DOI:
10.1039/C3PY00647F
(Paper)
Polym. Chem., 2014,
5, 144-152
Post-metalation of porous aromatic frameworks for highly efficient carbon capture from CO2 + N2 and CH4 + N2 mixtures†
Received
21st June 2013
, Accepted 22nd July 2013
First published on 24th July 2013
Abstract
The development of microporous materials for carbon capture, especially for carbon dioxide and methane, is a rapidly growing field based on the increasing demand for clean energy and pressing environmental concerns of global warming effected by greenhouse gases. To achieve this goal of developing carbon selective porous materials, a new porous aromatic framework featuring carboxyl-decorated pores, PAF-26-COOH, has been synthesized successfully. The modification of PAF-26 materials with representative light metals is exemplified by Li, Na, K and Mg via a post-metalation approach. The obtained PAF-26 products exhibit moderate surface area and controllable pore size at the atomic level. Gas sorption of CO2, CH4 and N2 is carried out on as-prepared PAF-26 samples at mild temperatures (273 K and 298 K). It is found that the PAF-26 materials show high adsorption capacity for CO2 and CH4 and low ability toward N2. Particularly, as-synthesized PAF-26 compounds exhibit remarkably high isosteric heats of adsorption toward CO2 and CH4, indicating high affinity for CO2 and CH4 gases. The gas selectivity for CO2–N2 and CH4–N2 mixtures is predicted by the IAST model. High selectivity of 80 for CO2 over N2 is obtained for PAF-26-COOMg. In addition, high selectivity values of CH4 over N2 are observed. The high performance including high storage capacity and selectivity makes PAF-26 materials promising for carbon capture or sequestration.
Introduction
One of the most pressing environment concerns of our age is the escalating level of atmospheric greenhouse gases (mainly CO2), which is deemed as a significant contribution to global warming.1 Flue gas emissions of power plants are responsible for roughly 30% of total CO2 emissions. Nitrogen is a main component (>70%) of flue gas whereas the major impurity is CO2 (10–15%); thus it is required to separate CO2 from N2.2 Another energy-related separation involves the purification of CH4 from natural gas, since natural gas is mainly composed of valuable methane, typically 80–95%, with N2 and CO2 impurities of 5–10% depending on different gas reservoirs. In addition, landfill gas and coal-bed gas are rapidly growing as complements for natural gas; however, they often contain unacceptable levels of N2 contaminants. The separation of methane from nitrogen is essential for upgradation of natural gas and purification of landfill and coal-bed gases to improve their purity in order to meet specific criteria. Thus, carbon capture and separation (CCS) is therefore highly demanded. Consequently, sorption-based techniques or processes (usually involving solid adsorbents) have been innovated for CCS, which plays a leading role among versatile approaches.3 Therefore, intensive efforts have been made to investigate the use of solid adsorbents for carbon capture and storage. In the library of solid adsorbents, porous materials, such as zeolites,4 carbons,5 mesoporous silica-supported amines,6 and metal organic frameworks,7 have exhibited good performances for practical CCS implementation. Very recently, porous organic frameworks (POFs) have also been proposed as new porous adsorbents for carbon capture and storage.8 POFs composed of light elements via robust covalent bonds are emerging as a new family of porous architectures and are attracting increasing attention thanks to their high stability, tunable building blocks, controlled pore connectivity, and featuring opportunities for further functionality.9 To achieve the goal of high-performance gas capture and storage with enhanced capacity and selectivity using POF materials, rational modification of POF skeletons with variable functionalities is deemed to be an appropriate approach.10 Zhou's group has reported that sulfonate-grafted and polyamine-tethered porous polymer networks exhibit exceptionally high binding affinity and selectivity for CO2.10a,11g Generally, gas storage in POFs is greatly related to the pore size and the polarity of the pore surface. Controlling the pore properties such as pore size and polarity in POFs is possible by changing the functional group in the organic monomer. Tailoring electrostatic interaction on the pore surface at the atomic level such as introducing different metal ions in the porous skeleton may be a possible way to fine-control the pore property. The incorporation of metal ions can introduce point charges into the host material framework. By changing the atomic numbers of the incorporated metals, we can tune the electrostatic charge-quadrupole and charge-induced dipole interactions with gas molecules on the pore surface.11 In this study, we report the design and synthesis of a carboxyl-functionalized porous aromatic framework (PAF) material with permanent porosity, PAF-26-COOH, and a series of light metalized derivatives, referred to as PAF-26-COOM (M = Li, Na, K, Mg) (Scheme 1). The resulting PAF-26-COOM materials are examined for CH4, CO2 and N2 sorption. Further, the effects of pore size, surface area and isosteric heat of adsorption on a group of PAF-26-COOM materials will be investigated in detail. Besides, the adsorption capacity and selectivity of these metalized PAF materials for CO2 and CH4 in CO2–N2 and CH4–N2 binary mixtures are estimated.
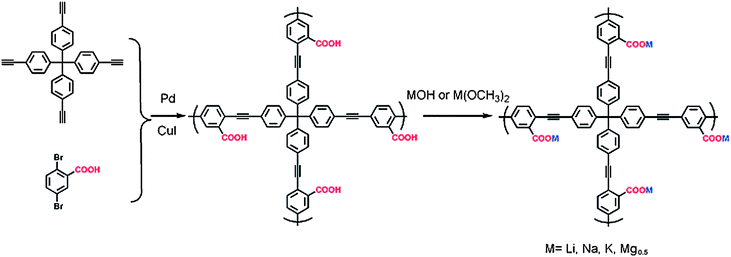 |
| Scheme 1 Schematic representation of the synthesis of PAF-26-COOH and PAF-26-COOM using tetrakis(4-ethynylphenyl)methane and 2,5-dibromobenzoic acid. | |
Experimental section
1 Chemicals
The chemicals were purchased from Aldrich, Alfa-Aesar, and Aladdin-reagent, and used as received unless it was noted. N,N-Dimethylformamide (DMF) and triethylamine (Et3N) were dehydrated with CaH2. Magnesium methoxide solution (7–8%) in methanol was purchased from Alfa-Aesar. Tetrakis(4-ethynylphenyl)methane was prepared according to the previously reported method.9f 2,5-Dibromo-benzoic acid was purchased from Aladdin-reagent.
