DOI:
10.1039/C3NR03791F
(Paper)
Nanoscale, 2014,
6, 334-341
Redox preparation of mixed-valence cobalt manganese oxide nanostructured materials: highly efficient noble metal-free electrocatalysts for sensing hydrogen peroxide†
Received
23rd July 2013
, Accepted 2nd October 2013
First published on 4th October 2013
Abstract
High-performance hydrogen peroxide sensors provide valuable signals of biological interactions, disorders, and developing of diseases. Low-cost metal oxides are promising alternatives but suffer from low conductivity and sensing activity. Multi-component metal oxides are excellent candidates to accomplish these challenges, but the composition inhomogeneity is difficult to manage with conventional material preparation. We demonstrated redox preparation strategies to successfully synthesize highly homogeneous, noble metal-free H2O2 sensors of spinel nanostructured cobalt manganese oxides with enhanced conductivity, multiple mixed-valence features, and efficient H2O2 sensing activities. The designed redox reactions accompanied with material nucleation/formation are the key factors for compositional homogeneity. High conductivity (1.5 × 10−2 S cm−1) and H2O2 sensing activity (12 times higher than commercial Co3O4) were achieved due to the homogeneous multiple mixed-valence systems of Co(II)/(III) and Mn(III)/(IV). A wide linear detection range (from 0.1 to 25 mM) with a detection limit of 15 μM was observed. Manganese species assist the formation of large surface area nanostructures, enhancing the H2O2 reduction activities, and inhibit the sensing interference. The material controls of hierarchical nanostructures, elemental compositions, porosity, and electrochemical performances are highly associated with the reaction temperatures. The temperature-dependent properties and nanostructure formation mechanisms based on a reaction rate competition are proposed.
1. Introduction
A significant amount of scientific evidence shows that hydrogen peroxide is involved in cell signalling pathways of growing processes in living organisms.1,2 The disorder of H2O2 production is associated with the onset and development of diabetes, cancers, cardiovascular diseases, etc.3 Noble metals (Au, Ag, Pt, etc.) and their composites4–11 show remarkable electrochemical performance for sensing H2O2 due to excellent conductivities and homogeneous mixed-valence redox pairs on surface. However, the scarcity and high cost of noble metals hinder their practical applications. Alternatively, transition metal oxides with low production costs, easy storage/activity preservation, and exceptional anti-corrosion stabilities make them promising candidates for inexpensive H2O2 sensors.7,12–14 As the potential replacement for noble metals, both the improvement of conductivity and large amount of accessible electrocatalytic-active sites in metal oxides pose the key challenges.
Doping impurities in host materials is a common synthetic approach to achieve these requirements. Due to different chemical reactivities between each metal species, however, a homogenous distribution of dopants in host materials is difficult to manage, particularly for conventional methods by precipitation and high-temperature sintering. For example, under particular pH conditions, binary metal oxides prepared by co-precipitation could encounter inhomogeneous composition problems as each metal species has different intrinsic precipitation rates (or solubility product constant values, Ksp). An inhomogeneous distribution of metal cations not only interrupts the electron hopping process, but also inhibits the formation of local active pairs comprised of multiple valence cations. Material preparation with segregation-free elemental homogeneity is desired for highly-efficient multi-component metal oxide H2O2 sensors.
In our previous work, a general concept of utilizing redox routes to produce binary metal oxide materials with controls of the hierarchical nanostructures, specific composition ratios, and highly homogeneous elemental distribution was demonstrated.15 In contrast to the conventional methods above, the aim of our redox routes was to create a direct electron exchange situation between the only two metal reagents used in the preparation. While the redox occurring is associated with the crystal nucleation of products, a homogeneous distribution of elements in the products is highly achievable.
Studies have shown that charge transfer via hopping processes between cations of different valencies requires relatively lower activation energies.16 Thus intrinsic mixed-valence systems can significantly improve the conductivity and catalytic activities of metal oxides. A spinel structure is a thermodynamically stable phase naturally comprised of mixed-valence metal cations. To obtain high sensing activities, we attempted to create a multiple mixed-valence system based on a spinel structure with more than one metal cation, including cobalt and manganese. With a homogeneous distribution of multiple mixed-valence species, the complex catalytic interactions and hopping possibilities would be more beneficial for the sensing applications.
Herein, we successfully prepared highly-efficient, nanostructured spinel cobalt manganese oxide sensors with high conductivity, multiple mixed-valence features, and large surface areas via a single-step redox preparation. Spinel cobalt oxide hosts with a mixed-valence pair of Co(II)/Co(III), as well as the incorporated manganese species of Mn(III)/Mn(IV), were selected for multiple mixed-valence systems due to their ease of structural transformation and high activity.16–18 Our synthetic strategies were designed based on: (1) homogeneous hydroxide precursors of cobalt and manganese being generated first by the simple redox routes, (2) the precursors then being transformed into a spinel structure to obtain the desired multiple mixed-valence systems with high homogeneity, and (3) using manganese incorporation to stimulate the formation of porous hierarchical nanostructures for efficient mass transport. The as-obtained spinel samples display much enhanced electrochemical sensing activities compared to those of commercial Co3O4, consolidating a positive response to our design concept of redox preparation. Experimental results further reveal the possible nanostructure formation mechanisms dominated by the reaction rate competition between two different pathways.
2. Experimental
2.1. Reagents
Cobalt sulfate heptahydrate (CoSO4·7H2O), potassium permanganate (KMnO4), and Nafion solutions (5% in alcohols with 20% water) were supplied by Sigma-Aldrich. Dopamine (DA), ascorbic acid (AA), and uric acid (UA) were obtained from Alfa Aesar. Hydrogen peroxide solution (30%) was obtained from Katayama Chemical Industry company. Sodium dihydrogen phosphate (NaH2PO4) and disodium hydrogen phosphate (Na2HPO4) were purchased from Panreac Co. All the chemicals were used in the experiments without further purification.
2.2. Preparation of spinel cobalt manganese oxides
Cobalt sulfate heptahydrate (8.08 mmol) and potassium permanganate (2.69 mmol) was mixed in 71 mL deionized (D.I.) water to yield a purple solution, transferred to a 125 mL Teflon-lined autoclave and reacted hydrothermally with the temperature ranging from 60 °C to 150 °C for 24 hours. The as-obtained precipitates were collected and washed several times with D.I. water, and then dried at 60 °C for 12 hours to yield black powders. These powders were further calcined at 500 °C for 6 hours to obtain nanostructured spinel cobalt manganese oxides (SCM). SCM samples prepared at several reaction temperatures of 60 °C, 80 °C, 100 °C, 150 °C, and 200 °C are denoted as SCM-60, SCM-80, SCM-100, SCM-150, and SCM-200.
2.3. Preparation of SCM-modified electrodes
Glassy carbon electrodes (GCE) with a diameter of 3 mm were polished with alumina powders and rinsed with D.I. water to yield a clean surface. A homogeneous suspension of 1 mg SCM in 1 mL ethanol was obtained after 30 min sonication. A drop of 10 μL from this suspension was placed on the surface of a GCE and dried for 10 minutes at room temperature. An aliquot of 10 μL Nafion (0.1%) was deposited on top of the dried samples to generate the modified electrodes for electrochemical measurements.
2.4. Characterization and electrochemical measurements
The X-ray diffraction (XRD) patterns were obtained using a Bruker D8 Advance diffractometer with a CuKα X-ray source. The morphology of the samples was studied with a Zeiss Supra 55 Gemini field emission scanning electron microscope (FE-SEM) with an acceleration voltage of 1 kV. Elemental analyses of the materials were carried out using the techniques of energy dispersive X-ray spectroscopy (EDXS), equipped in the FE-SEM. Transmission electron microscopy (TEM) images were obtained with a FEI Tecnai F20 at 200 kV. Nitrogen adsorption–desorption isotherms were measured with a Micromeritics ASAP 2010 instrument. The Brunauer–Emmett–Teller (BET) method was used to calculate the surface areas. The pore size distributions were derived from the adsorption branches of the Barrett–Joyner–Halenda (BJH) method. Thermogravimetry analysis (TGA) was carried out on a PerkinElmer TGA4000 thermal analyzer in N2 with a flow rate 30 mL min−1 at temperatures ranging from room temperature to 900 °C with a heating rate of 10°C min−1. The conductivity measurements were performed with a Quatek 5601Y Sheet Resistivity Meter 4 Point Probe.
In a typical hydrogen peroxide sensing experiment, certain amounts of H2O2 solution (10, 50, 100 μL of 0.5 M H2O2) were injected into a 0.1 M phosphate buffer solution (PBS) at pH = 7.2. A CHI 614D electrochemical analyzer was used for all the electrochemical measurements with a conventional three-electrode configuration using Ag/AgCl (saturate NaCl) as the reference electrode and Pt as the counter electrode.
3. Results
3.1. Synthesis and characterization of the spinel cobalt manganese oxides
The binary cobalt manganese oxide precursors were produced via a single-step redox reaction between Co(II) and Mn(VII) in acidic conditions first. The Co(II) in cobalt sulfate was oxidized to Co(III) with the reduction of Mn(VII) in potassium permanganate to Mn(IV), yielding black fine powders as the precursors in this study. The XRD patterns confirm the presence of a pure cobalt oxide hydroxide (CoOOH) phase in the as-obtained precursors (Fig. S1†). The redox chemistry is expressed as the following equation:15 | 3 CoII + MnVII → 3 CoIII + MnIV | (1) |
The XPS results of the precursors (Fig. S2†) confirm the presence of Co(III) and Mn(IV) species. In the blank experiments, the absence of KMnO4 leads to no reaction, indicating that the generation of products is based on the redox pathway of eqn (1).
After a calcination at 500 °C for 6 hours, the layer-structure hydroxide precursors were transformed to the spinel-structure final products, named spinel cobalt manganese oxides (SCM). The SCM samples prepared at 100 °C (SCM-100) show three dimensional (3D) hierarchical nanostructures, in which spherical aggregations of 1–2 micron in diameter are observed (Fig. 1a). The higher magnification SEM images (Fig. 1b) demonstrate that the nanostructures are comprised of continuous networks of nanoflakes with a thickness in the range of 5–10 nm. No significant morphological changes were observed after the calcination procedure. The XRD patterns of SCM-100 in Fig. 1c reveal the presence of the spinel Co3O4 phase (JCPDS 9-418) after the calcination. The XPS results (Fig. S2†) verify the presence of Co(II)/Co(III), but the difference of manganese species after calcination is not clear due to the line-broadening effect.19 The EDXS results of elemental analysis show the signals of both manganese and cobalt in SCM-100 (Fig. 1d). All the characterization results clearly show that the manganese is successfully incorporated into the Co3O4 host structure.
