DOI:
10.1039/C3MD00164D
(Concise Article)
Med. Chem. Commun., 2014,
5, 20-24
Sucrose laurate-enhanced transcutaneous immunization with a solid-in-oil nanodispersion†
Received
12th June 2013
, Accepted 4th October 2013
First published on 8th October 2013
Abstract
A novel transcutaneous immunization system was developed using a solid-in-oil (S/O) nanodispersion, consisting of a nano-sized particle of a protein–surfactant complex dispersed in an oil vehicle. Permeability of the model antigen protein, ovalbumin, was enhanced by coating with sucrose laurate, which is a hydrophobic surfactant. The nanodispersion prepared with sucrose laurate induced a 5-fold increase in mouse antigen-specific antibody production compared with that observed using sucrose erucate, indicating that stability of the S/O nanodispersion in the epidermis plays a key role in enabling effective immunization. In spite of the coating with the surfactants, only fluorescence-labeled ovalbumin permeated the deep epidermis beneath the stratum corneum and was observed by laser scanning confocal microscopy. These findings will lead the way to an improved transcutaneous immunization system based on S/O nanodispersion.
Introduction
Since the introduction of vaccines into medical practice, they have served a crucial role in the prevention of infectious diseases. Injection is the most common method for the administration of vaccines, but needle-based immunization has a number of problems including needle phobia, needlestick injuries, and the transmission of pathogens through the reuse of needles and syringes.1
Needle-free immunization by oral, nasal, and transcutaneous routes is advantageous, avoiding both the above risks and the loss of efficacy resulting from first-pass metabolism. Hence, the development of needle-free delivery is becoming a global aim. In particular, the topical application of vaccine patches onto the skin is simple, does not require assistance from medical professionals, and will be suitable for mass administration when crises arise from viral pandemics or bio-terrorism.2,3 Moreover, one can expect effective immunization with a small amount of vaccine antigen, because the skin tissue is abundant in antigen-presenting cells, called Langerhans cells, with little protease expression.4 Nevertheless, the efficient delivery of hydrophilic antigen peptides or proteins through the skin remains challenging, because of the low permeability of the stratum corneum (SC), the outer layer of the skin, which is hydrophobic and acts as a strong barrier against chemical compounds and pathogens.
In our previous studies, an enhanced permeation of protein drugs through the skin was exhibited using a solid-in-oil (S/O) nanodispersion, an oil-based nanodispersion of hydrophilic proteins coated with hydrophobic surfactant molecules.5,6 Moreover, a S/O nanodispersion containing a model antigen protein, ovalbumin (OVA), effectively induced transcutaneous immunization, resulting in a high level of anti-OVA IgG production compared with that induced by a phosphate buffered saline (PBS) solution of the antigen, without skin pretreatment or an immune-stimulating adjuvant.7 Another study successfully demonstrated the transcutaneous perfusion of a large protein (molecular weight 66 kDa), bovine serum albumin (BSA), indicating the potentially powerful application of the S/O nanodispersion to medical and cosmetic uses.8 The S/O system does not require any special equipment or skin pretreatment, which are often required for other transcutaneous immunization systems, including those using microneedles, electroporation, and jet injection. Using the S/O nanodispersion system, the hydrophilic protein molecules can efficiently permeate into the hydrophobic SC layer by the assistance of the oil vehicle. In addition, we can prepare higher concentrations of protein dispersions compared to the emulsion-based vaccine carriers dispersed in oil vehicles, because the S/O nanodispersion is small and stable.
The previously mentioned reports used sucrose fatty acid esters or cholesterol as surfactants to prepare stable nano-sized particles, and isopropyl myristate (IPM) as an oil vehicle to facilitate skin permeation. In the present study, we focused on the type and the content of sucrose fatty acid esters used to induce enhanced immunization, with increased skin permeation efficiency. Moreover, the intercellular permeation pathway of the fluorescence-labeled antigen was observed using a confocal laser fluorescence microscope.
Results and discussion
Particle size and OVA-releasing efficiency
We used three lipophilic sucrose fatty acid esters that are soluble in cyclohexane (sucrose laurate (L-195, C12), sucrose oleate (O-170, C18), and sucrose erucate (ER-290, C22)) to examine the effect of carbon chain length of the surfactants, and the surfactant concentration on the stability and the release rate of the S/O nanodispersions. OVA, a glycoprotein of molecular weight 45 kDa, was used as the model antigen protein. Translucent dispersions of OVA–surfactant complexes in IPM were obtained using the surfactants (Fig. 1a), while, in contrast, a physical mixture of OVA and IPM formed a white precipitate. The mean particle sizes of the OVA–surfactant complexes were analyzed by dynamic light scattering, and were in the nano size range, with narrow dispersities (Table 1 and Fig. S1 in ESI†). The particle size was decreased when the surfactant concentration was increased. In water-in-oil (W/O) emulsions, particle size generally decreases with an increase in surfactant concentration.9 The sizes of the OVA–surfactant S/O particles clearly corresponded to the sizes of W/O emulsions just before lyophilization. The OVA-release efficiency of each S/O nanodispersion in PBS was then monitored for 24 h. The highest OVA release rate was observed when L-195 was used as a surfactant and the OVA–surfactant ratio was 1
:
50 (Fig. 1b). Similar to W/O emulsions in general, the S/O nanodispersion prepared with a lower concentration of surfactants with shorter carbon chains was rather unstable in the hydrophilic environment.9,10
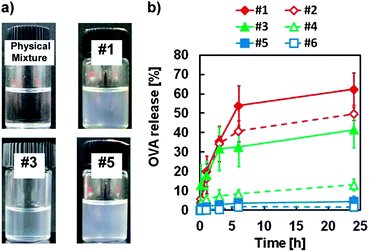 |
| Fig. 1 Physical appearance of a mixture of OVA in IPM and the S/O nanodispersions (a), and the OVA-release efficiency of the S/O nanodispersions in PBS (b). The data represent the mean ± SD of three experiments. | |
Table 1 Particle sizes and polydispersity indices (PDI) of the S/O nanodispersionsa
Sample no. |
Surfactant |
OVA–surfactant ratio |
Z-Average |
PDI |
Data represent the mean ± SD of three experiments.
|
#1 |
L-195 |
1 : 50 |
399 ± 86 |
0.08–0.20 |
#2 |
|
1 : 100 |
256 ± 30 |
0.04–0.22 |
#3 |
O-170 |
1 : 50 |
223 ± 17 |
0.31–0.57 |
#4 |
|
1 : 100 |
206 ± 14 |
0.16–0.47 |
#5 |
ER-290 |
1 : 50 |
204 ± 90 |
0.04–0.21 |
#6 |
|
1 : 100 |
165 ± 60 |
0.17–0.29 |
Penetration of the S/O nanodispersion into mouse epidermis
We compared the skin penetration efficiency, derived from their carbon chain length, of the surfactants L-195 and ER-290, using fluorescein isothiocyanate-labeled OVA (FITC-OVA). The FITC-OVA in PBS solution had not penetrated the mouse auricle skin 24 h after patch attachment, and little fluorescence was observed in the tissue section (Fig. S2 in ESI†). Meanwhile, the FITC-OVA encapsulated in the S/O nanodispersion penetrated the epidermis and dermis, and green fluorescence was observed. