DOI:
10.1039/C3NJ00799E
(Paper)
New J. Chem., 2014,
38, 248-254
Preparation, characterization, and highly effective mercury adsorption of L-cysteine-functionalized mesoporous silica
Received
(in Montpellier, France)
20th July 2013
, Accepted 23rd October 2013
First published on 24th October 2013
Abstract
A novel L-cysteine-functionalized mesoporous SBA-15 (SH-SBA-15) material was synthesized by an easy two-step post-grafting method of L-cysteine using poly(ethylene glycol)-block-poly(propylene glycol)-block-poly(ethylene glycol) as a template under acidic conditions. The material maintained its well-ordered mesostructure after two-step modification as confirmed by powder X-ray diffraction and transmission electron microscopy analyses. X-ray photoelectron spectroscopy and 13C cross-polarization/magic angle spinning NMR revealed that L-cysteine was successfully introduced into SBA-15. The material showed excellent mercury adsorption capacity (429 mg g−1) at room temperature and mercury uptake time (<5 min). Adsorption isotherms and uptake kinetics were also investigated. Equilibrium data were found to be represented better by the Freundlich isotherm model than the Langmuir one. The pseudo-second-order kinetics model best described the kinetic adsorption process of Hg(II) ions onto SH-SBA-15. Moreover, the material possessed high selectivity for mercury and can be easily eluted with 5% thiourea in 1 mol L−1 aqueous HCl.
Introduction
Health and environmental problems related to mercury (Hg) in waste effluents are universally acknowledged. Methods of removing Hg(II) from aqueous solutions include ion exchange, solvent extraction, precipitation, and adsorption.1–8 Among them, the adsorption method is the most effective and low cost one. In this regard, ordered mesoporous materials may offer a promising method of adsorbing Hg in the future.9–11 Mesoporous silica is one of the most attractive mesoporous adsorbents for trapping heavy metal ions because of its high specific surface area, regular and tunable pore size, and so on.12 The adsorption properties of these adsorbents depend on the functional group(s) on their surfaces because research has shown that adsorbents containing sulfur-based ligands (such as thiol, thiourea, and thioether groups) effectively form complexes with mercury ions.13–26
Nevertheless, the aforementioned mercury mesoporous silica adsorbents have all been synthesized using sulfur-containing silane coupling agents or reacted with other sulfur-containing organic compounds, which always have toxicity characteristics and are not cost effective to a certain extent. However, L-cysteine is an accessible and non-toxic biomolecule with a thiol group. In addition, the carboxyl in L-cysteine enables it to combine with alkaline groups such as amino and hydroxyl groups. L-Cysteine-modified multiwalled carbon nanotubes (MWCNTs) and cellulose fibers have been used for the solid-phase extraction of Cd and Hg, respectively.27,28 Unfortunately, the hydrophilicity, long-range order, and specific surface area of MWCNTs and cellulose fibers are limited, leading to low adsorption capacity and rate. Thus, mesoporous silica may be a good choice for wastewater treatment. Thus, L-cysteine-functionalized mesoporous silica is useful for the green treatment of mercury. To the best of our knowledge, no related studies have been reported so far.
In the present study, a highly ordered hexagonal SBA-15-bearing thiol material was prepared for the first time by post-grafting L-cysteine using poly(ethylene glycol)-block-poly(propylene glycol)-block-poly(ethylene glycol) (P123) as a template under acidic conditions. The successful incorporation of thiol groups was confirmed by X-ray photoelectron spectroscopy (XPS) and 13C cross-polarization (CP)/magic angle spinning (MAS) NMR. Hg(II) adsorption behavior on the L-cysteine-modified adsorbent was investigated in terms of mercury static adsorption capacity and uptake kinetics.
Experimental section
Reagents and materials
P123 (average molecular weight, Mav = 5800) and (3-aminopropyl)-triethoxysilane (APTES) were obtained from Sigma-Aldrich. Tetraethoxysilane, L-cysteine (BR; Mav = 121), HNO3, HCl, and NH3·H2O solutions were obtained from Shanghai Sinopharm Chemical Reagent Co., Ltd., China. Dicyclohexylcarbodiimide (DCC) and 4-dimethylaminopyridine (DMAP) were obtained from Shanghai Medpep Co., Ltd, China.