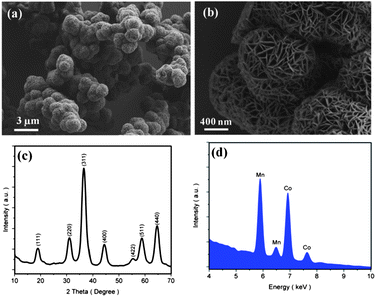 |
| Fig. 1 Material characterization results of SCM-100: (a) the low-magnification SEM images; (b) the high-magnification SEM images; (c) the XRD patterns corresponding to the spinel Co3O4 phase; (d) the EDXS elemental analysis of cobalt and manganese compositions. | |
The TEM results of SCM-100 show a spherical aggregation of nanoflakes without the presence of a hollow core (Fig. 2a). The high magnification images of nanoflakes (Fig. 2b), corresponding to the red square area in Fig. 2a, exhibit a nanoporous feature with pore sizes of around 10 nm. The high resolution (HR) TEM images (Fig. 2c) reveal the lattice fringes with a spacing of 0.289 nm, corresponding to d220 in the spinel Co3O4 structure. In Fig. 2d, the selected area electron diffraction (SAED) patterns with polycrystalline ring features confirm the presence of spinel Co3O4 structures locally in the nanoflakes, showing a good agreement with the bulk phase analysis data from XRD. No impure phases or materials were observed in the TEM characterization, indicating the success of producing single-phase binary materials via the simple redox synthetic preparation.
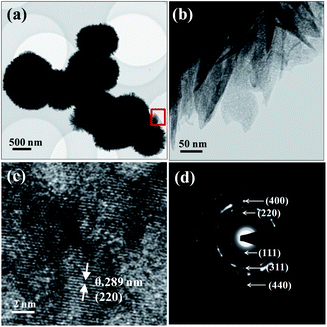 |
| Fig. 2 The TEM results of SCM-100: (a) the low-magnification TEM images; (b) the images of the thin nanoflakes corresponding to the red square area in (a); (c) the HR-TEM images of the nanoflakes showing lattice fringes of d220; (d) the corresponding SAED patterns. | |
3.2. Temperature-dependent growth of the nanostructures
Understanding of the nanostructure formation process and its impacts on electrochemical activities provides valuable information for the optimization of material performances in sensing applications. A series of temperature-dependent experiments was carried out, and the SEM results show that the growth of hierarchical nanostructures is highly associated with the reaction temperature (see ESI, Fig. S3†). The formation of nanostructures is not well observed in the samples prepared at low temperatures (e.g. 60 °C). With the increase of the reaction temperatures, the growth of nanostructures (SCM-80, SCM-100, and SCM-150) is pronounced up to 150 °C. At 200 °C, the generation of two different materials with morphologies of nanowires and nanoflaked microspheres was observed (Fig. S3d†). The EDXS analysis indicates that the nanoflaked microspheres have similar Co/Mn ratios to the other SCM samples, while the nanowires are mainly composed of manganese with a relatively small amount of cobalt (>38% of Mn). The XRD results (Fig. S1b†) show that an additional γ-MnO2 phase can be identified in SCM-200, indicating the presence of the side product of γ-MnO2 nanowires in the sample.
3.3. Porosity and surface area
The results of the Brunauer–Emmett–Teller (BET) surface area are summarized in Table 1. SCM-100 and SCM-150, with 3D nanostructures, have almost identical values of surface area, 57 and 58 m2 g−1, respectively. The relatively low surface area of 42 m2 g−1 was measured for SCM-60, which might be due to the absence of 3D hierarchical nanostructures. The typical isotherms of N2 adsorption–desorption of SCM-100 with the inset showing the pore size distribution are shown in Fig. 3. The uniform pore size distribution at 12 nm is in good agreement with the pore structures of the nanoflakes (around 10 nm) visually observed in the TEM results (Fig. 2b). The hysteresis of the N2 adsorption–desorption isotherms shows a mesopore characteristic in SCM-100. The pore size distribution of nanostructured SCM-150, however, exhibits a broad distribution range centered at around 70 nm (Fig. S4†), unlike the uniform distribution in SCM-100. Although the surface areas of these two nanostructured samples are similar, their pore structures are different.
Table 1 The summary of chemical compositions, conductivity, and surface areas of the SCM samples
Sample |
Co/Mnb |
Conductivity (S cm−1) |
Surface area (m2 g−1) |
The conductivity value is from ref. 44.
The data were acquired using EDXS.
