the suspension. The silica nanoparticles were observed to be more stable in the dispersion medium due to the strong electrostatic stabilization which renders them dispersible in aqueous media. This allowed for the facile dispersion of small ceria nanoparticles attached to silica without any significant agglomeration. However unstabilized small ceria nanoparticles without any surface stabilizing agent agglomerated intensively due to the weak repulsive forces between particles which is related to the diffusivity of the particles in solution and extends to favorable conditions for an increase in collision efficiency. In the following section we have further characterized the “ageing solution stability” of silica–ceria hybrids against agglomeration and settling in different biological media conditions as compared to unmodified ceria nanoparticles.
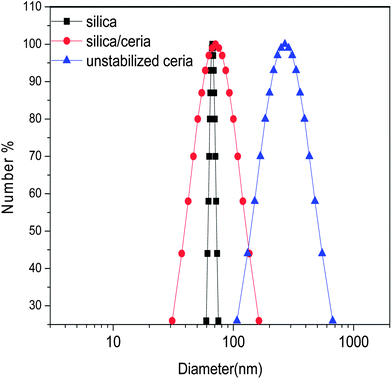 |
| Fig. 1 DLS particle size analysis for silica template, silica–ceria hybrid and for comparison of unmodified ceria particles of similar size range. The silica particle size distribution was measured using the as synthesized silica sol without any further dilution. For the silica–ceria hybrid and unmodified ceria particle size measurements, DI water was used as the solvent for dispersion. | |
The XRD analysis was performed to characterize the phase purity and crystallinity of the pure silica, unsupported ceria (control), and silica–ceria hybrid. The XRD patterns for the above mentioned samples are shown in Fig. 2. The pure silica nanoparticles had no diffraction peak except a broad hump approximately between 25 and 40° indicating the amorphous properties of silica. The silica supported ceria samples had main diffraction patterns at 2θ = 45°, 50°, 72°, 88°, 94°, 113° corresponding to (111), (200), (220), (311), (222) and (400) ceria reflections confirming the formation of cubic crystalline ceria nanoparticles.
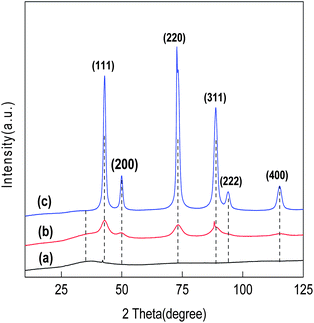 |
| Fig. 2 XRD diffraction analysis for (a) silica template, (b) silica–ceria hybrid and (c) unstabilized ceria. The primary crystallite size of ceria nanoparticles attached to silica was ∼3–5 nm. The silica particles before ceria attachment only showed a broad peak representing the amorphous nature of the particles. | |
The silica supported ceria diffraction peak was broadened compared to unsupported ceria samples indicating the small crystallite size of the as formed ceria. The crystallite size was calculated from the peak broadening using the Debye–Scherrer equation.25 The average ceria crystallite size for unsupported ceria and silica supported ceria was calculated be 8 nm and 5 nm respectively. Absence of any other noticeable trace peaks for the formation of additional complexes was evident from the XRD analysis.