Organic solvents such as toluene, ethanol, and dimethylformamide (DMF) (Shanghai Sinopharm Chemical Reagent Co., Ltd, China) were dried with activated 4 Å-type molecular sieves overnight before use. Heavy metal ion (Hg, Cr, Cd, Pb, and Cu) solutions were obtained by diluting stock standard solutions of 1.000 g L−1 (State Nonferrous Metals and Electronic Materials Analysis and Testing Center, Beijing, China). Water (resistance = 18.25 MΩ cm) used to prepare standard solutions was obtained from a Milli-Q System (Millipore, Bedford, MA, USA). Glassware was soaked in (1 + 1) HCl overnight and cleaned with Milli-Q water before use. All reagents were of analytical grade.
Synthesis of functionalized SBA-15 mesoporous silica
A hexagonal (plane group p6mm) SBA-15 material was prepared with a poly(alkaline oxide) triblock copolymer surfactant in acidic medium according to the method described by Zhao et al.29 The amino-hybrid material was obtained by post-grafting with APTES,30 and the resulting material was named NH2-SBA-15.
Finally, the grafted L-cysteine mesoporous silica was synthesized by reaction between NH2-SBA-15 and L-cysteine. Approximately 1.2 g of NH2-SBA-15 was mixed with 0.3 g, 0.6 g, 1.2 g and 2.4 g of L-cysteine, respectively. 1.0 g of DCC and 0.06 g of DMAP were added to 120 mL of DMF as solvent. The mixture was refluxed at 100 °C for 48 h in a nitrogen atmosphere. The optimal L-cysteine dosage was determined to be 0.6 g, according to the mercury adsorption capacity. The resulting solid was filtered out, sequentially washed with DMF, ethanol, and water, and then dried in an air atmosphere at 80 °C for 5 h. The final sample with an attached thiol group was yellow in colour and named SH-SBA-15 (thiol derivatized mesoporous silica). Scheme 1 shows the synthesis routes for SH-SBA-15.
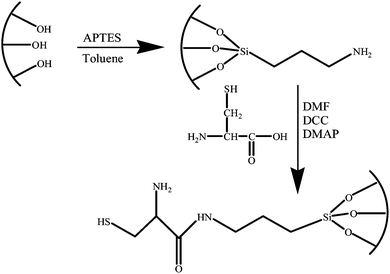 |
| Scheme 1 Synthesis routes for SH-SBA-15. | |
Mercury ion adsorption
Typically, 5 mg of the adsorbent was added to 20 mL of aqueous solutions containing mercury ions (5 mg L−1 to 900 mg L−1) and stirred at room temperature. The solution pH was adjusted with aqueous HNO3 and NH3·H2O. The mixture was then filtered, and the residual Hg(II) ions in the solution were measured by inductively coupled plasma-atomic emission spectroscopy (ICP-AES).
The mass of mercury adsorbed on the adsorbent was calculated based on the difference between the initial and final concentrations of the solution. The adsorption capacity (qe, in mg g−1) of the metal ions adsorbed onto SH-SBA-15 was calculated based on the following equation:
| 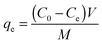 | (1) |
where
C0 and
Ce are the initial and the equilibrium concentrations of the metal ions in the testing solution (mg L
−1),
V is the volume of the testing solution (L), and
M is the weight of the adsorbent.
Characterization
Powder X-ray diffraction (XRD) patterns were collected on a Rigaku D/Max 2200PC diffractometer (Japan Rigaku Corporation, Japan) using Cu Kα radiation at 40 kV and 40 mA. Transmission electron microscopy (TEM) images were recorded using a JEOL 200CX Electron Microscope (JEOL, Japan) at 160 kV. N2 adsorption–desorption isotherms were measured at 77 K using a Micromeritics TriStar 3000 V6.05A analyzer (Micromeritics, USA). The sample was degassed at 300 °C for 5 h before measurements. Specific surface areas were calculated using the Brunauer–Emmett–Teller (BET) method within the relative pressure range of P/P0 = 0.05–0.3. The pore size distribution was obtained based on the Barrett–Joyner–Halenda (BJH) method. Pore volumes were obtained from the volumes of N2 adsorbed at P/P0 ≈ 0.99.
XPS was performed using a spectrometer (Thermo Scientific ESCALAB 250, Thermo Fisher Scientific, USA) with a monochromatized Al Kα X-ray source (1486.6 eV photons) at a constant dwelling time of 50 ms, a pass energy of 20 eV, and an anode current of 15 mA. C1s with 285 eV binding energy was used as the reference. Solid-state 13C MAS NMR spectra were recorded on a Varian VNMRS 400WB NMR spectrometer (400 MHz, Varian, USA). 13C CP/MAS NMR spectra were recorded at a contact time of 1 ms. 13C chemical shifts were externally referenced to tetramethylsilane at 0.0 ppm.