Not available.
|
SCM-60
|
3.0 |
0.9 × 10−2 |
42 |
SCM-100
|
2.6 |
1.5 × 10−2 |
57 |
SCM-150
|
2.3 |
2.9 × 10−2 |
58 |
Co3O4a |
—c |
3.1 × 10−5 |
— |
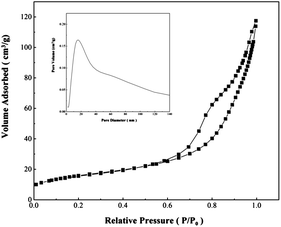 |
| Fig. 3 The N2 adsorption–desorption isothermal plots and pore size distribution (inset) of SCM-100. | |
3.4. Elemental composition and conductivity
The electrochemical performance of materials is known to significantly depend on both chemical compositions and conductivities. The summary of conductivities and chemical compositions in the SCM samples is shown in Table 1. Higher contents of Mn are observed with the higher reaction temperatures in the range of 60–150 °C. The Co/Mn ratios of SCM-60 with values around 3 were measured, close to the stoichiometric redox ratios in eqn (1). Nevertheless, the Co/Mn ratios of SCM-100 and SCM-150 are 2.62 and 2.27, respectively. This observation suggests that additional reaction mechanisms may occur at the elevated reaction temperatures.
The significantly improved conductivities of the SCM materials are around two-three orders of magnitude higher than both spinel Co3O4 (Table 1) and spinel manganese oxide materials20 (10−4 S cm−1), showing the critical role of multiple mixed-valence systems to achieve conductivity improvement. The conductivity difference among SCM samples prepared at different temperatures is less than 0.3% of the improvement over commercial Co3O4, demonstrating the reliable control of material conductivity.
3.5. Thermal stability
The TGA analysis was carried out to understand the thermal behaviors and phase transformation of the SCM-100 precursors (Fig. 4). The uncalcined precursors prepared at 100 °C exhibit an 8.0% weight loss ranging from room temperature to 250 °C due to the desorption of physisorbed water and gaseous molecules on the surface. The large accumulation of surface physisorbed small molecules because of the mesoporous features leads to a significant weight loss of 8%. The second weight loss of 7.2% was observed from 250 °C up to around 330 °C, corresponds to the structural transformation from layered hydroxide form to cubic spinel structures. This transformation temperature range is similar to that of the pure cobalt oxide materials reported in the literature.21,22 The XRD results also confirm that a single phase of the Co3O4 structure was observed in the products after the thermal treatment at 500 °C. No dramatic weight loss at 800 °C, referring to the formation of CoO, was observed.21 Instead, a small weight loss was shown (∼3.3%) from 350 °C to 600 °C, showing the high thermal stability of the binary cobalt manganese oxides.
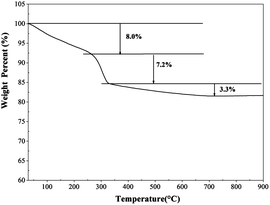 |
| Fig. 4 The TGA curve of the as-obtained SCM-100 precursor. | |
3.6. Electrocatalytic activities of the SCM
The electrocatalytic activities of the SCM modified electrodes for H2O2 sensing were studied using cyclic voltammetry (CV). The CV results (Fig. 5a) show small background signals of GCE in a buffer solution of 1 mM H2O2. The presence of SCM-100 electrocatalysts significantly increases the H2O2 reduction current peaks around −0.75 V, indicating the high electrocatalytic activities of the SCM nanostructured materials. Commercial Co3O4 samples were tested under the same conditions for comparison, and showed much weaker reduction signals. The reduction peaks of SCM-100 are around 2.3 times greater than that of commercial Co3O4.
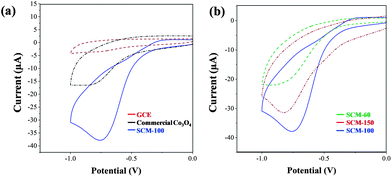 |
| Fig. 5 The cyclic voltammograms (CVs) of the SCM modified electrodes with the presence of 1 mM of hydrogen peroxide in PBS: (a) bare GCE (red dash line), commercial Co3O4 (black dot-dash line) and SCM-100 (blue solid line); (b) the comparison of SCM samples prepared at different temperatures: SCM-60 (dash green line), SCM-150 (red dot-dash line), and SCM-100 (blue solid line). | |
The effects of nanostructure on the electrochemical activities were studied with different SCM samples prepared at various temperatures. Samples of SCM-60, SCM-100, and SCM-150 represent three typical stages in the nanostructure formation process, and were therefore are selected for comparison. SCM-200 was not included in the activity comparison because of the inhomogeneous nanostructures, phases, and side products. As shown in Fig. 5b, SCM-100 exhibits the highest electrochemical activities compared to SCM-60 and SCM-150. The reduction peaks of SCM-100 are around 1.7 times larger than that of SCM-60. Since the nanostructures are absent in SCM-60, the activity enhancement observed in SCM-100 could be mainly due to the formation of the 3D nanostructures.15 The reduction peaks of SCM-150 are ∼80% and 140% of these observed in SCM-100 and SCM-60, respectively. Again, the nanostructures in SCM-150 may result in the stronger reduction activities than “non-nanostructured” SCM-60. A tendency that the samples with 3D nanostructures and high surface areas generally show stronger electrochemical activities is observed. The superior mass transport and large accessible surface active sites gained by the nanostructures significantly contribute to the enhancement of the electrochemical properties.23 The SCM-100 represents the optimized electrocatalyst from the preparation conditions in this series of experiments, and is selected for further detailed electrochemical studies.