Formation mechanism of the silica–ceria hybrid nanostructure. The nucleation and growth of nano-crystalline ceria particles on the surface of amorphous silica template particles were further investigated by pre-treatment of silica nanoparticles. To elucidate the mechanism of ceria growth on the silica surface via the active functional groups (–OH and Si–O–H), the silica sol nanoparticles were isolated by centrifugation from the sol–gel synthesized liquid and were pre-treated by heating @ ∼60–80 °C for 7 h and drying at 35 °C overnight. The synthesis of silica–ceria hybrid nanostructure procedure was followed as mentioned in the experimental section using the pre-treated silica as templates for growth of nano-crystalline ceria. The XRD analysis of the resultant product showed no distinguishable diffraction peaks for crystalline cerium oxide, and that the material is apparently X-ray amorphous (Fig. 3(a)). It is possible that the concentration or type of silanol groups (non-hydrogen bonded or H-bonded) in silica particles was decreased or modified with thermal treatment due to the condensation of silanol functional groups to form a siloxane bond.26 However utilizing the as prepared silica sol, without any thermal treatment, at neutral or acidic pH provides negatively surface charged Si–OH groups which acts as active binding sites for increased local concentration of Ce 3+ cations directly on the silica surface. Furthermore the addition of urea acts as a base catalyst which upon mild heat treatment at 85 °C decomposes to ammonia which increases the base acidity of the silica sol and the nucleation threshold for growth of Ce 3+ ceria cations directly on the silica surface. The formation of cerium oxide might have occurred with complexion with –OH groups on the silica surface to form intermediate hydroxide and to the final oxide phase. Our TEM image analysis further confirms our assumption of the role of surface silica functional groups on the growth of nano-crystalline ceria. Fig. 3(b) shows the electron microscopy image of same product analyzed by XRD (Fig. 3(a)). Several regions of image showed particles of irregular morphology and size exceeding 100 nm. From the TEM measurements it can be interpreted as nucleation and growth of many small numbers of crystallites of ceria within a nano-crystalline material which lacks long range order. This observation further confirms our assumption that with the reduction of silica surface functional groups by thermal treatment, the ability of the ceria precursor to form a chemical bond linkage with the silica surface is reduced. This is due to the decomposition of urea to ammonia and carbonate species in DI water above 80 °C which reacts with cerium nitrate metal salts to form the particle like features of irregular size and shape within the silica matrix.27 Our XPS surface analysis discussed in the later sections further strengthens the proposed mechanism of silica–ceria bond formation.
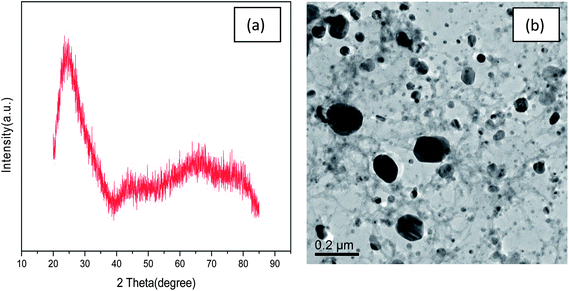 |
| Fig. 3 XRD diffraction analysis for silica–ceria hybrid control samples synthesized via thermal pre-treatment of the silica template which appears to be (a) apparently X-ray amorphous, and (b) non-homogenous in size and shape. | |
3.3. Morphology and structure analysis
The morphology and structure of unsupported ceria, silica and the silica–ceria hybrid nanostructure were analyzed by electron microscopy analysis. For TEM analysis the unsupported ceria and silica–ceria dry powder was dispersed in DI water and applied to TEM grids as described in the materials and methods section. The unsupported ceria nanoparticles (control) looked very irregular in shape and size (Fig. S1†). The surface morphology of silica particles was evaluated before and after ceria coating. Fig. 4(a) and (b) shows the TEM image of amorphous silica particles which are spherical and monodisperse and of median size ∼55 to 60 nm consistent with DLS size analysis measurements. Fig. 4(c) shows an image of silica nanoparticles after ceria nanoparticles coating. The image reveals darker regions representing ceria within the silica