The chemical composition of water solutions was analyzed by ICP-AES (Varian Vista AX, USA). The pH was measured using a PHS-3E digital pH meter (Shanghai Precision and Scientific Instrument, China).
Results and discussion
Characterization of SH-SBA-15
XRD and TEM.
Fig. 1 shows the small-angle XRD patterns of the modified SBA-15 samples and unmodified SBA-15. Three resolved XRD diffraction peaks at 2θ = 0.5–2.5° that can be indexed to (100), (110), and (200) diffraction peaks corresponded to the typical pattern of P6mm symmetry. With increased functional groups, the intensities of (110) and (200) diffraction peaks became less intense,31 suggesting a reduction in mesoporous ordering. The TEM images of SH-SBA-15 (Fig. 2) confirmed its hexagonal structure with electron beams parallel and perpendicular to the pore channels. No large amorphous agglomerates were found in the sample. The modified materials maintain their well-ordered mesostructure after two-step modification.
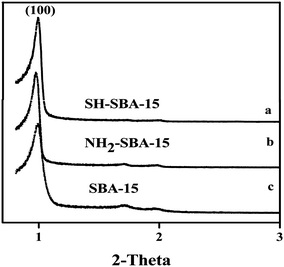 |
| Fig. 1 Small-angle XRD patterns of (a) SH-SBA-15, (b) NH2-SBA-15, and (c) unmodified SBA-15. | |
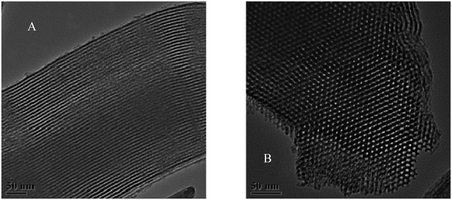 |
| Fig. 2 TEM images of SH-SBA-15 with electron beams parallel (A) and perpendicular (B) to the pore channels. | |
N2 adsorption–desorption isotherms.
Fig. 3 shows that the N2 adsorption–desorption isotherms of amine- and thiol-grafted SBA-15, as well as unmodified SBA-15, were type-IV isotherms. The adsorption and desorption branches exhibited parallel and vertical classified H1 loops in the SBA-15 adsorption isotherm, which were characteristic of materials with cylindrical pore geometry and high degree of pore size uniformity. However, the amino- and thiol-modified materials were inclined to H2 hysteresis loops considered to result from the presence of pores with narrow mouths (ink-bottle pores). The calculated specific surface area of these materials decreased from 363 m2 g−1 to 272 m2 g−1; the pore diameter calculated by the BJH method also decreased. Notably, the lower surface area and pore diameter of modified SBA-15 may result from the increase in organic groups. Their corresponding pore structural parameters are summarized in Table 1.
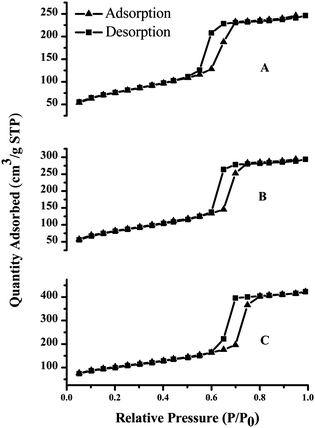 |
| Fig. 3 N2 adsorption–desorption isotherms of (A) SH-SBA-15, (B) NH2-SBA-15, and (C) SBA-15. | |
Table 1 Physical parameters of mesoporous silicas
Samples |
SBA-15 |
NH2-SBA-15 |
SH-SBA-15 |
a
0 is calculated using .
Wall thickness was calculated using the a0 minus BJH average pore diameter.
|
BET surface area (m2 g−1) |
363 |
299 |
272 |
Pore diameter (BJH) (Å) |
60.2 |
50.2 |
46.2 |
Pore volume (cm3 g−1) |
0.65 |
0.46 |
0.38 |
d(100) (Å) |
93.11 |
90.07 |
88.62 |
a
0
(Å) |
107.5 |
104.0 |
102.3 |
Wall thicknessb (Å) |
47.3 |
53.8 |
56.1 |
XPS and 13C CP/MAS NMR.