The electrochemical response of SCM-100 to various concentrations of H2O2 (0.1–0.9 mM) are shown in Fig. 6. The higher H2O2 concentrations result in stronger reduction peaks. The calibration curve of the corresponding amperometric response is shown as the inset in Fig. 6, revealing a linear relationship up to 0.9 mM of H2O2 (R2 = 0.995). These results demonstrate a typical catalytic H2O2 reduction via the SCM materials with an outstanding sensitivity to the changes in the concentration of hydrogen peroxide. Typical current–time plots of the SCM-100 in PBS over successive step changes of H2O2 concentration are shown in Fig. 7. The baseline stabilization time before performing H2O2 measurement is less than 10 seconds (Fig. S5†). The current changes quickly reach the 95% steady state currents within 3 seconds, exhibiting rapid amperometric response properties. The response steps are sharp in the region of lower concentrations of H2O2 and become noisier with further concentration increases, particularly in the region of ∼30 mM. The detection limit was estimated to be 15 μM at a single-to-noise ratio of 3 with a wide linear detection range from 0.1 to 25 mM (R2 = 0.995). The sensitivity is 2.9 μA mM−1, 12 times higher than commercial Co3O4 materials measured under the same conditions (Fig. S6†), clearly demonstrating the significantly enhanced H2O2 sensing activities in the SCM.
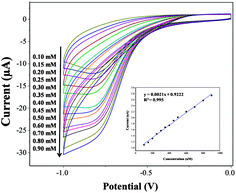 |
| Fig. 6 The CV results of SCM-100 in the presence of H2O2 over a concentration range from 0.1 to 0.9 mM at a scan rate 50 mV s−1. Inset: the corresponding calibration curve of the amperometric response with changes of H2O2 concentration. | |
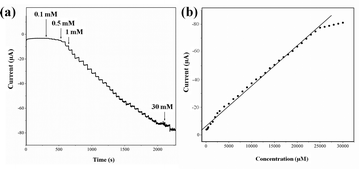 |
| Fig. 7 (a) The amperometric response of SCM-100 at −0.65 V with successive additions of H2O2. (b) The corresponding calibration curve of (a). | |
Different types of the reported electrocatalysts based on noble metals and their composites for enzymeless H2O2 sensors are summarized in Table S1† for comparison, including the working conditions and sensing performance. The noble metal-free SCM sensors exhibit comparable or even superior performance compared to electrocatalysts such as Ag NPs–TiO2 composites, roughened Ag electrodes, porous Au–Pt systems, SiO2/Au core–shell nanowires, and gold nanoplates.4,24–26 In addition, various H2O2 sensors based on metal oxide composites are also summarized in Table S2† for comparison.27–33
3.7. Selectivity and stability
The sensing interference caused by common species of ascorbic acid, uric acid, dopamine, glucose, and acetaminophen was investigated. The concentrations of H2O2 are 2 mM and that of the other five interfering species based on their physiological levels.31,34–36 As shown in Fig. 8, the presence of these interfering species causes negligible current changes compared to that of H2O2. These results indicate the remarkable anti-interference properties of the SCM materials compared to noble metal biosensors.26
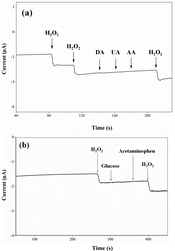 |
| Fig. 8 The current–time curves for the interference studies of H2O2 sensing with SCM-100 and the presence of interfering species of 0.1 mM DA, UA, and AA in (a), as well as glucose (5 mM) and acetaminophen (0.1 mM) in (b). The SCM-100 electrocatalysts are sensitive to the presence of H2O2 (2 mM) and show negligible responses to the interfering species. | |
The long-term stability studies reveal a steady amperometric response with less than ∼7% decay in a continuous H2O2 measurement longer than 1000 seconds (Fig. S7a†). The stability of sensors was evaluated by continuously performing H2O2 detection every day for a period of 7 days (Fig. S7b†). The results exhibit highly stable and reproducible H2O2 sensing signals with a relative standard deviation (RSD) of 3.9%. In addition, the RSD of hydrogen peroxide sensing signals from three SCM-100 modified electrodes is 4.9%. These results demonstrate the excellent selectivity and stability of the SCM materials for detecting H2O2.
4. Discussion
4.1. Homogeneous elemental distribution via simple redox routes
Homogeneous elemental distribution is the first important task for preparing highly-efficient electrocatalysts. This issue significantly affects the performance of multiple mixed-valence systems in the final products. Our strategy was to start by preparing the homogeneous SCM precursors, whose composition uniformity may be passed on to the products. In our preliminary work, the elemental mapping data of the precursors demonstrated excellent homogeneity of Co and Mn.15 The results acquired from bulk measurements of ICP-MS and EDXS of selected local areas were very close to each other (Table S3†), revealing the uniform compositions on all scales. Furthermore, the compositions of the calcined SCM products (both ICP-MS and EDXS results) correspond well to those of the precursors, supporting the concept that pre-determined homogeneity within the precursors can be preserved for segregation-free mixed-valence features in SCM products. Considering unpredictable dopant contents and poor homogeneity in co-precipitation products,37 the redox procedure provides a new pathway to control the composition homogeneity in binary metal oxide preparation.
