DOI:
10.1039/C3RA45859H
(Paper)
RSC Adv., 2014,
4, 8415-8420
Synthesis and characterization of a highly stable poly (3,4-ethylenedioxythiophene)-gold nanoparticles composite film and its application to electrochemical dopamine sensors†
Received
16th October 2013
, Accepted 13th January 2014
First published on 14th January 2014
Abstract
In this work, a highly stable nanocomposite film based on embedding gold nanoparticles (nanoAus) into a poly(3,4-ethylenedioxythiophene) (PEDOT) modified Pt electrode was fabricated and explored for dopamine sensing. The PEDOT film was synthesized in ionic liquids electrolyte-1-butyl-3-methylimidazolium tetrafluoroborate ([bmim][BF4]). Citrate coated nanoAus were immobilized on the polymer matrix via electrostatic interactions to facilitate the mass transport and weaken the capacitive current. This modified electrode took advantage of the high stability and excellent permselectivity of PEDOT, and exhibited a wide linear response to dopamine from 6.0 × 10−6 to 0.013 M. The detection limit was 0.2 μM (s/n = 3) and the amperometric response time was 2.5 s with chronoamperometry. Over the 8 months period of this study, the nanocomposite modified electrode still retained 85% of the original current response to dopamine. The highly stable modified electrode with improved sensitivity and selectivity could provide an ideal matrix for commercial applications.
1. Introduction
Inclusion of noble metallic functionalities inside conducting polymer matrix has attracted significant academic and technological attention and these materials have been widely used as recognition elements for the development of electrochemical sensors.1–4 The combination of such two materials into a composite may result in an enhancement of their original properties by overlapping their electronic or catalytic properties. Additionally, incorporating metallic nanoparticles into a conducting polymer can weaken the capacitive current induced by porous film.1
Among the numerous conducting polymers, poly (3,4-ethylenedioxythiophene) (PEDOT) has emerged as one of the most extensively studied materials and it holds potential applications in electronics, optical devices and sensors.5,6 Nevertheless, the relatively poor stability of conducting polymer is currently a bottleneck to its commercial applications. It has been demonstrated that the nature of dopant and electrolyte would strongly influence the electrochemical and physical properties of polymer during the polymeric process.7,8 Based on this viewpoint, different methods have been applied to increase its stability.9–11 Recent researches have reported that using ionic liquids as electrolytes, in which both the cation and the anion of the ionic liquids form an integral part of the polymer matrix,12,13 is extremely beneficial in improving the electrochemical efficiency and lifetime of conducting polymers. Mattes et al. reviewed the behavior of PEDOT as electrochemical mechanical actuators.14 When cycled in environmentally stable ionic liquids, PEDOT obtained an enhanced lifetime (up to 1 million cycles). Our previous work indicated that PEDOT15 synthesized in [bmim][BF4] displayed higher capacitive current and longer lifetime than that synthesized in traditional organic electrolyte. In this experiment, environmentally stable ionic liquid, 1-butyl-3-methylimidazolium tetrafluoroborate ([bmim][BF4]) was used as electrolyte to synthesize stable PEDOT film.
Dopamine, one of the most significant neurotransmitters in biological organisms,16 plays an important role in message transfer. The rapid and accurate determination of dopamine will influence the diagnosis in neurological disorder, such as Parkinsonism and schizophrenia.17 However, dopamine has close oxidation potential with some interference, which can cause poor selectivity and sensitivity in its determination.18,19 To overcome these problems, different kinds of electrochemical sensors have been prepared and applied for dopamine detection.20–24 Whereas, a simple dopamine sensor with sufficiently high stability and enhanced sensitivity for the commercial application, has not been reported. Therefore, the combination of PEDOT and gold nanoparticles (nanoAus), whose catalytic properties are well known,25,26 has been proposed for achieving a dopamine sensor with higher sensitivity and stability.
In this work, the electrochemical performance of the nanoAus/PEDOT nanocomposite material, when fabricated by successively electropolymerizing PEDOT in ionic liquid and incorporating the inert high-surface-area material of nanoAus, has been investigated for the oxidation of dopamine. The nanocomposite based sensor possessed a wide linear range and low detection limit towards dopamine detection. It was notable that it had a high stability of about 8 months.
2. Experimental section
2.1 Chemicals and apparatus
[bmim][BF4] was prepared using a previously reported method and purified by constant potential electrolysis.15 3,4-Ethylenedioxythiophene (EDOT) (Bayer AG more than 97%), dopamine (Sigma-Aldrich), gold chloride tetrahydrate (Shanghai Regent 1st Factory) were used as received. The other chemicals were of analytical reagent grade. Aqueous solutions were prepared with ultrapure water (>18 MΩ cm) obtained from a Milli-Q Plus system (Millipore).
Electrochemical measurements, such as cyclic voltammetry (CV), differential pulse voltammetry (DPV) and chronoamperometry were performed on a CHI 660B workstation (Shanghai Chenhua, Shanghai, China) with a conventional three-electrode system comprised of a platinum wire as the auxiliary, a saturated calomel electrode as the reference and the Pt disk electrode (1 mm diameter) as the working electrode. The morphologies of the samples were investigated using a Philips XL30S field emission scanning electron microscope (SEM) and the X-ray scatting experiments were carried out on an X-ray diffraction instrument (XRD, Micscience M18XHF with a Cu KR radiation).
2.2 Construction of nanoAus/PEDOT modified electrode
NanoAus solution with a mean size of 16 nm was prepared by reducing gold chloride tetrahydrate with citric acid at 100 °C for half an hour.27
Prior to modification, the Pt disk electrode was first polished with alumina slurry and sonicated sequentially in ethanol and ultrapure water in turn. After sonication, CV measurement was employed to deposit PEDOT onto the Pt disk electrode by potential scanning from −0.6 to 1.6 V (vs. Ag wire pseudo-reference electrode) for 8 cycles in [bmim][BF4] containing 0.1 M EDOT. After being rinsed thoroughly by acetonitrile, the resulted PEDOT film was immersed into the prepared nanoAus solution for 10 h at 4 °C, and then washed with phosphate buffer solution (PBS, pH 7.4) and stored at 4 °C.
2.3 Dopamine sensing on modified electrodes
Electrochemical measurements to characterize modified electrodes were performed in 0.1 M PBS (pH 7.0) by using different electrochemical techniques, including CV, DPV, and chronoamperometry. The CVs were recorded from −0.4 to 0.8 V at the scan rate of 50 mV s−1 after the addition of dopamine. DPV experiments were performed from −0.3 V to 0.5 V with an amplitude of 50 mV and the pulse period of 0.2 s. The chronoamperometry were carried out in 0.1 M PBS (pH 7.0) by applying the working potential of +0.35 V and allowing the transient current to decay to a steady-state value prior to the addition of the dopamine and the subsequent current monitoring. Freshly prepared solutions of dopamine and ascorbic acid were used for all the experiments. All experiments were carried out at room temperature (25 °C). Electrolyte solutions were deoxygenated before and during measurements.
3. Results and discussion
3.1 Optimization of the nanoAus/PEDOT modified electrode preparation conditions
The monomer concentration in the polymerization process would affect the thickness of the deposit, which would further affect the electrochemical behavior of the sensor. To evaluate the effect of monomer concentration on the response of nanoAus/PEDOT modified electrode to dopamine, PEDOT was synthesized in [bmim][BF4] with varying monomer concentrations from 0.01 M to 0.3 M. The response of the nanocomposite film to dopamine increased with an increase of monomer concentration up to 0.1 M (Fig. 1A), then the further increase of monomer concentration led to a decrease of response current. Therefore, the optimum monomer concentration was 0.1 M.