XPS spectra of SH-SBA-15 were obtained to determine its contents and confirm the introduction of thiol. The S fitting spectra of the SH-SBA-15 surface showed a peak at 163.7 eV (Fig. 4) and the same binding energy of S3/2 2p in standard L-cysteine.32 This finding strongly confirmed the presence of the SH group of L-cysteine on the surface of functionalized SBA-15. Moreover, the calculated content of thiol was 1.75%, and the L-cysteine was 6.62%.
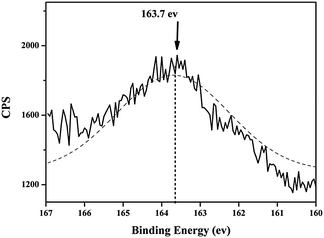 |
| Fig. 4 XPS spectrum of SH-SBA-15. | |
The successful incorporation of organic functional groups into an inorganic silica support was also confirmed by 13C CP/MAS NMR spectra (Fig. 5). The resonances at 11 and 23 ppm (24 ppm) were assigned to 1C and 2C of Si–1CH22CH23CH2–NH2 and Si–1CH22CH23CH2–NH4CO5CH(NH2)6CH2–SH, which corresponded to the methylene carbon directly bonded to the silicon atom and the other methylene carbons adjacent to 3C, respectively. The chemical shifts in 3C of NH2-SBA-15 and SH-SBA-15 at 45 and 41 ppm resulted from the change in the 3C chemical environment (transformation from 3CH2NH2 to 3CH2NH4CO). These chemical shifts well agreed with the aminopropylsilane-modified silica mentioned in previous studies.33,34 The signals at 175 ppm corresponding to the amide 4C from the condensation of the amino group with L-cysteine proved the successful second-step grafting. Furthermore, the resonance at 54 ppm belonged to the 6C (6C–S) from the incorporation of L-cysteine, which confirmed the introduction of L-cysteine.
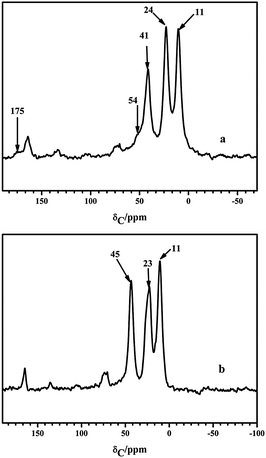 |
| Fig. 5
13C CP/MAS NMR spectrum of (a) SH-SBA-15 and (b) NH2-SBA-15. | |
Mercury adsorption study
Static adsorption capacity.
The solution pH, adsorption time, and Hg concentration were investigated to obtain the maximum adsorption capacity. The effect of solution pH on the adsorption ratio was studied within the pH range of 0.1 to 9.5. Results showed that a high mercury adsorption ratio of more than 95% was reached throughout the pH range. The SH-SBA-15 adsorbed mercury in different forms, i.e., Hg2+ and Hg(OH)+ in acidic medium (pH < 4), and Hg(OH)2 with increased pH to 4.35,36 pH 2.5 was selected to avoid mercury hydroxide precipitation and simplify the experimental procedure. Adsorption time is another important factor affecting mercury adsorption capacity. The range of 2 min to 2 h was studied, and results showed that the adsorption process was fairly rapid and almost reached equilibrium by SH-SBA-15 within 2 min at a low mercury concentration. However, the time of 1 h was selected considering the adsorption equilibrium at different mercury concentrations.
The experimental Hg adsorption capacity of SH-SBA-15 was 429 mg g−1 (calculated using eqn (1)) under the optimal conditions described above (Fig. 6). Moreover, the total amount of adsorbed mercury was ca. 2.1 mmol g−1 when the amount of grafted sulfur-containing groups was ca. 0.55 mmol g−1 (calculated by XPS). This finding indicated that SH-SBA-15 adsorbed an average of four mercury ions per attached ligand, which can be attributed to the stereo-coordination chemistry of S with Hg(II).18 The Hg(II) adsorption capacity was quantitatively compared with other materials, as shown in Table 2. The SH-SBA-15 materials exhibited remarkably high adsorption capability of Hg(II) ions. This value was higher than those of non-silicate materials functionalized with γ-glutamic acid and thiol,37,38 as well as those of thiol-functionalized mesoporous silicas described in the literature,39–42 except thiourea-functionalized mesoporous silicas. This exception can be attributed to the high thiourea and mercury complexation ability compared with thiol mercury.