spheres which appear lighter in contrast. The ceria nanoparticles were distributed all over the silica nanoparticles surface in a more regular pattern than those obtained by calcination at higher temperature which leads only to non-homogenous coating and aggregation of ceria on the silica surface.20 Fig. 4(d) shows a high magnification image of a single silica particle after attaching ceria. The image reveals a dense surface coverage of ceria nanoparticles on the silica surface. The lattice fringes seen in the TEM images can be attributed to nano-crystalline ceria particles distributed on the surface of amorphous silica particles (Fig. 4(d) inset). The measurement of the lattice fringes distance substantiates the formation of cubic crystalline ceria nanoparticles on the silica surface. The size distribution of ceria nanoparticles attached to the silica surface was measured in the size range of 3–5 nm consistent with XRD crystallite size analysis. While most of the ceria particles are bound to the surface of silica, the presence of unbound ceria nanoparticles was also observed in small proportions possibly due to nucleation and growth of isolated ceria crystallites in solution. Fig. 4(e) and (f) show the SEM image/EDX analysis of silica–ceria which appears nearly spherical in shape and size. The EDX analysis on the marked regions of SEM images showed characteristic X-ray energies for O Lα1, Si Kα1, and Ce Lα1 confirming the presence of ceria within the silica matrix. All the above observations suggest that by utilizing sol–gel derived silica as a guest template it is possible to synthesize cerium oxide particles with a high surface area to volume ratio. Similarly Khalil et al. reported the synthesis of Fe2O3/SiO2 nanocomposites by hydrolysis of iron(III) nitrate in sols of silica particles and our results are in agreement with their findings where sol–gel derived silica particles sol with surface active functional groups can serve as anchor sites for nucleation and growth of oxide nanoparticles.28
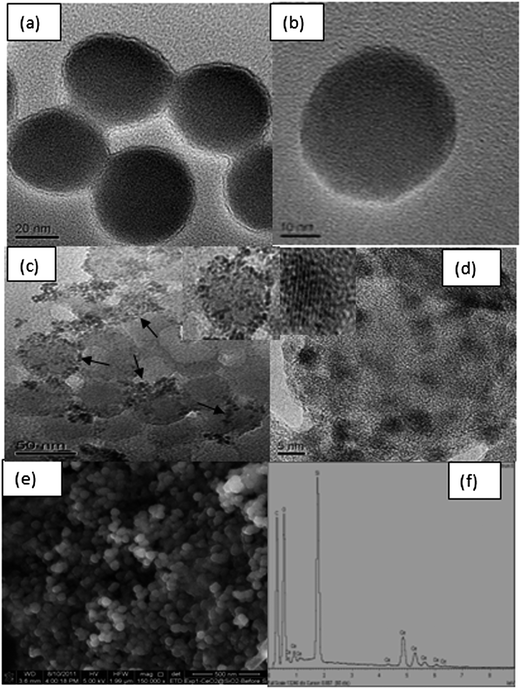 |
| Fig. 4 (a, b) TEM image of silica nanoparticle templates. (c) Silica nanoparticle after ceria coating marked by arrows (inset: silica nanoparticle appears bright due to low atomic number covered by dark spots of ceria). (d) Image of single silica particle after ceria coating. Ceria particles are of uniform size and morphology with visible lattice fringes confirming the formation of nano-crystalline ceria. SEM image and EDX chemical analysis of the silica–ceria hybrid nanostructure (e and f). | |
3.4. Nature of the bonding between silica and ceria
The chemical state of ceria nanoparticles and chemical bonding information between silica and ceria was characterized by XPS, a surface sensitive technique. Fig. 5(a) shows the XPS 3d spectral region for the silica–ceria hybrid nanostructure. The spectral features of silica supported ceria are consistent with the unsupported ceria samples control which substantiates the formation of cerium oxide. Assignment of the XPS peaks indicates the presence of ceria in mixed oxidation states (3+ and 4+). The ceria particles in the nano-size range, on an average less than 5 nm, exist in a mixed oxidation state. The features observed at 880.60 eV, 885.80 eV and 903.9 eV can be attributed to ceria in the 3+ oxidation state. The presence of 3+ states can be ascribed to oxygen vacancies in the atomic crystal structure.5 We further probed the chemical bonding characteristics between silica and ceria particles. The high resolution O 1s and Si 2p spectra for bare silica and ceria attached silica are shown in Fig. 5(a) right and (b). Comparing Si 2p and O 1s spectra for silica particles before and after attaching ceria, three main characteristic changes were observed: (i) the shift in the binding energy of both Si 2p and O 1s towards lower binding energy of 0.2 eV, (ii) the difference in full width at half maximum (broadening) of the peaks was calculated to be 1.7–2 eV (charge reference was made based on C 1s), (iii) compared to uncoated silica a shoulder peak at ∼530 eV was observed after ceria coating.