2 Synthesis of PAF-26-COOH
PAF-26-COOH was synthesized via palladium-catalyzed Sonogashira–Hagihara cross-coupling reaction. Typically, tetrakis(4-ethynylphenyl)methane (250 mg, 0.6 mmol), 2,5-dibromobenzoic acid (335 mg, 1.2 mmol), tetrakis(triphenylphosphine)palladium (45 mg), and copper iodide (15 mg) were dissolved in a mixture of DMF (15 mL) and Et3N (15 mL) in a 50 mL two-neck flask. After degassing via three freeze–pump–thaw cycles, the mixture was stirred at 100 °C for 36 h under a N2 atmosphere. After cooling down to room temperature, the resulting PAF-26-COOH was collected by filtration, followed by consecutive washing with chloroform (10 mL), methanol (10 mL), and water (10 mL) to remove the unreacted monomers and catalysts. As-prepared PAF-26-COOH was further purified via Soxhlet extraction with methanol for 48 h. After drying at 90 °C in vacuum overnight, PAF-26-COOH was obtained in the form of a yellow powder (352 mg, 89% yield).
3 Post-metalation of PAF-26-COOH
3.1 Synthesis of PAF-26-COOLi, PAF-26-COONa and PAF-26-COOK.
10 mg LiOH was added to a mixture of PAF-26-COOH (100 mg) in CH3OH–H2O (10 mL/10 mL). The resulting mixture was stirred at room temperature for 1 day. Subsequently, the solid was collected by filtration, washed with water several times to completely remove LiOH residues, and dried to produce PAF-26-COOLi as a yellow powder (95 mg).
PAF-26-COONa and PAF-26-COOK were prepared by the same procedure using NaOH and KOH as alkaline sources, respectively.
3.2 Synthesis of PAF-26-COOMg.
Magnesium methoxide (7–8% in methanol solution) is considered as the suitable alkaline source to overcome the poor solubility of Mg(OH)2 in CH3OH or water. Typically, 210 μL magnesium methoxide solution was added to a mixture of PAF-26-COOH (100 mg) and anhydrous methanol (15 mL) in a dry 50 mL flask. The resulting mixture was then stirred in a dry atmosphere at room temperature for 12 h. Then, the solid was collected by filtration and washed with anhydrous methanol several times. After drying at 90 °C in vacuum for 10 h, PAF-26-COOMg was obtained as a yellow powder (92 mg).
4 Characterizations
FTIR spectra were obtained using an IFS 66V/S Fourier transform infrared spectrometer. Thermogravimetric analysis was implemented using a Netzch Sta 449c thermal analyzer system at a heating rate of 10 °C min−1 under air. Scanning electron microscopy (SEM) imaging was performed on a JEOS JSM 6700. X-ray diffraction (XRD) measurements were carried out on a Riguku D/MAX2550 diffractometer with Cu-Kα radiation (λ = 1.5418 Å) operating at a voltage of 50 kV and a current of 200 mA. Solid-state 13C CP/MAS NMR measurement was carried out on a Bruker Avance III model 400 MHz NMR spectrometer at a MAS rate of 5 kHz. The metal content in metalized porous frameworks was determined by inductively coupled plasma (ICP) spectroscopy (Perkin-Elmer ICP-OES Optima 3300DV). The typical procedure includes: (1) the digestion of samples (∼15 mg) in 3
:
1 HCl–HNO3 10 mL solution and heating at 120 °C in a 15 mL autoclave for 12 h; (2) after cooling down to room temperature, the solution was filtered and transferred to a 50 mL volumetric flask with distilled water.
5 Low-pressure gas adsorption measurements
The gas sorption isotherms were measured on a Quantachrome Autosorb iQ2 analyzer. Prior to the measurements, the samples were degassed at 120 °C for 24 h. N2 sorption isotherms were recorded at 77 K, 273 K, and 298 K; CH4 and CO2 sorption measurements were performed at 273 K and 298 K, respectively. Ultra-high-purity grade (99.99%) N2, CH4, and CO2 gases were used for all adsorption measurements. Liquid nitrogen baths were utilized to control the temperature at 77 K. Ice-water and water baths equipped with a temperature sensor were used to control the temperature at 273 K and 298 K.
Results and discussion
Description of PAF-26-COOH and PAF-26-COOM
To build a porous architecture, secondary building blocks with tetrahedral geometry are employed to form 3-D porous organic frameworks via their co-condensation with linear building blocks. Additionally, the functional groups on the building blocks and their connection modes determine the physicochemical properties of organic frameworks. Using this concept, PAF-26-COOH is prepared by the condensation of tetrakis(4-ethynylphenyl)methane as the tetrahedral node, and 2,5-dibromo-benzoic acid as the linear linker via Sonogashira–Hagihara cross-coupling reaction (Scheme 1).12 Further, PAF-26-COOH bearing free carboxylic groups in the skeleton is post-modified with different light metals, and the corresponding products are designated as PAF-26-COOM. For better illustration of the connectivity and geometry of carbon atoms in the PAF-26-COOH structure, this PAF material was characterized by solid-state 13C cross-polarization magic-angle spinning (CP/MAS) NMR and Fourier transform infrared (FTIR) spectroscopy. Fig. 1 shows the 13C CP/MAS NMR spectrum of a PAF-26-COOH solid sample. An NMR signal around 168 ppm is observed, which is assigned to the carbon atom in the carboxyl groups. This means that the carboxyl groups remain intact, which makes further modification possible. A well-resolved peak is detected at 64.8 ppm associated with the quaternary carbon atom in tetrakis(4-ethynylphenyl)methane, indicating that tetrakis(4-ethynylphenyl)methane is the main component in the final PAF-26-COOH product.13 A distinct NMR shift assigned to the benzene rings at 131.1 ppm occurs, which indicates that the PAF-26-COOH framework is composed of highly conjugated phenyl groups.