The occurrence of redox reactions accompanied with material nucleation/formation may give rise to the composition homogeneity in the precursors. Chen et al. reported that the oxidation of Co(II) by persulfate in acidic conditions led to the generation of pure CoOOH.38 The acidic conditions exclude the two-stage pathway possibility that divalent cobalt hydroxide intermediates were formed first in basic conditions, then followed by an oxidation to generate CoOOH products.21 As no product could be obtained without oxidation, the nucleation of CoOOH-structure products is highly associated with the Co(II) oxidation in a very short time domain. The use of permanganate (metal-contained oxidant) to oxidize Co(II) is proposed to trap the Mn cations into the rapid nucleation of the Co(III) species and form local Co–Mn microstructural units/seeds with stoichiometric Co/Mn ratios close to 3 (eqn (1)). Continuous growth of the Mn-traped seeds leads to the observed homogeneity in the SCM precursors with a CoOOH structure.
4.2. Conductivity improvement and multiple mixed-valence systems
In addition to composition homogeneity, high conductivity and catalytic active sites composed of multiple oxidation states are equally critical for high-performance electrocatalysts. With the success of preparing the homogeneous mixed-valence SCM products, the conductivities and electrocatalytic activities were greatly improved. The precursors of SCM-100 possess a conductivity of 4.0 × 10−4 S cm−1, which is around one order of magnitude larger than those of the single oxidation state MnOx (e.g. 10−5–10−6 S cm−1)39 and pure Co3O4. This conductivity improvement in the precursors may represent the effect of multi-cation (or impurity doping) solely, without involving a multiple mixed-valence effect. In contrast, the multiple mixed-valence conductivity in SCM-100 is greater than the precursors by two orders of magnitude. Our conductivity enhancement strategy based on the multiple mixed-valence systems is more effective than mono-cation mixed-valence materials (e.g. Co3O4) and simple multi-cation systems, conventionally obtained by co-precipitation or other methods. Diverse electron hopping mechanisms and catalytic activation routes enable the SCMs to be more efficient sensor materials than conventional metal oxides.
To understand the electrocatalytic effects of multiple mixed-valences better, additional CV experiments were performed on SCM-100 and its hydroxide precursors in the presence of hydrogen peroxide (Fig. 9). No appreciable reduction peak was observed for the hydroxide precursors, indicating that the electrocatalytic H2O2 reduction may not take place effectively with single oxidation-state systems. Since the major difference between these two electrocatalysts lies in the multiple mixed-valence configurations, this phenomenon not only reveals the critical interactions between Co(II)/Co(III) and Mn(III)/Mn(IV) for electrocatalytic reduction activity, but also demonstrates the essential synthetic designs involving multiple mixed-valence pairs for high-performance sensors.
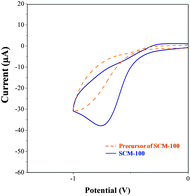 |
| Fig. 9 The CVs of SCM-100 (blue solid line) and the uncalcined precursors of SCM-100 (orange dash line) in the presence of 1 mM hydrogen peroxide in PBS. | |
4.3. Reduction activity enhancement via manganese incorporation
Many metal oxide sensors were developed based on H2O2 oxidation signals, particularly for Co3O4.40,41 But they suffer severely from sensing interference owing to the easy oxidation of other physiological species. Alternatively, signals of H2O2 reduction can be advantageous to inhibit the interference caused by the oxidative-sensing approach. Noble metal sensors also perform in this way generally to avoid interference.26 To realize the goal of utilizing reduction signals, manganese was selected and incorporated into the SCM materials due to its promising reduction activity in other types of electrocatalysis.16,42 As observed in the experimental results (Fig. 5a), the SCM materials show enhanced reduction peaks greater than regular Co3O4 by more than two-fold. The strongly enhanced reduction activity allows SCM sensors to utilize H2O2 reduction signals for sensing, and thus successfully eliminate the interference problems caused by common species including DA, UA, AA, glucose, and acetaminophen (Fig. 8). We further compared the electrocatalytic H2O2 oxidation signals between SCM-100 and commercial Co3O4, however, no significant difference was observed (Fig. S8†). Together with the dramatically enhanced reduction signals in SCM-100, the Mn incorporation exhibits a selective enhancement on reduction activity but not on H2O2 oxidation. This observation would be valuable for the further design of highly selective metal oxide sensors.