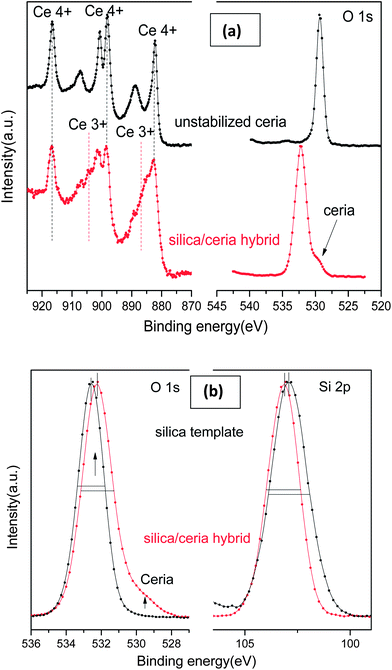 |
| Fig. 5 Comparison of XPS high resolution spectra between control ceria, silica template, and silica–ceria hybrid nanostructure (a) Ce 3d and O 1s spectra for control ceria and silica–ceria. (b) O 1s and Si 2p spectra of silica and silica–ceria nanostructure. | |
The shift in binding energy and peak broadening for silica–ceria represents the modified local surface chemical environment of silica nanoparticles. The negative shift in the binding energy of Si 2p and O 1s after ceria coating represents an increase in electron density on the surface of Si and surrounding O, which infers that this region of Si was covered by Ce. Furthermore the O 1s peak at ∼533 eV belongs to silica nanoparticles and a shoulder at ∼530 eV for ceria attached silica nanostructures represents Ce 3+ resulting from the formation of Si–O–Ce bond on the surface of silica particles.
To characterize the dependence of ceria nanoparticles formation on the surface silanol groups of silica, we performed solid state FTIR spectroscopic analysis of the silica particles template prior to and after ceria deposition (Fig. 6). Prior to ceria deposition, the structural Si–O–Si and Si–OH bands for silica were observed. The broad absorption band observed between at 3730 to 2550 cm−1 and 1630 cm−1 can be assigned to the stretching (ν) and bending (δ) modes of adsorbed water molecules and H-bonded silanols.29 The structural silica bands νas (Si–O–Si) are observed at 1200 and 1090 cm−1. The band at 950 cm−1 corresponds to ν (Si–OH) and SiO− groups.30 Similarly the band at 800 cm−1 and 470 cm−1 can be assigned to νs (Si–O–Si) and δ (Si–O–Si) mode.29,30 The silica–ceria nanostructure has similar groups of vibrational bands with few definite characteristic changes in the silanol bonds of silica. The Ce–O stretching bands are expected to be ca. 400 to 450 cm−1 and not clearly distinguishable from silica stretching modes (Si–O–Si) which occur in the same region.31 As such the absorption bands at 950 cm−1 due to dangling bands of oxygen atoms are reduced in intensity and vibration modes of the Si–O–Si siloxane bonds at 1090 cm−1 and 1200 cm−1 are shifted. This can be attributed to attachment of electropositive cerium ions to highly electronegative oxygen of silica nanoparticles.32
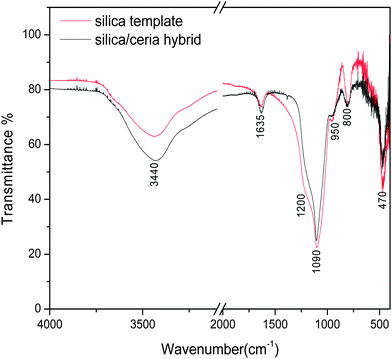 |
| Fig. 6 FTIR spectra of the silica nanoparticles template before and after attachment of ceria nanoparticles. | |
All the above observations indicate that ceria deposition on the silica nanoparticle surface takes place via growth of ceria on the silica nanoparticle surface. The proposed mechanism of cerium oxide nanocrystals growth could have occurred with the formation of covalent bond linkage between ceria and silica forming cerium–silicate bond. Our proposed mechanism is supported by the experimental observation of color change of the stirring reaction solution from colorless to murky white (hydroxide) to yellowish (oxide).