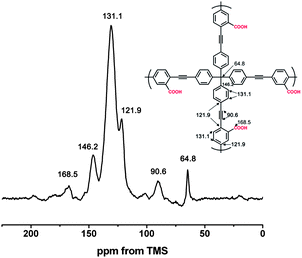 |
| Fig. 1
13C CP/MAS NMR spectrum of PAF-26-COOH. | |
The PAF-26-COOH sample is further studied by FT-IR spectroscopy. The IR spectra of as-prepared PAF-26-COOH and their starting monomers are shown in Fig. 2. The disappearance of the peak at 3283 cm−1 associated with
C–H in the PAF-26-COOH product (Fig. 2c) provides direct evidence for the completed cross-coupling reaction. The IR bands corresponding to the stretching vibrations of the –COO group (1728 and 3431 cm−1) in the PAF-26-COOH sample are similar to those of its monomer 2,5-dibromo-benzoic acid (Fig. 2a), which means that –COO groups are kept unchanged during the reaction. The band related to the C–Br vibration mode at 1153 cm−1 (Fig. 2a) vanishes into the background, which gives distinct proof for the complete reaction between these two monomers (Fig. 2c). The metalized derivatives PAF-26-COOM were also characterized by IR spectroscopy and their IR spectra are recorded in Fig. 3. Shoulder bands around 1683 cm−1, 1683 cm−1, 1681 cm−1 and 1685 cm−1 for PAF-26-COOLi, PAF-26-COONa, PAF-26-COOK and PAF-26-COOMg samples grow conjointly with the ones at 1722 cm−1, 1721 cm−1, 1720 cm−1, and 1724 cm−1, respectively. These additional bands are assigned to the –COO groups linked to metal cations, which are not observed in the case of PAF-26-COOH. The metal loading in PAF-26-COOM was analyzed by inductively coupled plasma optical emission spectroscopy (ICP-OES). It is found that the protons in the carboxyl groups of PAF-26-COOH are almost stoichiometrically substituted by metal ions (Table 1), affording the corresponding PAF-26-COOLi, PAF-26-COONa, PAF-26-COOK and PAF-26-COOMg products. This high replacement of protons (about 85%) confirms that nearly all the carboxyl groups in the PAF-26-COOH framework are available for post-metallization, which is in agreement with the IR result. The isolated PAF-26-COOH and its corresponding derivatives PAF-26-COOM powders were subjected to XRD and SEM characterizations. Based on the XRD study (Fig. S1†), all of them exist as amorphous polymers. Spherical aggregates with small particles in the size of 3–5 μm are observed in SEM pictures (Fig. S2†). The thermal stability of PAF26s is studied by TGA (Fig. S3†). A distinct weight loss in the range of 300–400 °C is observed, which corresponds to the decomposition or collapse of the organic skeleton.
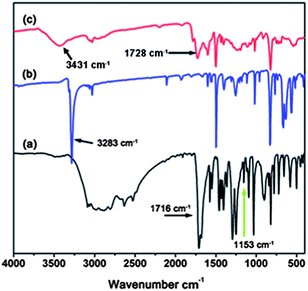 |
| Fig. 2 FT-IR spectra of starting materials 2,5-dibromobenzoic acid (a), tetrakis(4-ethynylphenyl)methane (b) and as-prepared PAF-26-COOH (c). | |
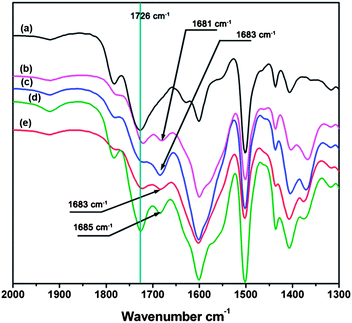 |
| Fig. 3 FT-IR spectra of (a) PAF-26-COOH, (b) PAF-26-COOK, (c) PAF-26-COONa, (d) PAF-26-COOMg, (e) PAF-26-COOLi. | |
Table 1 Results from chemical analysis (ICP) and N2-sorption measurements for PAF-26 samples including metal loadings, BET surface areas, pore volumes and their ratio, and pore sizes
Samples |
Theoretical metal loading (mmol g−1) |
Actual metal loadinga (mmol g−1) |
BET SSAb (m2 g−1) |
V
micro (cm3 g−1) |
V
total
(cm3 g−1) |
V
micro/Vtotal |
Pore sized (nm) |
The actual metal loading was analyzed by inductively coupled plasma optical emission spectroscopy (ICP-OES).
BET SSA was calculated in the partial pressure (p/p0) range of 0.01–0.05 which gives the best linearity.
Total pore volume at relative pressure p/p0 = 0.99.
The pore size distribution calculated using the DFT method.
|
PAF-26-COOH |
|
— |
717 |
0.31 |
0.36 |
0.86 |
0.52 |
PAF-26-COOLi |
3 |
2.45 |
591 |
0.24 |
0.28 |
0.86 |
0.52 |
PAF-26-COONa |
3 |
2.6 |
483 |
0.20 |
0.23 |
0.87 |
0.49 |
PAF-26-COOK |
3 |
2.4 |
430 |
0.19 |
0.20 |
0.95 |
0.48 |
PAF-26-COOMg |
1.5 |
1.3 |
572 |
0.23 |
0.28 |
0.82 |
0.57 |
Porosity of PAF-26-COOH and PAF-26-COOM
The porosity and pore structures of the PAF-26 series were investigated by nitrogen sorption measurement at 77 K. As shown in Fig. 4, a sharp increase in gas uptake is observed at low pressure in the nitrogen adsorption–desorption isotherms of PAF-26-COOH and PAF-26-COOM, which confirms the existence of micropores in PAFs. A weak hysteretic shoulder in the desorption isotherms proves that the materials also feature a small degree of mesoporosity. For clear illustration, a series of pore parameters derived from the nitrogen isotherms are listed in Table 1, including Brunauer–Emmett–Teller (BET) apparent surface area, pore size, total pore volume and micropore volume. The surface area of PAF-26-COOM is a bit lower than that of PAF-26-COOH (717 m2 g−1 for PAF-26-COOH, 591 m2 g−1 for PAF-26-COOLi, 483 m2 g−1 for PAF-26-COONa, 430 m2 g−1 for PAF-26-COOK and 572 m2 g−1 for PAF-26-COOMg, respectively). The replacement of hydrogen atoms with metal ions leads to a decrease of surface area, which is consistent with an increase of atomic weights from Li to K.
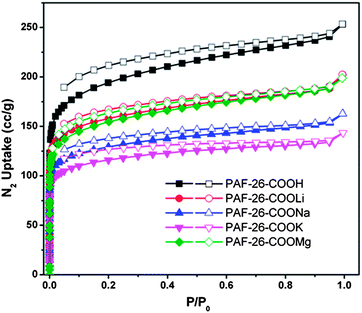 |
| Fig. 4 N2 adsorption–desorption isotherms measured at 77 K for PAF-26-COOH, PAF-26-COOLi, PAF-26-COONa, PAF-26-COOK and PAF-26-COOMg; the adsorption branch is denoted by closed symbols, and desorption branch by open symbols. | |
Based on the non-local density functional theory (NLDFT), the pore size distribution (PSD) of PAF-26-COOH exhibits a dominant pore diameter of 0.52 nm, while the PSD of PAF-26-COOM exhibits dominant ones of about 0.52, 0.49, 0.48 and 0.57 nm, for PAF-26-COOLi, PAF-26-COONa, PAF-26-COOK and PAF-26-COOMg, respectively (Table 1 and Fig. S4†). The decreasing trend in the pore size of PAF-26-COOLi, PAF-26-COONa, and PAF-26-COOK samples can be found in comparison with that of the parent sample PAF-26-COOH, which can be explained by the increased ionic radius of Li+ (90 pm), Na+ (116 pm), and K+ (152 pm). The pore size of PAF-26-COOMg is enlarged by exchanging protons with Mg2+ ions. This phenomenon can be interpreted with a first cleavage of hydrogen bonds between neighboring carboxylic groups, and further interaction with the Mg2+ ion. Additionally, the pores in PAF-26-COOH and PAF-26-COOM are very uniform in the microporous range, which is evidenced by the narrow pore size distribution (Fig. S4†) and high micropore volume ratio (∼90%, Table 1).