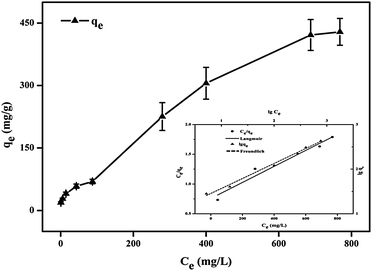 |
| Fig. 6 Mercury adsorption capacity of SH-SBA-15 [linear Langmuir (straight line) and Freundlich (dot line) plots in the inset figure]. | |
Table 2 Comparison of materials for mercury(II) adsorption
Adsorbent |
q
m (mg g−1) |
Material support |
Functional ligand |
Ref. |
SH-SBA-15 |
429 |
Silica |
Thiol |
This work |
γ-PGA |
96.79 |
Extracellular biopolymer |
γ-Glutamic acid |
37
|
Zn0.46Fe2.54O4 |
416 |
Zn-doped biomagnetite |
Thiol |
38
|
MP-HMS |
310 |
Silica |
Thiol |
39
|
SH-HMS |
140.10 |
Silica |
Thiol |
40
|
SH-PMOs |
64 |
Silica |
Thiol |
41
|
MCM-3T |
234.88 |
Silica |
Thiol |
42
|
MCM-41BTU |
1000 |
Silica |
Thiourea |
17
|
Two classical adsorption models, namely, Langmuir and Freundlich were used to describe Hg(II) ion adsorption onto the SH-SBA-15 surface. The Langmuir adsorption isotherm can be written as
|  | (2) |
The above equation can also be linearly expressed as
|  | (3) |
The Freundlich adsorption isotherm can be written as
|  | (4) |
The linear form can be represented by
| 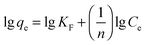 | (5) |
where
Ce (mg L
−1) is the equilibrium concentration of the solute,
qe (mg g
−1) is the equilibrium adsorption capacity of the adsorbent,
qm is the maximum adsorption capacity (mg g
−1), and
b,
KF (L g
−1), and
n are the Langmuir and Freundlich adsorption constants, respectively.
Langmuir isotherms are derived to describe the adsorption of an adsorbate on a homogeneous, flat surface of an adsorbent and monolayer adsorption onto a surface with a finite number of identical sites. Unlike the Langmuir model, the Freundlich model was used to describe the adsorption of an adsorbate onto the heterogeneous surface of an adsorbent. The values of these isotherm parameters are shown in Fig. 6 and summarized in Table 3. Results showed that the adsorption process well fitted the Freundlich model, as indicated by the very high correlation coefficient (R2 = 0.9928). These findings demonstrated the heterogeneous nature of Hg(II) binding on SH-SBA-15.
Table 3 Langmuir and Freundlich isotherm constants
Langmuir adsorption isotherm |
Freundlich adsorption isotherm |
q
max = 757.57 |
K
F = 10.01 |
b = 0.00132 |
n = 1.7655 |
R
2 = 0.9476 |
R
2 = 0.9928 |
Adsorption kinetics.
Fig. 7 shows the adsorption kinetics of Hg by SH-SBA-15. The SH-SBA-15 adsorbent was equilibrated with 20 mL of 10 mg L−1 Hg(II) solution for 2 min to 30 min to test the adsorption rate. Hg(II) adsorption initially increased rapidly and then tapered off in a few minutes. The maximum adsorbed Hg(II) on the SH-SBA-15 adsorbent was about 8 mg L−1. The adsorption almost reached equilibrium, and 7.5 mg L−1 was adsorbed in the first 2 min.
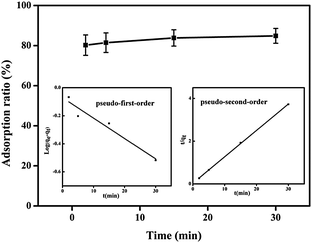 |
| Fig. 7 Uptake kinetics of SH-SBA-15 in an aqueous solution of Hg(II). | |
To understand the observed adsorption kinetics of Hg(II) ions on the SH-SBA-15 surface, the pseudo-first-order and pseudo-second-order kinetic models were used to generate the kinetic adsorption plots. The pseudo-first-order kinetic model can be written as follows:
| 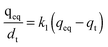 | (6) |
The linear expression can be represented as
| lg(qeq − qt) = lg qeq − k1t/2.303 | (7) |
The pseudo-second-order kinetic model can be written as follows:
| 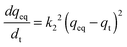 | (8) |
This equation can be linearly expressed as
| 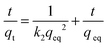 | (9) |
where
qt is the adsorption capacity at time
t (mg g
−1),
k1 (min
−1) is the rate constant of pseudo-first adsorption, and
k2 (g mg
−1 min
−1) is the rate constant of pseudo-second-order adsorption.