3.5. Agglomeration kinetics and sedimentation stability of silica–ceria nanoparticles in biological media conditions
For biomedical applications nanoparticles have to be dispersed in aqueous biological media conditions and stability of nanoparticles, surface modified or not, is most often affected by constituents and media conditions. Hence we examined the stability of silica–ceria hybrid by measuring the change in mean particle size in different biological media conditions (Fig. 7). The particle size measurements were started immediately following dispersion of particles in different media suspension. We found that silica–ceria hybrid particles remained stable in all of the media with minimal agglomeration indicated by a minor increase in particle size. In contrast unsupported ceria (control) showed much poorer dispersion in all of the tested media. The particle size varied based on type of medium, ionic strength of media, where particles agglomerated to form small to very large aggregates in the micron size range. The increase in aggregate size of unstabilized ceria particles in tested dispersion media can be explained based on the Brownian agglomeration mechanism where intrinsic surface charge of small ceria particles in high ionic strength media, like PBS, will be neutralized which leads to a reduced surface charge barrier and the chances of particle random collision and sticking together is increased. By attachment of small particles like ceria to larger silica particles the diffusivity of the particles will be less by which random collision between particles will be possibly reduced. Moreover the intrinsic surface property of silica, negative surface charge, provides enough surface screening, electrostatic repulsion, by which particles remain in solution and dispersion properties are improved. Though the concentration of ceria nanoparticles may be different between unsupported and silica–ceria the high agglomeration of unsupported ceria particles was observed even at very low concentrations indicating the instability of these systems under all of the measurement conditions.
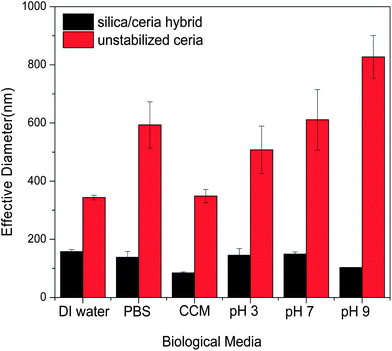 |
| Fig. 7 Average particle size of silica–ceria hybrid nanostructures in different pH conditions, PBS and CCM. | |
The agglomeration of nanoparticles in solution can affect the “life time” stability of nanoparticles in solution which in turn can affect the dosimetry for in vitro biological cell uptake experiments. To further evaluate the long term colloidal stability of silica supported ceria nanoparticles we performed time resolved DLS particle size measurements and characterized the suspension stability of silica–ceria nanoparticles by sedimentation stability analysis (Fig. 8(a) and (b)). The agglomeration kinetic studies indicate no change in average agglomerate particles size for the silica–ceria hybrid over a period of 2 h. However based on type of media used little change in initial particle size was observed and steady state was maintained throughout the end of the experiment. By comparison the unstabilized small ceria nanoparticles steeply increased in size purposefully in PBS media. This observation further confirms our Brownian agglomeration mechanism for smaller sized ceria nanoparticles due to surface charge neutralization in high ionic strength solutions. Our proposed hybrid nanostructure was successfully shown to overcome the higher diffusivity of small particles by attaching to the bigger support matrix by which rapid continuous movement of nanosize particles can be greatly reduced. Fig. 8(b) shows the change in the concentration of particles, “life time stability” measured by UV-Vis spectroscopic analysis. The cerium oxide nanoparticles have optical absorbance in the UV region centered on 300 and 325 nm. The settling rate was calculated by monitoring changes in absorbance intensity of the ceria nanoparticles suspension as a function of time. In agreement with dispersion results the silica supported ceria nanoparticles exhibited a high level of stability without any sign of rapid settling in all of the tested suspension media over the measurement time period. However after a period of 6 h the settling rate of the silica–ceria hybrid was found to increase with the final concentration of particles measured to be ∼80%. This is understandable considering the gravitation sedimentation which is size dependent. However the control unsupported ceria particles sediment out of the solution (PBS) at a much faster rate due to the high degree of agglomeration with more than 60% of the particles sedimenting out within the first 3 h of measurement duration. Considering the rate of settling and our previous time dependent agglomeration studies it is possible that the smaller unstabilized particles are predominantly dominated by both Brownian motion and gravitational agglomeration while as shown in Fig. 8(a) within few minutes (<2 min) the initial agglomerate size of particles was ∼500 nm. This size was found to increase followed by a correlative decrease in suspension stability that indicates that the small ceria particles are dominated initially by Brownian agglomeration and further by a gravitational collision mechanism. All of our observations indicated that similar sized ceria nanoparticles upon surface stabilization with larger silica nanoparticles exhibit higher colloidal stability by staying in suspension over a longer ageing period by overcoming random collision movement and gravitational agglomeration.
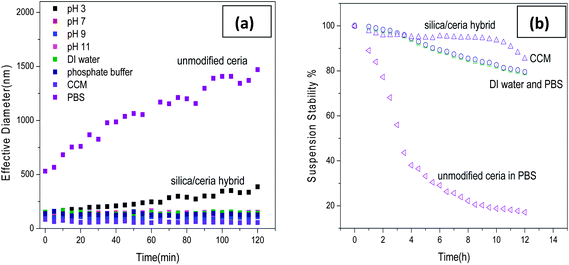 |
| Fig. 8 (a) Agglomeration kinetics of the silica–ceria hybrid in a variety of biological media commonly used for the dispersion of nanoparticles (b) suspension stability of silica–ceria hybrid measured as function of time in DI water, PBS and CCM for a period of 12 h. | |
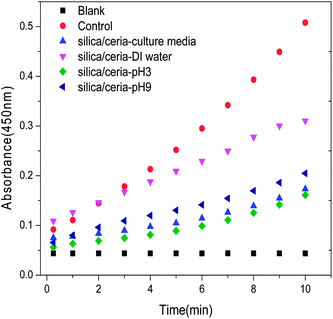 |
| Fig. 9 Analysis of bio-catalytic activity of silica supported ceria nanoparticles in biological relevant media conditions. | |
3.6. Analysis of surface reactivity of silica supported ceria nanoparticles in various media conditions by SOD mimetic assay
Recently the redox properties of nano-ceria have made them useful candidates as free radical scavengers and redox cycling antioxidants. The ceria atoms trivalent oxidation state (3+) at the surface of oxide nanoparticles can act as a reactive site that mimics SOD catalyst activity. However for biological testing, nanoparticles have to be dispersed in variety of cell cultures and physiological buffer conditions which contain small molecules, proteins, peptides, enzymes etc. which can limit the activity of small nanoparticles like ceria by surface screening effect or agglomeration. Hence we tested the superoxide radical scavenging ability of ceria which exists in 3+ oxidation state due to higher oxygen and electron vacancy as attached to silica templates. The silica–ceria nanostructures were incubated in the selected biological media DI water, CCM, and aqueous acidic and basic buffers (Fig. 9). For the free radical scavenging activity test, the silica–ceria nanostructure dispersed in biological media was tested along with cytochrome C control reagent. Experimentally the xanthine reagent gets converted to xanthine oxidase with free radical release which reacts with WST-1 reagent to form a colored product (formazan) which gives absorbance @ 450 nm. In comparison to the control reagent, the increase in absorbance was slow with inclusion of silica–ceria hybrids dispersed in different medias which indicated that the rate of catalytic activity or reaction of released free radical with WST-1 reagent is reduced in the case of silica–ceria inclusion as compared to the control reagent. This mechanism was consistently observed in all of the media in which silica–ceria was dispersed. Hence it is safe to say that compared to cytochrome C control the ceria nanoparticles reactive oxidation state (3+) is not limited by attachment to silica templates. Furthermore the silica–ceria hybrid nanostructure scavenging activity is as efficient as in any of the biological media conditions in which the catalytic activity was measured, and so is unlikely to be inhibited by the constituents present in solution.