Gas adsorption
With the successful preparation of PAF-26 polymers with high porosity, fully available functional groups and a pore size around 0.5 nm, PAF-26-COOH and PAF-26-COOM materials were evaluated for gas adsorption or capture. Typically, the adsorption properties of PAF materials for CO2, CH4, and N2 were studied by gas adsorption measurements, of which CO2 and CH4 are two main carbon sources in flue gas, natural gas or landfill gas. Fig. 5 displays CO2 and CH4 adsorption isotherms recorded at 273 K. There is a steep uptake at low relative pressures followed by continuous sorption of CO2 upon increasing the pressure (Fig. 5a). CO2 uptake for the PAF-26-COOH sample is 52 cm3 g−1 at P = 101 kPa. With the substitution of protons by metal ions, improved sorption ability for CO2 is attained (Fig. 5a). For a better understanding of the effect of different metal ions on the capture performance, the gas uptakes were normalized by the pore volume (Vtotal) to assess the sorption capacities of different adsorbents.5f The uptakes per effective Vtotalversus pressure for all PAF-26 materials toward CO2 are plotted in Fig. 5b. A distinct enhancement is observed in the uptake per effective Vtotal after metallization with Li+, Na+, K+ and Mg2+ if the proton form of the PAF-26-COOH material is taken as a reference (Fig. 5b). PAF-26-COOK can adsorb 572 mg CO2 per cm3, which is much higher than that of its parent PAF-26-COOH (286 mg cm−3). This sheds light on the improved adsorption affinity of PAF-26-COOM toward CO2. In the same manner, methane adsorption was also carried out. More than 16 cm3 g−1 of methane is adsorbed in PAF-26 samples at 273 K and 101 kPa (Fig. 5c). The dependence of uptakes per effective Vtotal on pressure for the PAF-26 series towards CH4 is also calculated and shown in Fig. 5d. It can be found that CH4 uptake per effective Vtotal increases from 34 mg cm−3 (PAF-26-COOH) to 46 mg cm−3 (PAF-26-COOLi, 35% increase), 54 mg cm−3 (PAF-26-COOMg, 59% increase), 56 mg cm−3 (PAF-26-COONa, 65% increase) and 60 mg cm−3 (PAF-26-COOK, 76% increase). Based on the observations above, it can be concluded that introduction of metal active centers would greatly promote gas adsorption capacity (especially for CO2 and CH4) and discriminate the adsorption affinity toward specific gases. To probe the influence of water on CO2 uptake, the pretreated PAF-26-COOMg samples were exposed to wet air (the average relative humidity is about 50%) for 3 days. As indicated in Fig. S8†, CO2 uptake of the humidified sample represents a 38% drop relative to the outgassed network. This behavior is similar to –OH containing POPs under humid conditions, which also preferentially adsorb water molecules.14 The cyclic CO2 adsorption–desorption study is also carried out. As shown in Fig. S8†, the CO2 uptake of PAF-26-COOMg remains almost constant after exposure to air for more than 4 months, which implies that metalized PAFs are quite stable.
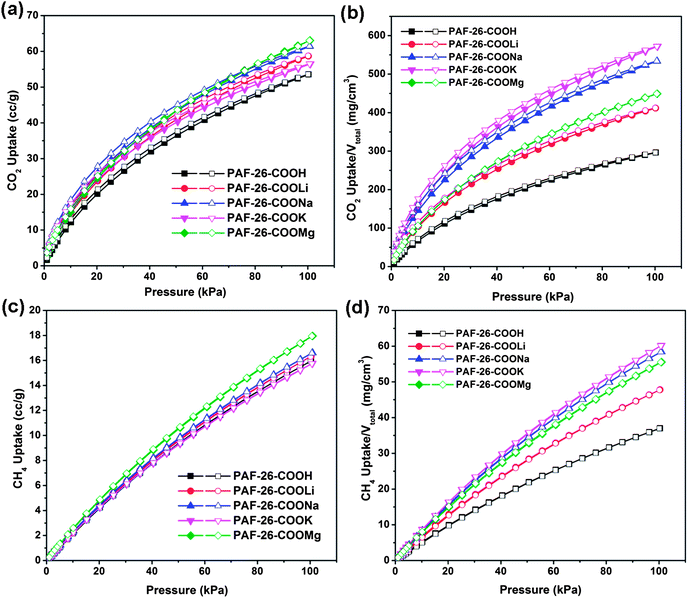 |
| Fig. 5 Gas sorption isotherms of CO2 (a), CO2 uptakes per effective Vtotal (b), CH4 (c), CH4 uptakes per effective Vtotal (d) for PAF-26-COOH, PAF-26-COOLi, PAF-26-COONa, PAF-26-COOK and PAF-26-COOMg samples at 273 K and 101 kPa. | |
As we know, the isosteric heat of adsorption (Qst) is another index to estimate the affinity of an adsorbate to an adsorbent,15 which is helpful to understand the relationship between apparent sorption capacity and metal ion modified frameworks. Based on the adsorption isotherms at different temperatures (273 K and 298 K, Fig. S5 and S6†), the isosteric heats of adsorption of CO2 and CH4 can be determined for each material according to the Clausius–Clapeyron equation (the calculation is detailed in the ESI†). As shown in Fig. 6a, the Qst values of CO2 for PAF-26-COOM significantly increased compared with its counterpart PAF-26-COOH. Typically, the CO2Qst of PAF-26-COOH at low uptake is 28.1 kJ mol−1 (Fig. 6a and Table 2), while the CO2Qst for PAF-26-COONa reaches 35.0 kJ mol−1 and notably high Qst values for other metalized materials are also obtained (31.8 kJ mol−1, 32.6 kJ mol−1 and 30.0 kJ mol−1 for PAF-26-COOLi, PAF-26-COOK and PAF-26-COOMg, respectively). The present data from adsorption measurements for iso-structural PAF-26 materials with exchanged metal ions show a strong influence of metal sites on isosteric heats of adsorption. More interestingly, there is a decreasing trend in CO2Qst values of 35.0, 32.6, 31.8, 30.0 kJ mol−1 with the sequence PAF-26-COONa, PAF-26-COOK, PAF-26-COOLi and PAF-26-COOMg, which coincides with their corresponding basicities originating from compensated alkali or alkaline earth ions (pKb for PAF-26-COONa, PAF-26-COOK, PAF-26-COOLi, and PAF-26-COOMg are 37.96, 36, 33.95, and 15.08). Because there is less –COOH in the structural unit, the CO2Qst of PAF-26-COOH (28.1 kJ mol−1) is lower than that of CMP-1-COOH (33 kJ mol−1).12c Zhou's group reported a sulfonate-grafted PPN–SO3H with a CO2Qst value of 30.4 kJ mol−1. After lithiation, the CO2Qst of PPN-6-SO3Li reached 35.7 kJ mol−1.11g In this case, after metalization with Na+, the CO2Qst of PAF-26-COONa is up to 35 kJ mol−1, which is comparable to PPN-6-SO3Li and Li doped MOFs.11h Nevertheless, these Qst values of PAF-26-COOM are among those of many state-of-the-art excellent porous materials, which can be clearly seen in Table S2.†