4.4. Nanostructure growth mechanism
Although the generation of high surface area nanostructures greatly contributes to efficient mass transport and superior sensing activities, understanding regarding the formation mechanisms is limited. According to previous results, the manganese incorporation stimulates the formation of the continuous interconnected nanoflakes.15 The time-dependent experiments show no morphological evolution in the nanostructure except for the size difference. In this work, we observed that both the Mn contents and morphological evolution were strongly associated with the reaction temperatures. The decrease of Co/Mn ratios (Table 1) indicates that, with reaction temperatures above 60 °C, a certain portion of permanganate species might be incorporated through other pathways, rather than following the ideal redox stoichiometry in eqn (1). Since the synthetic condition is simple with only two chemical reagents in water, the most likely reason is that KMnO4 might be directly reduced by water along the synthetic process:43 | 4 MnO4− + 2 H2O → 4 MnO2 + 4 OH− + 3 O2 | (2) |
The reaction of eqn (2) would lead to the decrease of Co/Mn ratios since permanganate can be reduced without involving Co(II). The drop of the Co/Mn ratios with the increase of reaction temperature indicates that the reaction rate of eqn (2) might be accelerated much more than that of eqn (1). The formation of γ-MnO2 side-products at 200 °C further supports this proposed rate-competition mechanism. As shown in the detailed SEM studies of SCM-200 (Fig. S9†), first, the much faster rates of eqn (2) could result in the rapid formation of 1D manganese oxide nanowire bundles (Fig. S9a†). Second, the nanoflake-like materials, with similar Co/Mn ratios to those observed in the SCM microspheres, are generated on the surface of manganese oxide bundles as the secondary products (Fig. S9b†). In the presence of growth interfaces between SCM nanoflakes and bulk MnO2 bundles (the red box in Fig. S9c†), the secondary formation of SCM microspheres would be most likely due to the relatively slow reaction rates of eqn (1). Finally, the completely separated nanowires covering/wrapping the SCM microspheres were observed to be in the majority (Fig. S9d†). According to these experimental results, the temperature-dependent growth behaviors of SCM materials governed by a reaction rate competition mechanism were rationalized.
5. Conclusions
A redox preparation for SCM materials as low-cost, noble metal-free, and high-performance electrocatalysts for sensing hydrogen peroxide was successfully developed. The challenge of composition homogeneity in binary SCM electrocatalysts was accomplished via the redox routes. The segregation-free multiple mixed-valence systems of Co(II)/Co(III) and Mn(III)/Mn(IV) contribute to the high conductivity and electrocatalytic performance. Manganese incorporation results in high surface area nanostructures, strong H2O2 reduction activities, and low sensing interference. Two major material formation pathways (eqn (1) and (2)) were recognized, and their competition was proposed to be highly temperature-dependent, particularly for eqn (2). The control of reaction temperature exhibits a significant impact on the 3D nanostructures, porosity, Co/Mn ratios, and electrochemical behaviors. The H2O2 sensors composed of SCM-100 show a detection limit of 15 μM with a wide linear detection range from 0.1–25 mM, and an enhanced H2O2 reduction sensitivity that is 12 times larger than commercial products. Our redox approach with the designed synthetic strategy provides a simple protocol to produce reliable and repeatable nanostructured electrocatalysts, offering new synthetic platforms for highly active metal oxide materials for various applications.
Acknowledgements
We acknowledge the financial support of the Taiwan National Science Council under grant NSC101-2113-M-110-005-MY2, and NSYSU-KMU Joint Research Project NSYSUKMU 2013–P017. We also thank Professor Chao-Ming Chiang and Jyh-Tsung Lee for valuable discussion.
Notes and references
- S. G. Rhee, Science, 2006, 312, 1882–1883 CrossRef PubMed.
- J. R. Stone and S. Yang, Antioxid. Redox Signaling, 2006, 8, 243–270 CrossRef CAS PubMed.
- G. C. Van de Bittner, E. A. Dubikovskaya, C. R. Bertozzi and C. J. Chang, Proc. Natl. Acad. Sci. U. S. A., 2010, 107, 21316–21321 CrossRef CAS PubMed.
- J. Shen, X. Yang, Y. Zhu, H. Kang, H. Cao and C. Li, Biosens. Bioelectron., 2012, 34, 132–136 CrossRef CAS PubMed.
- V. K. Shukla, P. Yadav, R. S. Yadav, P. Mishra and A. C. Pandey, Nanoscale, 2012, 4, 3886–3893 RSC.
- J. Tian, H. Li, W. Lu, Y. Luo, L. Wang and X. Sun, Analyst, 2011, 136, 1806–1809 RSC.
- S. Li, Y. Zheng, G. W. Qin, Y. Ren, W. Pei and L. Zuo, Talanta, 2011, 85, 1260–1264 CrossRef CAS PubMed.
- X.-M. Miao, R. Yuan, Y.-Q. Chai, Y.-T. Shi and Y.-Y. Yuan, J. Electroanal. Chem., 2008, 612, 157–163 CrossRef CAS PubMed.
- W. Chen, S. Cai, Q. Q. Ren, W. Wen and Y. D. Zhao, Analyst, 2012, 137, 49–58 RSC.
- Y. Xiao, H. X. Ju and H. Y. Chen, Anal. Chim. Acta, 1999, 391, 73–82 CrossRef CAS.
- C. Lei, S. Hu, G. Shen and R. Yu, Talanta, 2003, 59, 981–988 CrossRef CAS.
- X. Cao, N. Wang, L. Wang, C. Mo, Y. Xu, X. Cai and L. Guo, Sens. Actuator,, 2010, 147, 730–734 CrossRef CAS PubMed.
- Q. Yan, Z. Wang, J. Zhang, H. Peng, X. Chen, H. Hou and C. Liu, Electrochim. Acta, 2012, 61, 148–153 CrossRef CAS PubMed.
- X. Hu, J. C. Yu, J. Gong, Q. Li and G. Li, Adv. Mater., 2007, 19, 2324–2329 CrossRef CAS.
- W.-J. Lan, C.-C. Kuo and C.-H. Chen, Chem. Commun., 2013, 49, 3025–3027 RSC.
- E. Rios, J. L. Gautier, G. Poillerat and P. Chartier, Electrochim. Acta, 1998, 44, 1491–1497 CrossRef CAS.