The pseudo-first-order kinetic model is based on the adsorption rate related to the number of unoccupied, adsorptive sites. The pseudo-second-order kinetic model is derived based on the notion that the adsorption should be related to the squared product of the difference between the number of equilibrium adsorptive sites available on an adsorbent and the number of occupied sites. Based on the Hg/S ratio, the equilibrium adsorptive sites available on SH-SBA-15 were higher than those of occupied sites, which indicated that adsorption well fitted the pseudo-second-order kinetic model. The correlation coefficient of the pseudo-second-order kinetic model was 0.9996, which agreed with the experimental data. Moreover, the qeq (cal) values (8.095) as obtained from the pseudo-second-order kinetic model were very close to the experimentally observed values (8.06) than the values (0.8483) obtained from the pseudo-first-order kinetic model. The rate constants k1, k2, and qeq, as well as the correlation coefficients, were calculated from linear plots and are listed in Table 4.
Table 4 Comparison of pseudo-first-order and pseudo-second-order constants
q
eq (exp) (mg g−1) |
Pseudo-first-order model |
Pseudo-second-order model |
k
1 (min−1) |
q
eq (cal) (mg g−1) |
R
2
|
k
2 (g mg−1 min−1) |
q
eq (cal) (mg g−1) |
R
2
|
8.06 |
0.03346 |
0.8483 |
0.9184 |
0.4489 |
8.095 |
0.9996 |
Selective adsorption of mercury.
Several conventional heavy metal ions [Hg(II), Cu(II), Cd(II), Cr(VI), Cr(III), and Pb(II)] in aqueous solution were selected as analytes. Single treatment with SH-SBA-15 reduced the Hg(II) concentration from 13.4 mg L−1 to 0.3 mg L−1 and the Cr concentration from 9.9 mg L−1 to 5.1 mg L−1. However, little or no affinity with other metal ions was observed. This phenomenon can be explained by the theory of hard and soft acids and bases. Softer transition metal ions are prone to forming stable complexes with ligands carrying softer donor atoms and vice versa.43 Sulfur is regarded as a soft donor atom that is inclined to combine with the soft metal Hg ion and showed low complexation affinity to other relatively hard metal ions (Cu, Cr, Pb, and Cd). Among these hard metal ions, Cr adsorption occurred in the adsorbent from hexavalent chromium at pH < 3.44Table 5 lists the ion concentrations before and after SH-SBA-15 treatment, distribution coefficient (Kd), and selectivity coefficient (αHg/M).
Table 5 Selective adsorption of Hg(II), Cu(II), Cra, Cd(II), and Pb(II) using SH-SBA-15
|
Cu(II) |
Cra |
Hg(II) |
Pb(II)b |
Cd(II) |
Chromium consisting of hexavalent and trivalent chromium.
Adsorption time = 60 min; pH of the Pb solution was adjusted to 1.5 and that of others was 2.5; dosage = 20 mg; temperature = 25 °C.
K
d (mL g−1) = (Ci − Ce)V/CeM, where Ci is the initial concentration (μg mL−1), Ce is the concentration after SH-SBA-15 treatment (μg mL−1), V is the volume of solution (mL), and M is the adsorbent mass (g).
.
|
No treatment (mg L−1) |
11.0 |
9.9 |
13.4 |
18.6 |
10.3 |
After SH-SBA-15 treatment (mg L−1) |
10.4 |
5.1 |
0.3 |
18.6 |
9.6 |
Partition coefficient Kdc |
55.5 |
905.0 |
41987.2 |
0 |
70.1 |
Selectivity coefficient αHg/Md |
756.5 |
46.4 |
— |
— |
599.0 |
Mild mercury desorption.
Mercury desorption studies were performed on the samples previously loaded with mercury. The following three solutions were investigated to identify the optimal desorption agent: 2 mol L−1 HCl, 8 mol L−1 HCl, and 5% thiourea (CS(NH2)2) in 1 mol L−1 aqueous HCl. The desorption ratios of Hg(II) ions were very high (up to 97%) when 5% thiourea in 1 mol L−1 of aqueous HCl was used as the desorption agent. However, the desorption ratios of 2 and 8 mol L−1 HCl achieved only 16% and 66%, respectively. The mixed solution was effective in recovering Hg(II) because of the double effects from HCl and thiourea. The increase in hydrogen ions from HCl improved desorption from 16% to 66%. Increasing the concentration of HCl to achieve a high desorption ratio was dominant considering the mild eluent. However, the N and S atoms of thiourea interacted with the adsorbed Hg(II) ions stronger than SH-SBA-15. As a result, the 5% thiourea in 1 mol L−1 aqueous HCl removed 97% mercury. Therefore, thiourea played the most important role in the elution process.
Conclusions
A thiol-functionalized organic–inorganic mesoporous composite was synthesized for the first time by post-grafting APTES and L-cysteine. The new composite materials possessed a well-ordered mesoporous structure. They were also highly selective for the adsorption of Hg(II) resulting from the soft acid of mercury by specifically binding with the soft base of thiol. The maximum adsorption capacity of 429 mg g−1 was reached for Hg(II) ions, and their adsorption time was less than 5 min. Adsorption well fitted the Freundlich model and the pseudo-second-order kinetic model, which indicated that the process may be chemical adsorption or chemisorption. SH-SBA-15 can be regenerated under mild conditions by washing with 5% thiourea in 1 mol L−1 HCl aqueous solution. Thus, the material can be a promising adsorbent for wastewater treatment or environmental material analysis.
Acknowledgements
This project was supported by National Natural Science Foundation of China (No. 21175145), the Science and Technology Commission of Shanghai Municipality (No. 09142201800) and (No. 13142201200).
Notes and references
- S. Chiarle, M. Ratto and M. Rovatti, Water Res., 2000, 34, 2971–2978 CrossRef CAS.
- D. Sevdic and H. Meider, Solvent. Extr. Ion Exch., 1990, 16, 487–504 Search PubMed.
- Z. Li, Q. Wei, R. Yuan, X. Zhou, H. Liu, H. Shan and Q. Song, Talanta, 2007, 71, 68–72 CrossRef CAS PubMed.
- M. M. Matlock, B. S. Howerton and D. A. Atwood, J. Hazard. Mater., 2001, B84, 73–82 CrossRef.
- D. M. Findlay and R. A. N. McLean, Environ. Sci. Technol., 1981, 15, 1388–1390 CrossRef CAS.
- M. E. Mahmoud, A. A. Yakout and M. M. Osman, J. Hazard. Mater., 2009, 164, 1036–1044 CrossRef CAS PubMed.
- I. Lagadic, M. Mitchell and B. H. Payne, Environ. Sci. Technol., 2001, 35, 984–990 CrossRef CAS.
- M. E. Paaez-Hernaandez, K. Aguilar-Arteaga, C. A. Galaan-Vidal, M. Palomar-Pardave, M. Romero-Romo and M. T. Ramiarez-Silva, Environ. Sci. Technol., 2005, 39, 7667–7670 CrossRef.
- Z. Zhu, X. Yang, L. He and W. Li, RSC Adv., 2012, 2, 1088–1095 RSC.
- L. Sun, Y. Li, M. Sun, H. G. Wang, S. F. Xu, C. Q. Zhang and Q. B. Yang, New J. Chem., 2011, 35, 2697–2704 RSC.
- P. Van Der Voort, D. Esquivel, E. De Canck, F. Goethals, I. Van Driessche and F. J. Romero-Salguero, Chem. Soc. Rev., 2013, 42, 3913–3955 RSC.
- Z. Wu and D. Zhao, Chem. Commun., 2011, 47, 3332–3338 RSC.
- X. Feng, G. E. Fryxell, L. Q. Wang, A. Y. Kim, J. Liu and K. M. Kemner, Science, 1997, 276, 923–926 CrossRef CAS.
- A. M. Liu, K. Hidajat, S. Kawi and D. Zhao, Chem. Commun., 2000, 1145–1146 RSC.
- G. Sánchez, D. Curiel, I. Ratera, A. Tárraga, J. Veciana and P. Molina, Dalton Trans., 2013, 42, 6318–6326 RSC.
- V. Antochshuk and M. Jaroniec, Chem. Commun., 2002, 258–259 RSC.
- V. Antochshuk, O. Olkhovyk, M. Jaroniec, I. S. Park and R. Ryoo, Langmuir, 2003, 19, 3031–3034 CrossRef CAS.
- L. Zhang, W. Zhang, J. Shi, Z. Hua, Y. Li and J. Yan, Chem. Commun., 2003, 210–211 RSC.