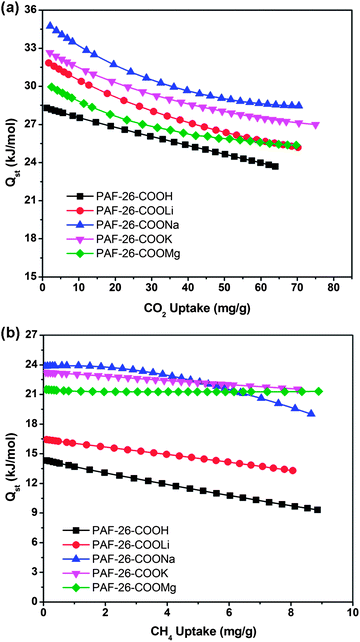 |
| Fig. 6 The isosteric heats of adsorption for CO2 (a) and CH4 (b) of PAF-26-COOH, PAF-26-COOLi, PAF-26-COONa, PAF-26-COOK and PAF-26-COOMg as a function of gas uptake. | |
Table 2 Isosteric heats of adsorption for CO2 and CH4, and selectivities of CO2–N2 and CH4–N2 for PAF-26 materials
Samples |
Q
st for CO2 |
Q
st for CH4 |
Selectivity for CO2–N2a |
Selectivity for CO2–CH4a |
Selectivity for CH4–N2a |
Selectivity was calculated by IAST at 298 K and 110 kPa.
|
PAF-26-COOH |
28.1 |
14.3 |
20 |
4 |
4.2 |
PAF-26-COOLi |
31.8 |
16.5 |
24 |
5.6 |
4.4 |
PAF-26-COONa |
35.0 |
24.0 |
53 |
9 |
5.0 |
PAF-26-COOK |
32.6 |
23.0 |
50 |
8.6 |
5.8 |
PAF-26-COOMg |
30.0 |
21.5 |
73 |
8.4 |
6.5 |
Parallel Qst determination of CH4 for PAF-26 materials was also carried out. The isosteric heats of adsorption for all measured materials are shown in Fig. 6b as a function of CH4 gas uptake. As clearly seen in Fig. 6b and Table 2, the initial CH4Qst values for PAF-26-COOLi, PAF-26-COOMg, PAF-26-COOK and PAF-26-COONa are 16.5, 21.5, 23.0, and 24.0 kJ mol−1, which are remarkably higher than that of PAF-26-COOH (14.3 kJ mol−1). In general, a high isosteric heat of adsorption could lead to high gas storage at low pressures, which has already been exemplified by the adsorption measurements (Fig. 5). Notably, the high Qst of PAF-26-COOM for CH4 (16–24 kJ mol−1) guarantees PAF-26 materials to be ranked in the top level of porous materials as can be seen from other outstanding porous materials, such as MIL-53 (18.0–19.0 kJ mol−1),16 HKUST-1 (16.6–20.7 kJ mol−1),17 MOF-5 (12.2 kJ mol−1),18 and BILP-1 to BILP-7 (13–18.4 kJ mol−1).19 Furthermore, higher Qst values for PAF-26-COOM provide evidence for the stronger interaction between CH4 molecules and PAF-26-COOM frameworks in contrast to PAF-26-COOH. Based on the results above, the possible reasons for the strong interaction between PAF-26-COOM materials and CH4 gas molecules can be explained as follows: on one hand, more charges on metal centers may facilitate polarization of the CH4 molecule and result in a stronger binding force. On the other hand, pore effect has to be considered. Small pores would result in an overlap of the van der Waals forces of neighboring atoms, which gives rise to a strong interaction.20 From the adsorption observations, one can conclude that PAF-26 materials with permanent small pores and high isosteric heats of adsorption have been synthesized.
Gas selectivity studies
The capture of CO2 and CH4 carbon resources is very important in energy conservation. For practical application, the separation of CO2 from N2 in flue gas is critical to reduce global greenhouse effect. Upgradation of landfill gas or coal-bed gas is demanded to extract CH4 from N2. High adsorption capacities of PAF-26 materials toward CO2 and CH4 are demonstrated, while a very small uptake of 3 cm3 g−1 is observed for N2 at 101 kPa and 298 K (Fig. S7†). Adsorption selectivity is another key parameter in the pressure swing adsorption separation process with porous solid materials toward gases especially for carbon-rich CO2 and CH4, which are promising for carbon storage or capture in the future.
In this respect, the selectivity in the capture of CO2 and CH4 from CO2–N2, CH4–N2 gas mixtures is evaluated by the ideal adsorption solution theory (IAST), which is well recognized and employed to predict gas mixture adsorption behaviors in porous materials.21 As a result, selectivity studies of theoretical gas mixtures of CO2–N2, CH4–N2 were conducted using the IAST model utilizing single-component isotherms.
Based on single gas adsorption isotherms using the IAST model, the selectivity of CH4 over N2 (1
:
1 in volume) for PAF-26 materials according to the experimental data is calculated and the results are shown in Fig. 7a. The obtained values of the resulting selectivity of CH4 over N2 are 4.2, 4.4, 5.5, 5.8 and 6.5 for PAF-26-COOH, PAF-26-COOLi, PAF-26-COONa, PAF-26-COOK and PAF-26-COOMg, respectively (Table 2). There is no loss in CH4 selectivity for these four samples in the whole test pressure range (0–110 kPa). As seen in Table 2, the selectivity of CH4 over N2 for PAF-26-COOM is higher than that of PAF-26-COOH (4.2), which provides supporting evidence for the enhanced affinity to CH4 post metalation. The selectivity of all PAF-26 iso-structural materials (PAF-26-COOH and its light metalized derivatives, PAF-26-COOM) for CH4 over N2 surpasses the previously reported porous adsorbents, especially for PAF-26-COOMg with an extraordinarily high value of 6.5, ranking PAF-26-COOMg among the best porous adsorbents for separating CH4 from N2 (Table S2†).
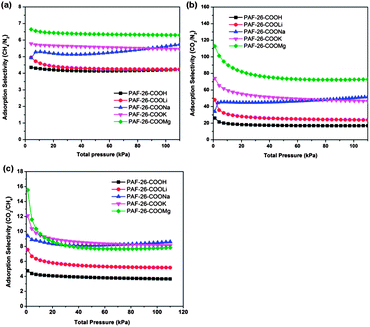 |
| Fig. 7 IAST-predicted adsorption selectivity of CH4–N2 (a) and CO2–N2 (b) and CO2–CH4 (c) at 298 K and 110 kPa for PAF-26-COOH, PAF-26-COOLi, PAF-26-COONa, PAF-26-COOK and PAF-26-COOMg. | |
The calculated adsorption selectivity for 15% CO2 and 85% N2 mixtures in PAF-26 materials as a function of bulk pressure is presented in Fig. 7b. After metalation, the selectivity of PAF-26-COOM increases significantly in comparison with PAF-26-COOH. At 110 kPa, the selectivity of CO2 over N2 in PAF-26-COOH, PAF-26-COOLi, PAF-26-COONa and PAF-26-COOK is estimated to be 20, 24, 50 and 53 in the plateau (Fig. 7b). Exceptionally in the case of PAF-26-COOMg, the selectivity of CO2 over N2 reaches 80 under 110 kPa. The excellent CO2 selectivity in the case of PAF-26-COOMg may be explained by progressive CO2 adsorption onto the active sites of high-valence magnesium under ambient pressure. The CO2–N2 selectivities of PAFs are lower than the N2-phobic azo-COPs reported by Patel et al., which have the highest CO2–N2 selectivity (288) at 323 K.8f The selectivity of CO2 over CH4 (1
:
1 in volume) is also examined as it involves the purification of CH4 from natural gas. As shown in Fig. 7c, the CO2–CH4 selectivity of PAF-26-COOM is also increased in comparison with that of PAF-26-COOH. The selectivity of PAF-26-COONa (9) at 110 kPa is about two times more than that of PAF-26-COOH (4). Combined with adsorption and selectivity studies, one can conclude that the carboxyl-functionalized and post-metalation PAF materials integrate two important characteristics of high sorption capacity and high selectivity. Such PAF materials may be an excellent candidate for carbon capture or storage.
Conclusions
In summary, we have successfully designed and synthesized a carboxyl-functionalized PAF material, PAF-26-COOH. Post-metalation of PAF-26-COOH yields a series of PAF-26-COOM derivatives (M = Li, Na, K, Mg). The structure, morphology and porosity of PAF-26 products are comprehensively investigated by IR, NMR, XRD, SEM and N2 adsorption measurements. Microporous PAF-26 materials with high surface area, high porosity and tunable pore size are achieved via a post-metalation method. High adsorption capacities toward CO2 and CH4 are demonstrated with as-prepared PAF-26 materials, while very low uptake for N2 is measured with the same samples. Additionally, an enhanced adsorption for CO2 and CH4 is observed for PAF-26-COOM in comparison with PAF-26-COOH, which indicates that introduction of light metal ions would improve the adsorption affinity to CO2 and CH4 gases. Further, PAF-26 materials exhibit very high isosteric heats of adsorption toward CO2 and CH4. Interestingly, the isosteric heats of adsorption for CO2 follow a sequence of PAF-26-COOMg < PAF-26-COOLi < PAF-26-COOK < PAF-26-COONa, which matches well with their corresponding basicities. The stronger interaction between CH4 molecules and PAF-26-COOM is verified by their higher isosteric heats of adsorption, which is due to the higher polarity of metal ions than that of protons and the small-pore effect. The selective adsorption toward CO2 and CH4 over N2 is studied and the predicted selectivity is calculated by the IAST model derived from their adsorption isotherms. All measured PAF-26 samples show high selectivity for CO2 over N2 with values of 20–80 and significantly high selectivity for CH4 over N2 with a highest value of 6.5. The combined merits of high sorption capacity and high selectivity make PAF-26 a potential stepping stone to porous materials for carbon capture and storage.
Acknowledgements
We are grateful for the financial support from the National Basic Research Program of China (973 Program, grant nos 2012CB821700), Major International (Regional) Joint Research Project of NSFC (grant nos 21120102034), NSFC (grant nos 20831002, 21201074) and Australian Research Council Future Fellowship (FT100101059).
Notes and references
-
(a) S. A. Montzka, E. J. Dlugokencky and J. H. Butler, Nature, 2011, 476, 43 CrossRef CAS PubMed;
(b) G. Férey, C. Serre, T. Devic, G. Maurin, H. Jobic, P. L. Llewellyn, G. D. Weireld, A. Vimont, M. Daturi and J. S. Chang, Chem. Soc. Rev., 2011, 40, 550 RSC;
(c) J. Liu, P. K. Thallapally, B. P. McGrail, D. R. Brown and J. Liu, Chem. Soc. Rev., 2012, 41, 2308 RSC;
(d) G. Xiao, P. Xiao, S. Lee and P. A. Webley, RSC Adv., 2012, 2, 5291 RSC.
-
(a) Y. S. Bae and R. Q. Snurr, Angew. Chem., Int. Ed., 2011, 50, 11586 CrossRef CAS PubMed;
(b) D. M. D'Alessandro, B. Smit and J. R. Long, Angew. Chem., Int. Ed., 2010, 49, 6058 CrossRef CAS PubMed;
(c) Q. Wang, J. Luo, Z. Zhong and A. Borgna, Energy Environ. Sci., 2011, 4, 42 RSC.
-
(a) P. Markewitz, W. Kuckshinrichs, W. Leitner, J. Linssen, P. Zapp, R. Bongartz, A. Schreiber and T. E. Müller, Energy Environ. Sci., 2012, 5, 7281 RSC;
(b) L. S. Fan, L. Zeng, W. Wang and S. Luo, Energy Environ. Sci., 2012, 5, 7254 RSC.