4. Conclusion
The colloidal stability of unmodified cerium oxide nanoparticles of smaller sizes (<10 nm) can be improved by attaching to a bigger support matrix like silica nanoparticles. The surface silanol functional group of sol–gel derived silica nanoparticles was found to be essential in promoting the growth of ceria on the surface of amorphous silica. The silica–ceria hybrid nanostructures showed excellent stability against agglomeration and were characterized to be stable in suspension over longer ageing periods in different biological media conditions. The synthesized ceria nanoparticles which exist in 3+ oxidation states showed remarkable SOD mimetic activity in DI water, acidic/basic pH and culture media showing resistance to adverse biological media conditions. All the above mentioned characteristics of the silica–ceria hybrid nanostructure makes it a promising candidate for further in vitro and in vivo studies as a bio-catalytic material for oxidative stress related and other biomedical applications.
Acknowledgements
A portion of the research reported here was performed in EMSL, a DOE user facility supported by the Office of Biological and Environmental Research and located at Pacific Northwest National Laboratory. We thank Dr Don Baer for his critical review and useful insights of the manuscript. We acknowledge Dr Chongmin Wang for assistance with TEM and Dr Nachimuthu Ponnusamy for XPS characterization and analysis.
References
- K. A. Amin, M. S. Hassan, E. T. Awad and K. S. Hashem, Int. J. Nanomed., 2011, 6, 143–149 CrossRef CAS PubMed.
- A. Asati, C. Kaittanis, S. Santra and J. M. Perez, Anal. Chem., 2011, 83, 2547–2553 CrossRef CAS PubMed.
- I. Celardo, J. Z. Pedersen, E. Traversa and L. Ghibelli, Nanoscale, 2011, 3, 1411–1420 RSC.
- S. Das, S. Singh, S. Chigurupati, M. R. Mughal, A. Kumar, C. R. Patra, S. Oommen, N. E. Vlahakis, D. Mukhopadhyay, W. T. Self, M. P. Mattson and S. Seal, Wound Repair Regen., 2012, 20, A20 Search PubMed.
- S. Kar, C. Patel and S. Santra, J. Phys. Chem. C, 2009, 113, 4862–4867 CAS.
- S. Patil, S. Seal, Y. Guo, A. Schulte and J. Norwood, Appl. Phys. Lett., 2006, 88, 243110 CrossRef PubMed.
- S. Deshpande, S. Patil, S. V. N. T. Kuchibhatla and S. Seal, Appl. Phys. Lett., 2005, 87, 133113 CrossRef PubMed.
- A. S. Karakoti, P. Munusamy, K. Hostetler, V. Kodali, S. Kuchibhatla, G. Orr, J. G. Pounds, J. G. Teeguarden, B. D. Thrall and D. R. Baer, Surf. Interface Anal., 2012, 44, 882–889 CrossRef CAS PubMed.
- D. Baer, J. Vac. Sci. Technol., A, 2013, 31, 050820–050821 Search PubMed.
- Z. E. Allouni, M. R. Cimpan, P. J. Hol, T. Skodvin and N. R. Gjerdet, Colloids Surf., B, 2009, 68, 83–87 CrossRef CAS PubMed.
- D. Yoon, D. Woo, J. H. Kim, M. K. Kim, T. Kim, E. S. Hwang and S. Baik, J. Nanopart. Res., 2011, 13, 2543–2551 CrossRef CAS.
- X. Wang, T. A. Xia, S. A. Ntim, Z. X. Ji, S. George, H. A. Meng, H. Y. Zhang, V. Castranova, S. Mitra and A. E. Nel, ACS Nano, 2010, 4, 7241–7252 CrossRef CAS PubMed.
- S. V. N. T. Kuchibhata, A. S. Karakoti, D. R. Baer, S. Samudrala, M. H. Engelhard, J. E. Amonette, S. Thevuthasan and S. Seal, J. Phys. Chem. C, 2012, 116, 14108–14114 Search PubMed.
- A. H. Lu, E. L. Salabas and F. Schuth, Angew. Chem., Int. Ed., 2007, 46, 1222–1244 CrossRef CAS PubMed.
- I. Slowing, B. G. Trewyn and V. S. Lin, J. Am. Chem. Soc., 2006, 128, 14792–14793 CrossRef CAS PubMed.
- Y. I. Chung, J. C. Kim, Y. H. Kim, G. Tae, S. Y. Lee, K. Kim and I. C. Kwon, J. Controlled Release, 2010, 143, 374–382 CrossRef CAS PubMed.
- D. Farrell, S. A. Majetich and J. P. Wilcoxon, J. Phys. Chem. B, 2003, 107, 11022–11030 CrossRef CAS.
- T. Akagi, M. Higashi, T. Kaneko, T. Kida and M. Akashi, Macromol. Biosci., 2005, 5, 598–602 CrossRef CAS PubMed.
- Z. F. Zhang, L. Yu, W. L. Liu and Z. T. Song, Appl. Surf. Sci., 2010, 256, 3856–3861 CrossRef CAS PubMed.
- X. L. Song, N. Jiang, Y. K. Li, D. Y. Xu and G. Z. Qiu, Mater. Chem. Phys., 2008, 110, 128–135 CrossRef CAS PubMed.
- S. H. Lee, Z. Y. Lu, S. V. Babu and E. Matijevic, J. Mater. Res., 2002, 17, 2744–2749 CrossRef CAS.
- S. H. Chung, D. W. Lee, M. S. Kim and K. Y. Lee, J. Colloid Interface Sci., 2011, 355, 70–75 CrossRef CAS PubMed.
- H. Hiramatsu and F. E. Osterloh, Langmuir, 2003, 19, 7003–7011 CrossRef CAS.
- V. Mahalingam, M. Q. Tan, P. Munusamy, J. B. Gilroy, M. Raudsepp and F. C. J. M. van Veggel, Adv. Funct. Mater., 2007, 17, 3462–3469 CrossRef CAS.
- A. L. Patterson, Phys. Rev., 1939, 56, 978–982 CrossRef CAS.
- N. C. Strandwitz and G. D. Stucky, Chem. Mater., 2009, 21, 4577–4582 CrossRef CAS.
- P. L. Chen and I. W. Chen, J. Am. Ceram. Soc., 1993, 76, 1577–1583 CrossRef CAS.
- K. M. S. Khalil, H. A. Mahmoud and T. T. Ali, Langmuir, 2008, 24, 1037–1043 CrossRef CAS PubMed.
- C. A. R. Costa, C. A. P. Leite and F. Galembeck, J. Phys. Chem. B, 2003, 107, 4747–4755 CrossRef CAS.
- M. C. Matos, L. M. Ilharco and R. M. Almeida, J. Non-Cryst. Solids, 1992, 147, 232–237 CrossRef.
- C. M. Ho, J. C. Yu, T. Kwong, A. C. Mak and S. Y. Lai, Chem. Mater., 2005, 17, 4514–4522 CrossRef CAS.
- A. Singh and C. A. Hogarth, J. Mater. Sci., 1988, 23, 1090–1097 CrossRef CAS.
Footnote |
† Electronic supplementary information (ESI) available. See DOI: 10.1039/c3ra44345k |
|
This journal is © The Royal Society of Chemistry 2014 |