- C.-H. Chen and S. L. Suib, J. Chin. Chem. Soc., 2012, 59, 465–472 CrossRef CAS.
- Z.-Y. Tian, P. H. Tchoua Ngamou, V. Vannier, K. Kohse-Höinghaus and N. Bahlawane, Appl. Catal., B, 2012, 117–118, 125–134 CrossRef CAS PubMed.
- E. C. Njagi, C.-H. Chen, H. Genuino, H. Galindo, H. Huang and S. L. Suib, Appl. Catal., B, 2010, 99, 103–110 CrossRef CAS PubMed.
- M. J. Iqbal and S. Zahoor, J. Power Sources, 2007, 165, 393–397 CrossRef CAS PubMed.
- J. Yang, H. Liu, W. N. Martens and R. L. Frost, J. Phys. Chem. C, 2010, 114, 111 CAS.
- F. Bardé, M. R. Palacin, B. Beaudoin, A. Delahaye-Vidal and J. M. Tarascon, Chem. Mater., 2004, 16, 299–306 CrossRef.
- G. Wang, D. Cao, C. Yin, Y. Gao, J. Yin and L. Cheng, Chem. Mater., 2009, 21, 5112–5118 CrossRef CAS.
- W. Lian, L. Wang, Y. Song, H. Yuan, S. Zhao, P. Li and L. Chen, Electrochim. Acta, 2009, 54, 4334–4339 CrossRef CAS PubMed.
- Y. J. Lee, C. Oh, J. Y. Park and Y. Kim, IEEE Trans. Nanotechnol., 2011, 10, 1298–1305 CrossRef.
- R. Ning, W. Lu, Y. Zhang, X. Qin, Y. Luo, J. Hu, A. M. Asiri, A. O. Al-Youbi and X. Sun, Electrochim. Acta, 2012, 60, 13–16 CrossRef CAS PubMed.
- Y. Ye, T. Kong, X. Yu, Y. Wu, K. Zhang and X. Wang, Talanta, 2012, 89, 417–421 CrossRef CAS PubMed.
- S. Thiagarajan, T. H. Tsai and S.-M. Chen, Int. J. Electrochem. Sci., 2011, 6, 2235–2245 CAS.
- M. U. Prathap, B. Kaur and R. Srivastava, J. Colloid Interface Sci., 2012, 370, 144–154 CrossRef PubMed.
- K. K. Lee, P. Y. Loh, C. H. Sow and W. S. Chin, Biosens. Bioelectron., 2013, 39, 255–260 CrossRef CAS PubMed.
- S. K. Maji, A. K. Dutta, G. R. Bhadu, P. Paul, A. Mondal and B. Adhikary, J. Mater. Chem. B, 2013, 1, 4127 RSC.
- H. Pang, F. Gao, Q. Chen, R. Liu and Q. Lu, Dalton Trans., 2012, 41, 5862–5868 RSC.
- W. Lu, Y. Luo, G. Chang and X. Sun, Biosens. Bioelectron., 2011, 26, 4791–4797 CrossRef CAS PubMed.
- J. Wang, H. Gao, F. Sun, Q. Hao and C. Xu, Biosens. Bioelectron., 2013, 42, 550–555 CrossRef CAS PubMed.
- C. X. Guo, Z. M. Sheng, Y. Q. Shen, Z. L. Dong and C. M. Li, ACS Appl. Mater. Interfaces, 2010, 2, 2481–2484 CAS.
- X. Qin, W. Lu, Y. Luo, G. Chang, A. M. Asiri, A. O. Al-Youbi and X. Sun, Electrochim. Acta, 2012, 74, 275–279 CrossRef CAS PubMed.
- J. A. Schwarz, C. Contescu and A. Contescu, Chem. Rev., 1995, 95, 477–510 CrossRef CAS.
- C.-H. Chen, S. F. Abbas, A. Morey, S. Sithambaram, L.-P. Xu, H. F. Garces, W. A. Hines and S. L. Suib, Adv. Mater., 2008, 20, 1205–1209 CrossRef CAS.
- A. Kayan, E. Tarcan, U. Kadiroglu and K. Esmer, Mater. Lett., 2004, 58, 2170–2174 CrossRef CAS PubMed.
- W. Jia, M. Guo, Z. Zheng, T. Yu, E. G. Rodriguez, Y. Wang and Y. Lei, J. Electroanal. Chem., 2009, 625, 27–32 CrossRef CAS PubMed.
- C. Hou, Q. Xu, L. Yin and X. Hu, Analyst, 2012, 137, 5803–5808 RSC.
- Y. Liang, H. Wang, J. Zhou, Y. Li, J. Wang, T. Regier and H. Dai, J. Am. Chem. Soc., 2012, 134, 3517–3523 CrossRef CAS PubMed.
- L. Zhang, L. Kang, H. Lv, Z. Su, K. Ooi and Z.-H. Liu, J. Mater. Res., 2011, 23, 780–789 CrossRef.
- Y. Li, P. Hasin and Y. Wu, Adv. Mater., 2010, 22, 1926–1929 CrossRef CAS PubMed.
Footnote |
† Electronic supplementary information (ESI) available. See DOI: 10.1039/c3nr03791f |
|
This journal is © The Royal Society of Chemistry 2014 |