-
(a) P. Y. Li and F. H. Tezel, Microporous Mesoporous Mater., 2007, 98, 94 CrossRef CAS PubMed;
(b) S. Cavenati, C. A. Grande and A. E. Rodrigues, J. Chem. Eng. Data, 2004, 49, 1095 CrossRef CAS;
(c) J. M. Leyssale, G. K. Papadopoulos and D. N. Theodorou, J. Phys. Chem. B, 2006, 110, 22742 CrossRef CAS PubMed;
(d) L. Grajciar, J. Čejka, A. Zukal, O. Areán, C. T. Palomino and G. P. Nachtigall, ChemSusChem, 2012, 5, 2011 CrossRef CAS PubMed.
-
(a) S. Cavenati, C. A. Grande and A. E. Rodrigues, Sep. Sci. Technol., 2005, 40, 2721 CrossRef CAS;
(b) R. Babarao, Z. Q. Hu, J. W. Jiang, S. Chempath and S. I. Sandler, Langmuir, 2007, 23, 659 CrossRef CAS PubMed;
(c) M. B. Kim, Y. S. Bae, D. K. Choi and C. H. Lee, Ind. Eng. Chem. Res., 2006, 45, 5050 CrossRef CAS;
(d) V. Goetz, O. Pupier and A. Guillot, Adsorption, 2006, 12, 55 CrossRef CAS;
(e) Z. Liu, C. A. Grande, P. Li, J. Yu and A. E. Rodrigues, Sep. Sci. Technol., 2011, 46, 434 CrossRef CAS;
(f) C. Pevida, M. G. Plaza, B. Arias, J. Fermoso, F. Rubiera and J. J. Pis, Appl. Surf. Sci., 2008, 254, 7165 CrossRef CAS PubMed.
-
(a) P. J. E. Harlick and A. Sayari, Ind. Eng. Chem. Res., 2007, 46, 446 CrossRef CAS;
(b) R. Serna-Guerrero, Y. Belmabkhout and A. Sayari, Adsorption, 2010, 16, 567 CrossRef CAS;
(c) Y. Kuwahara, D. Y. Kang, J. R. Copeland, N. A. Brunelli, S. A. Didas, P. Bollini, C. Sievers, T. Kamegawa, H. Yamashita and C. W. Jones, J. Am. Chem. Soc., 2012, 134, 10757 CrossRef CAS PubMed;
(d) S. A. Didas, A. R. Kulkarni, D. S. Sholl and C. W. Jones, ChemSusChem, 2012, 5, 2058 CrossRef CAS PubMed;
(e) W. Chaikittislip, R. Khunsupat, T. T. Chen and C. W. Jones, Ind. Eng. Chem. Res., 2011, 50, 14203 CrossRef;
(f) S. Choi, M. L. Gray and C. W. Jones, ChemSusChem, 2011, 4, 628 CrossRef CAS PubMed;
(g) W. Li, S. Choi, J. H. Drese, M. Hornbostel, G. Krishnan, P. M. Eisenberger and C. W. Jones, ChemSusChem, 2010, 3, 899 CrossRef CAS PubMed;
(h) J. H. Drese, S. Choi, R. Lively, W. J. Koros, D. J. Fauth, M. L. Gray and C. W. Jones, Adv. Funct. Mater., 2009, 19, 3821 CrossRef CAS;
(i) Y. Kuwahara, D. Y. Kang, J. R. Copeland, P. Bollini, C. Sievers, T. Kamegawa, H. Yamashita and C. W. Jones, Chem.–Eur. J., 2012, 18, 16649 CrossRef CAS PubMed.
-
(a) R. E. Morris and P. S. Wheatley, Angew. Chem., Int. Ed., 2008, 47, 4966 CrossRef CAS PubMed;
(b) T. M. McDonald, W. R. Lee, J. A. Mason, B. M. Wiers, C. S. Hong and J. R. Long, J. Am. Chem. Soc., 2012, 134, 7056 CrossRef CAS PubMed;
(c) J. R. Li, W. Lu and H. C. Zhou, Chem. Soc. Rev., 2012, 41, 7761 RSC;
(d) S. Choi, J. H. Drese and C. W. Jones, ChemSusChem, 2009, 2, 796 CrossRef CAS PubMed;
(e) K. Sumida, D. L. Rogow, J. A. Mason, T. M. McDonald, E. D. Bloch, Z. R. Herm, T. H. Bae and J. R. Long, Chem. Rev., 2012, 112, 724 CrossRef CAS PubMed;
(f) R. Luebke, J. F. Eubank, A. J. Cairns, Y. Belmabkhout, L. Wojtas and M. Eddaoudi, Chem. Commun., 2012, 48, 1455 RSC.
-
(a) P. Pandey, A. P. Katsoulidis, I. Eryazici, Y. Wu, M. G. Kanatzidis and S. T. Nguyen, Chem. Mater., 2010, 22, 4974 CrossRef CAS;
(b) R. Dawson, E. Stöckel, J. R. Holst, D. J. Adams and A. I. Cooper, Energy Environ. Sci., 2011, 4, 4239 RSC;
(c) T. C. Drage, C. E. Snape, L. A. Stevens, J. Wood, J. Wang, A. I. Cooper, R. Dawson, X. Guo, C. Satterley and R. Irons, J. Mater. Chem., 2012, 22, 2815 RSC;
(d) Q. Chen, M. Luo, P. Hammershoj, D. Zhou, Y. Han, B. W. Laursen, C. G. Yan and B. H. Han, J. Am. Chem. Soc., 2012, 134, 6084 CrossRef CAS PubMed;
(e) Y. Luo, B. Li, W. Wang, K. Wu and B. Tan, Adv. Mater., 2012, 24, 5703 CrossRef CAS PubMed;
(f) H. A. Patel, S. H. Je, J. Park, D. P. Chen, Y. Jung, C. T. Yavuz and A. Coskun, Nat. Commun., 2013, 4, 1357 CrossRef PubMed;
(g) N. Du, H. Park, G. P. Robertson, M. M. Dal-Cin, T. Visser, L. Scoles and M. D. Guiver, Nat. Mater., 2011, 10, 372 CrossRef CAS PubMed.