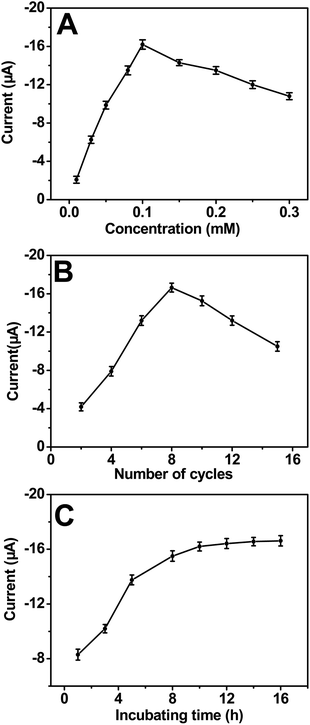 |
| Fig. 1 Effect of the (A) monomer concentration; (B) number of electropolymerization cycles; and (C) incubating time in nanoAus solutions on the nanoAus/PEDOT modified electrode response to dopamine. The current response was measured through the CV in pH 7.0 PBS with 1.0 mM dopamine. | |
The optimum number of CV cycles used to form the polymer film on electrode surface was investigated. The number of cycles during the electropolymerization was found to affect the sensitivity of the sensor. The nanoAus/PEDOT produced with more cycles owed thicker sensing films with less accessible catalytic active sites (Fig. 1B). The response of the nanocomposite film to dopamine increased with increasing the number of cycles up to 8 and leveled off when more cycles were used. Therefore, 8 cycles was chosen as the optimum polymerization cycles.
The time of embedding in nanoAus solution was also a key factor. The peak current increased along with increasing the embedding time, and tended to a plateau until 10 h (Fig. 1C). Consequently, the optimum embedding time was 10 h.
3.2 Characterizations of modified electrode
The PEDOT film was electropolymerized onto Pt electrode surface by cycling from −0.6 to 1.6 V (vs. Ag wire) in ionic liquid containing 0.1 M EDOT at a scan rate of 50 mV s−1 (Fig. 2A). From the CV shown in Fig. 2A (curve a), it was observed that EDOT oxidation started at 1.15 V and further shifted toward more positive potentials in the subsequent scans. The currents increased for successive potential cycles, indicating the further increasing amount of the polymer deposit. In comparison with the CVs for Pt electrode in ionic liquid Fig. 2A (curve b), the current corresponding to PEDOT/Pt electrode was visible, implied the excellent conductivity and large specific area.
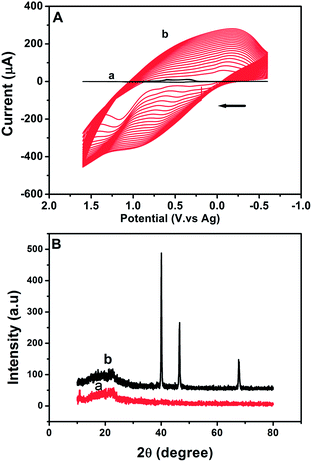 |
| Fig. 2 (A) Cyclic voltammetric behavior of bare Pt electrode in (a) neat [bmim][BF4] and (b) [bmim][BF4] containing 0.1 M EDOT at 50 mV s−1 and (B) XRD graphs of (a) PEDOT and (b) nanoAus/PEDOT. | |
Fig. 2B presented the XRD patterns of the PEDOT and nanoAus/PEDOT surface respectively. The wide diffraction peaks among 15° to 25° were attributed to the amorphous structure of PEDOT28 (Fig. 2B curve a). The diffraction peaks at 2θ = 40°, 46° and 68° could be indexed as the (111), (200) and (220) planes of Au respectively (Fig. 2B curve b). These results confirmed that the as-synthesized PEDOT polymer had an amorphous structure and combined with nanoAus uniformly.
The microstructures of PEDOT and nanoAus/PEDOT were tested by SEM. It was found that PEDOT film (Fig. 3A) exhibited a fibrillar network-like structure with the pore size from 50 to 100 nm, which enabled the PEDOT to have a much higher specific area. This network-like structure provided an open ion accessible structure, which was convenient to entrap the foreign material and yield a composite. Moreover, the permselective polymer membranes would create diffusion-limiting barriers to extend the linear measurement range. Some small bright particles with average diameter of 16 nm could be observed at the SEM image of nanoAus/PEDOT, which were evidence of the presence of nanoAus (Fig. 3B). The results of zeta potential showed that the polymer bore a net positive charge, thus citrate coated nanoAus were decorated onto the polymer matrix via electrostatic interactions. Besides, the covalent interaction of the unchelated Au–S bond was another interaction of the nanoAus bonding with PEDOT.29
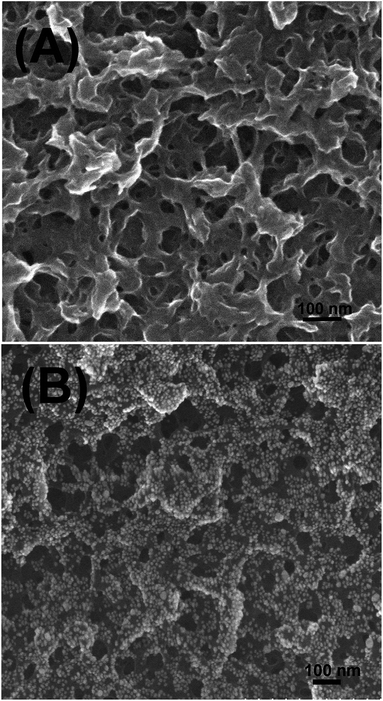 |
| Fig. 3 SEM images of (A) PEDOT and (B) nanoAus/PEDOT. | |
3.3 Oxidation of dopamine on nanoAus/PEDOT modified electrode
The voltammetric behavior of dopamine was examined by CV, where dopamine underwent the transfer of two electrons and two protons. Typical CVs of 1.5 mM dopamine in 0.1 M PBS on bare Pt electrode, PEDOT/Pt and nanoAus/PEDOT/Pt respectively were given in Fig. 4. The peak separation was found to be 0.274 V on bare Pt electrode, whereas it was 0.119 V on PEDOT/Pt and 0.096 V nanoAu/PEDOT/Pt, showing faster electron transfer kinetics on these two modified electrodes. The oxidation currents were about 3.0 fold on PEDOT and 4.88 fold on nanoAus/PEDOT/Pt than that on bare Pt electrode. It was notable that this increased ratio was obviously higher than the results obtained at the PEDOT modified electrode synthesized in traditional aqueous or organic electrolyte,1,30 indicating that the composite modified electrode possessed larger specific surface area and higher electron transfer rate. Moreover, the favorable weak adsorption of dopamine on nanoAus through the interaction of –NH2 with Au also enhanced the catalysis current.31
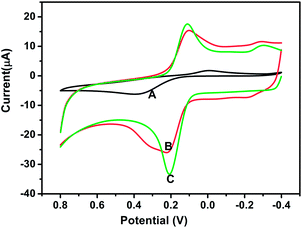 |
| Fig. 4 Cyclic voltammetric behaviors of 1.5 mM dopamine at: (A) bare Pt electrode, (B) PEDOT/Pt, and (C) nanoAus/PEDOT/Pt in 0.1 M PBS (pH 7.0) at 50 mV s−1. | |
Ascorbic acid coexisted with dopamine in biology media and they had similar oxidized potential at traditional electrodes. Therefore, the interference of ascorbic acid was a major obstacle in the electrochemical detection of dopamine. Researches had demonstrated that the electrostatic interaction could eliminate this interference.32 In this study, the nanoAus coated with citrate, which was in its anionic form at the working electrode surface, could attract dopamine molecules in their cationic form and repulsed the ascorbic acid in anionic form. Fig. 5 depicted the DPVs of standard additions of dopamine into 0.1 M pH 7.0 PBS solution containing 8 mM ascorbic acid. The electrochemical response of dopamine increased linearly with the dopamine concentration from 0.3 to 1.0 mM, while the peak currents of ascorbic acid kept unchanged, verifying that ascorbic acid did not present interference in the determination of dopamine.
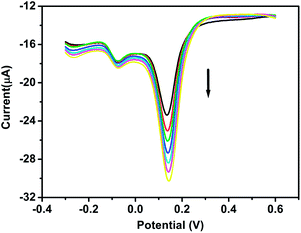 |
| Fig. 5 DPV of ascorbic acid and dopamine at the nanoAus/PEDOT/Pt in 0.1 M PBS (pH 7.0). The concentration of ascorbic acid is 8 mM and the concentration of dopamine was 0.3, 0.5, 0.6, 0.7, 0.8, 0.9 and 1.0 mM respectively. | |
3.4 Amperometric response of nanoAus/PEDOT coated electrode for dopamine
The amperometric response of nanoAus/PEDOT coated electrode with successive additions of dopamine to 0.1 M PBS (pH 7.0) at +0.35 V was illustrated in Fig. 6. Upon addition of dopamine, the response current increased steeply to reach a stable value within 3 s, indicating a fast amperometric response to dopamine oxidation. Such a rapid response could be attributed to the fast diffusion of the substrate in the porous and open frameworks of nanoAus/PEDOT film. The linear portion, which extended from 6.0 × 10−6 to 0.013 M with a correlation coefficient of 0.9997, was very wide and reproducible. The detection limit was estimated to be 0.2 μM at a signal-to-noise ratio of 3. When the concentration of dopamine was higher than 0.019 M, a response plateau was observed, implying a Michaelis–Menten kinetics model.
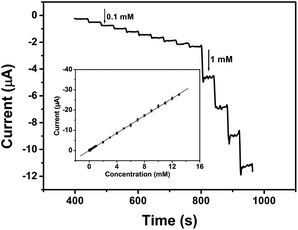 |
| Fig. 6 Typical amperometric current–time response curves of the sensor upon successive addition of dopamine into 0.1 M (pH 7.0) PBS at +0.35 V. Inset: linear plot of the amperometric response vs. dopamine concentration. | |
3.5 Reproducibility of nanoAus/PEDOT modified electrode
The reproducibility of the nanoAus/PEDOT modified electrode was investigated using a dopamine concentration of 1.0 mM and the relative standard deviation (RSD) was 1.2% for six successive assays. To evaluate the electrode-to-electrode reproducibility, six nanoAus/PEDOT electrodes were prepared under the same conditions independently. The RSD was 4.3%.
3.6 Analysis of dopamine in serum samples
The utilizations of the proposed method for the measurement of dopamine were studied by analyzing the serum samples containing known amount of dopamine. The serum samples were diluted 1
:
10 with 0.01 M PBS (pH 7.0) and then were detected. Then aliquots of the dopamine were added to the electrolyte solution and the current responses for dopamine were measured and the corresponding concentrations were estimated by the calibration graphs. The dopamine contents of the same samples were measured 6 times and the recovery was calculated to obtain the accuracy. The results were shown in the Table 1. The recovery ranged from 98.2% to 103% with RSD < 4.3%, indicating that the modified electrode were free from interferences of the serum sample matrix.
Table 1 Determination of dopamine in serum samples (n = 6)
Sample |
Dopamine added (mM) |
Dopamine found (mM) |
Recovery (%) |
RSD (%) |
1 |
0.0800 |
0.0816 |
102 |
4.3 |
2 |
0.500 |
0.493 |
98.6 |
3.8 |
3 |
0.700 |
0.719 |
103 |
2.6 |
4 |
1.00 |
0.982 |
98.2 |
4.0 |
5 |
8.00 |
8.15 |
102 |
3.1 |
3.7 Stability of the nanoAus/PEDOT modified electrode
The most remarkable characteristic of this device was the high operational stability, which was a very desirable parameter, particularly for in situ sensor applications. To characterize the nanocomposite film modified electrode stability, it was suspended at 4 °C in a refrigerator and periodically, the current response of nanoAus/PEDOT to 1.0 mM dopamine was measured in a pH 7.0 PBS solution at 25 °C. As shown in Fig. 7, the oxidation current did not decay at the initial days and it increased until 36th day. This should be associated with the porous structure along with a swelling of the material due to solvent and salt retention.33 The nanocomposite film maintained a similar response without obvious deactivation in the following four months, showing satisfactory long-term storage stability.34–36 After 240 days storage, it still retained 85% of its initial response and kept a linear range from 10 to 90 μM. On the contrary, the nanoAus/PEDOT modified electrodes that PEDOT were electropolymerized in acetonitrile electrolyte and aqueous solutions of a constant monomer concentration and polymeric quantity, lost nearly one half of their initial response signal after 3 months. Moreover, the nanoAus/PEDOT obtained in ionic liquid had higher sensitivity than that obtained in traditional organic or aqueous electrolyte. The good long-term stability and high sensitivity should be mainly attributed to the stable chemical and physical characterizations of PEDOT.
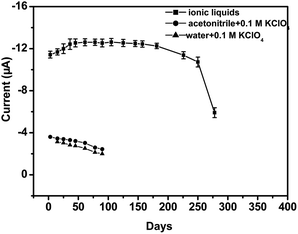 |
| Fig. 7 Stability tests of the different nanoAus/PEDOT modified electrodes. | |
4. Conclusions
In this work, a novel nanocomposite film based on nanoAus/PEDOT was assembled and applied in the determination of dopamine. The results indicated that the uniform three-dimensional porous structure of nanocomposite film provided a suitable matrix to facilitate the bioelectrocatalytic activity to dopamine with good stability of about 8 months. Moreover, the permselective film extended linear measurement range and quickened response time. To our knowledge, no previous attempt had been made to construct such a highly stable and sensible dopamine sensor based on nanoAus/PEDOT nanocomposite films. It is expected that this nanocomposite film could also be used to construct other new sensors. Therefore, the stable nanoAu/PEDOT nanocomposite suggested great potential in commercial applications.
Acknowledgements
This work was supported by the National Natural Science Foundation of China through the Young Program (21005034), the pre-research Foundation of Henan Academy of Sciences.
Notes and references
- S. S. Kumar, J. Mathiyarasu and K. L. Phani, J. Electroanal. Chem., 2005, 578, 95–103 CrossRef PubMed.
- F. H. Meng, W. Shi, Y. N. Sun, X. Zhu, G. S. Wu, C. Q. Ruan, X. Liu and D. T. Ge, Biosens. Bioelectron., 2013, 42, 141–147 CrossRef PubMed.
- M. Ates, Mater. Sci. Eng., C, 2013, 33, 1853–1859 CrossRef PubMed.
- L. C. Chang, H. N. Wu, C. Y. Lin, Y. H. Lai, C. W. Hu and K. C. Ho, Nanoscale Res. Lett., 2012, 7, 319–326 CrossRef PubMed.
-
K. Reuter, S. Kirchmeyer and A. Elschner, PEDOT–properties and technical relevance, in Handbook of the Thiophene-Based Materials: Applications in Organic Electronics and Photonics, ed. I. F. Perepichka and D. F. Perepichka, E-Publishing Inc., Chichester, 2009, pp. 549–576 Search PubMed.
-
J. C. Scott, History of conductive polymers, in Nanostructured conducting polymers, ed. A. Eftekhari, E-Publishing Inc., Chichester, 2010, pp. 1–17 Search PubMed.
- L. Li, D. C. Loveday, D. S. K. Mudigonda and J. P. Ferraris, J. Electrochem. Soc., 2002, 149, A1201–A1207 CrossRef PubMed.
- J. Tanguy, M. Slama, M. Hoclet and J. L. Baudouin, Synth. Met., 1989, 28, 145–150 CrossRef.
- T. A. Bendikov and T. C. Harmon, Anal. Chim. Acta, 2005, 551, 30–36 CrossRef PubMed.
- J. M. Nadeau and T. M. Swager, Tetrahedron, 2004, 60, 7141–7146 CrossRef PubMed.
- B. Winther-Jensen and K. West, React. Funct. Polym., 2006, 66, 479–483 CrossRef PubMed.
- G. A. Snook, P. Kao and A. S. Best, J. Power Sources, 2011, 196, 1–12 CrossRef PubMed.
- S. Ahmad, M. Deepa and S. Singh, Langmuir, 2007, 23, 11430–11433 CrossRef PubMed.
- W. Lu, A. G. Fadeev, B. Qi, E. Smela, B. R. Mattes, J. Ding, G. M. Spinks, J. Mazurkiewicz, D. Zhou, G. G. Wallace, D. R. MacFarlane, S. A. Forsyth and M. Forsyth, Science, 2002, 297, 983–987 CrossRef PubMed.
- K. K. Liu, Z. L. Hu, R. Xue and J. R. Zhang, J. Power Sources, 2008, 179, 858–862 CrossRef PubMed.
- N. F. Atta, A. Galal, F. M. A. Attia and S. M. Azab, J. Electrochem. Soc., 2010, 157, F116–F123 CrossRef PubMed.
- A. Spooren, P. Rondou, K. Debowska, B. Lintermans, L. Vermeulen, B. Samyn, K. Skieterska, G. Debyser, B. Devreese, P. Vanhoenacker, U. Wojda, G. Haegeman and K. V. Craenenbroeck, Cell. Signalling, 2010, 22, 600–609 CrossRef PubMed.
- K. S. Prasad, G. Muthuraman and J. M. Zen, Electrochem. Commun., 2008, 10, 559–563 CrossRef PubMed.
- F. J. Shang, L. Zhou, K. A. Mahmoud, S. Hrapovic, Y. Liu, H. A. Moynihan, J. D. Glennon and J. H. T. Luong, Anal. Chem., 2009, 81, 4089–4098 CrossRef PubMed.
- N. F. Atta, A. Galal and E. H. El-Ads, Electrochim. Acta, 2012, 69, 102–111 CrossRef PubMed.
- A. Celebanska, D. Tomaszewska, A. Lesniewski and M. Opallo, Biosens. Bioelectron., 2011, 26, 4417–4422 CrossRef PubMed.
- D. Lakshmi, A. Bossi, M. J. Whitcombe, I. Chianella, S. A. Fowler, S. Subrahmanyam, E. V. Piletska and S. A. Piletsky, Anal. Chem., 2009, 81, 3576–3584 CrossRef PubMed.
- X. W. Kan, H. Zhou, C. Li, A. H. Zhu, Z. L. Xing and Z. Zhao, Electrochim. Acta, 2012, 63, 69–75 CrossRef PubMed.
- M. Zheng, Y. Chen, Y. M. Zhou, Y. W. Tang and T. H. Lu, Talanta, 2010, 81, 1076–1080 CrossRef PubMed.
-
F. G. Bănică, Nanomaterial applications in chemical sensors, in Chemical Sensors and biosensors: Fundamentals and Applications, E-Publishing Inc., Chichester, 2012, pp. 135–156 Search PubMed.
- A. Merkoçi, Electroanalysis, 2013, 25, 15–27 CrossRef.
- B. V. Enustun and J. J. Turkevich, J. Am. Chem. Soc., 1963, 85, 3317–3328 CrossRef.
- J. W. Choi, M. G. Han, S. Y. Kim, S. G. Oh and S. S. Im, Synth. Met., 2004, 141, 293–299 CrossRef.
- J. Coetzee, W. F. Gabrielli, K. Coetzee, O. Schuster, S. D. Nogai, S. Cronje and H. G. Raubenheimer, Angew. Chem., Int. Ed., 2007, 46, 2497–2500 CrossRef PubMed.
- N. F. Atta, A. Galal and E. H. El-Ads, Electrochim. Acta, 2012, 69, 102–111 CrossRef PubMed.
- C. R. Raj, T. Okajima and T. Ohsaka, J. Electroanal. Chem., 2003, 543, 127–133 CrossRef.
- S. C. Fernandes, I. C. Vieira, R. A. Peralta and A. Neves, Electrochim. Acta, 2010, 55, 7152–7157 CrossRef PubMed.
- J. P. Ferraris, M. M. Eissa, I. D. Brotherston and D. C. Loveday, Chem. Mater., 1998, 10, 3528–3535 CrossRef.
- M. M. Liu, R. Liu and W. Chen, Biosens. Bioelectron., 2013, 45, 206–212 CrossRef PubMed.
- A. Kros, S. W. F. M. V. Hövell, N. A. J. M. Sommerdijk and R. J. M. Nolte, Adv. Mater., 2001, 13, 1555–1557 CrossRef.
- Y. L. Wang, B. H. Chu, C. Y. Chang, C. F. Lo, S. J. Pearton, A. Dabiran, P. P. Chow and F. Ren, Sens. Actuators, B, 2010, 146, 349–352 CrossRef PubMed.
Footnote |
† Electronic supplementary information (ESI) available. See DOI: 10.1039/c3ra45859h |
|
This journal is © The Royal Society of Chemistry 2014 |