- J. Aguado, J. M. Arsuaga and A. Arencibia, Microporous Mesoporous Mater., 2008, 109, 513–524 CrossRef CAS PubMed.
- M. Mureseanu, A. Reiss and N. Cioatera, J. Hazard. Mater., 2010, 182, 197–203 CrossRef CAS PubMed.
- D. Perez-Quintanilla, I. Hierro del, M. Fajardo and I. Sierra, Microporous Mesoporous Mater., 2006, 89, 58–68 CrossRef CAS PubMed.
- P. J. Chiu, S. Vetrivel, A. S. T. Chiang and H. M. Kao, New J. Chem., 2011, 35, 489–494 RSC.
- Y. Wang, B. Li, L. Zhang, L. Liu, Q. Zuo and P. Li, New J. Chem., 2010, 34, 1946–1953 RSC.
- M. Mureseanu, A. Reiss, N. Cioatera, I. Trandafir and V. Hulea, J. Hazard. Mater., 2010, 182, 197–203 CrossRef CAS PubMed.
- B. Lee, Y. Kim, H. Lee and J. Yi, Microporous Mesoporous Mater., 2001, 50, 77–90 CrossRef CAS.
- J. Brown, R. Richer and L. Mercier, Microporous Mesoporous Mater., 2000, 37, 41–48 CrossRef CAS.
- Y. Liu, Y. Li and X. P. Yan, Adv. Funct. Mater., 2008, 18, 1536–1543 CrossRef CAS.
- M. L. Chen, H. J. Ma, S. Q. Zhang and J. H. Wang, J. Anal. At. Spectrom., 2011, 26, 613 RSC.
- D. Zhao, Q. S. Huo, J. L. Feng, B. F. Chmelka and G. D. Stucky, J. Am. Chem. Soc., 1998, 120, 6024–6036 CrossRef CAS.
- L. Zhang, C. Yu, W. Zhao, Z. Hua, H. Chen, L. Li and J. Shi, J. Non-Cryst. Solids, 2007, 353, 4055–4061 CrossRef CAS PubMed.
- A. Sayari and S. Hamoudi, Chem. Mater., 2001, 13, 3151–3168 CrossRef CAS.
- N. R. Urban, K. Ernst and S. Bernasconi, Geochim. Cosmochim. Acta, 1999, 63, 837–853 CrossRef CAS.
- Z. Gao, L. Wang, T. Qi, J. Chu and Y. Zhang, Colloids Surf., A, 2007, 304, 77–81 CrossRef CAS PubMed.
- A. M. Chong and X. Zhao, J. Phys. Chem. B, 2003, 107, 12650–12657 CrossRef CAS.
- H. J. Im, C. E. Barnes, S. Dai and Z. Xue, Microporous Mesoporous Mater., 2004, 70, 57–62 CrossRef CAS PubMed.
- A. Walcarius and C. Delacote, Anal. Chim. Acta, 2005, 547, 3–13 CrossRef CAS PubMed.
- B. S. Inbaraj, J. S. Wang, J. F. Lu, F. Y. Siao and B. H. Chen, Bioresour. Technol., 2009, 100, 200–207 CrossRef CAS PubMed.
- F. He, W. Wang, J. W. Moon, J. Howe, E. M. Pierce and L. Liang, ACS Appl. Mater. Interfaces, 2012, 4, 4373–4379 CAS.
- L. Mercier and T. J. Pinnavaia, Adv. Mater., 1997, 9, 500–503 CrossRef CAS.
- W. Shi, S. Tao, Y. Yu, Y. Wang and W. Ma, J. Mater. Chem., 2011, 21, 15567–15574 RSC.
- E. De Canck, L. Lapeire, J. De Clercq, F. Verpoort and P. Van Der Voort, Langmuir, 2010, 26, 10076–10083 CrossRef CAS PubMed.
- Q. Zou, L. Zou and H. Tian, J. Mater. Chem., 2011, 21, 14441–14447 RSC.
- R. G. Pearson, J. Am. Chem. Soc., 1963, 85(22), 3533 CrossRef CAS.
- Z. Wang, D. Fang, Q. Li, L. Zhang, R. Qian, Y. Zhu, H. Qu and Y. Du, Anal. Chim. Acta, 2012, 725, 81–86 CrossRef CAS PubMed.
|
This journal is © The Royal Society of Chemistry and the Centre National de la Recherche Scientifique 2014 |
Click here to see how this site uses Cookies. View our privacy policy here.