-
(a) M. H. Weston, O. K. Farha, B. G. Hauser, J. T. Hupp and S. T. Nguyen, Chem. Mater., 2012, 24, 1292 CrossRef CAS;
(b) N. B. McKeown and P. M. Budd, Chem. Soc. Rev., 2006, 35, 675 RSC;
(c) J. X. Jiang, F. Su, A. Trewin, C. D. Wood, N. L. Campbell, H. Niu, C. Dickinson, A. Y. Ganin, M. J. Rosseinsky, Y. Z. Khimyak and A. I. Cooper, Angew. Chem., Int. Ed., 2007, 46, 8574 CrossRef CAS PubMed;
(d) R. Dawson, A. I. Cooper and D. J. Adams, Prog. Polym. Sci., 2012, 37, 530 CrossRef CAS PubMed;
(e) T. Ben, H. Ren, S. Q. Ma, D. P. Cao, J. H. Lan, X. F. Jing, W. C. Wang, J. Xu, F. Deng, J. M. Simmons, S. L. Qiu and G. S. Zhu, Angew. Chem., Int. Ed., 2009, 48, 9457 CrossRef CAS PubMed;
(f) W. Lu, D. Yuan, D. Zhao, C. I. Schilling, O. Plietzsch, T. Muller, S. Bräse, J. Guenther, J. Blümel, R. Krishna, Z. Li and H. C. Zhou, Chem. Mater., 2010, 22, 5964 CrossRef CAS;
(g) P. Kuhn, M. Antonietti and A. Thomas, Angew. Chem., Int. Ed., 2008, 47, 3450 CrossRef CAS PubMed;
(h) H. Furukawa and O. M. Yaghi, J. Am. Chem. Soc., 2009, 131, 8875 CrossRef CAS PubMed;
(i) A. P. Cote, A. I. Benin, N. W. Ockwig, M. O'Keeffe, A. J. Matzger and O. M. Yaghi, Science, 2005, 310, 1166 CrossRef CAS PubMed;
(j) H. M. Kaderi, J. R. Hunt, J. L. Mendoza, A. P. Cote, R. E. Taylor, M. O'Keeffe and O. M. Yaghi, Science, 2007, 316, 268 CrossRef PubMed.
-
(a) W. Lu, J. P. Sculley, D. Yuan, R. Krishna, Z. Wei and H. C. Zhou, Angew. Chem., Int. Ed., 2012, 51, 7480 CrossRef CAS PubMed;
(b) R. Dawson, A. Laybourn, Y. Z. Khimyak, D. J. Adams and A. I. Cooper, Macromolecules, 2010, 43, 8524 CrossRef CAS.
-
(a) K. L. Mulfort, O. K. Farha, C. L. Stern, A. A. Sarjeant and J. T. Hupp, J. Am. Chem. Soc., 2009, 131, 3866 CrossRef CAS PubMed;
(b) A. Li, R. Lu, Y. Wang, X. Wang, K. Han and W. Deng, Angew. Chem., Int. Ed., 2010, 49, 3330 CrossRef CAS PubMed;
(c) K. L. Mulfort and J. T. Hupp, J. Am. Chem. Soc., 2007, 129, 9604 CrossRef CAS PubMed;
(d) D. Himsl, D. Wallacher and M. Hartmann, Angew. Chem., Int. Ed., 2009, 48, 4639 CrossRef CAS PubMed;
(e) S. Yang, X. Lin, A. J. Blake, K. M. Thomas, P. Hubberstey, N. R. Champness and M. Schröder, Chem. Commun., 2008, 6108 RSC;
(f) F. Nouar, J. Eckert, J. F. Eubank, P. Forster and M. Eddaoudi, J. Am. Chem. Soc., 2009, 131, 2864 CrossRef CAS PubMed;
(g) W. Lu, D. Yuan, J. Sculley, D. Zhao, R. Krishna and H. C. Zhou, J. Am. Chem. Soc., 2011, 133, 18126 CrossRef CAS PubMed;
(h) Z. Xiang, Z. Hu, D. Cao, W. Yang, J. Lu, B. Han and W. Wang, Angew. Chem., Int. Ed., 2011, 50, 491 CrossRef CAS PubMed.
- The palladium-catalyzed Sonogashira–Hagihara cross-coupling reaction is also known for the synthesis of conjugated microporous polymers (CMPs) reported by the group of Andrew I. Cooper. See:
(a) J. X. Jiang, F. Su, A. Trewin, C. D. Wood, H. Niu, J. T. A. Jones, Y. Z. Khimyak and A. I. Cooper, J. Am. Chem. Soc., 2008, 130, 7710 CrossRef CAS PubMed;
(b) J. X. Jiang, A. Trewin, F. Su, C. D. Wood, H. Niu, J. T. A. Jones, Y. Z. Khimyak and A. I. Cooper, Macromolecules, 2009, 42, 2658 CrossRef CAS;
(c) R. Dawson, D. J. Adams and A. I. Cooper, Chem. Sci., 2011, 2, 1173 RSC.
- E. Stöckel, X. Wu, A. Trewin, C. D. Wood, R. Clowes, N. L. Campbell, J. T. A. Jones, Y. Z. Khimyak, D. J. Adams and A. I. Cooper, Chem. Commun., 2009, 212 RSC.
- R. Dawson, L. A. Stevens, T. C. Drage, C. E. Snape, M. W. Smith, D. J. Adams and A. I. Cooper, J. Am. Chem. Soc., 2012, 134, 10741 CrossRef CAS PubMed.
-
(a) S. K. Bhatia and A. L. Myers, Langmuir, 2006, 22, 1688 CrossRef CAS PubMed;
(b) R. C. Lochan and M. Head-Gordon, Phys. Chem. Chem. Phys., 2006, 8, 1357 RSC.
- S. Bourrelly, P. L. Llewellyn, C. Serre, F. Millange, T. Loiseau and G. Ferey, J. Am. Chem. Soc., 2005, 127, 13519 CrossRef CAS PubMed.
- J. R. Karra and K. S. Walton, J. Phys. Chem. C, 2010, 114, 15735 CAS.
- W. Zhou, H. Wu, M. R. Hartman and T. Yildirim, J. Phys. Chem. C, 2007, 111, 16131 CAS.
- M. G. Rabbani and H. M. El-Kaderi, Chem. Mater., 2012, 24, 1511 CrossRef CAS.
-
(a) Y. F. He and N. A. Seaton, Langmuir, 2005, 21, 8297 CrossRef CAS PubMed;
(b)
K. Mosher and W. Jennifer, The Impact of Pore Size On Methane and CO2 Adsorption In Carbon, M.S. thesis, Stanford University, 2011.
- A. L. Myers and J. M. Prausnitz, AIChE J., 1965, 11, 121 CrossRef CAS.
Footnote |
† Electronic supplementary information (ESI) available: Additional figures of PAF-26-COOM (IR, XRD, SEM), pore size distribution, calculation of Qst, basicity and Ideal Adsorption Solution Theory (IAST) calculations. See DOI: 10.1039/c3py00647f |
|
This journal is © The Royal Society of Chemistry 2014 |
Click here to see how this site uses Cookies. View our privacy policy here.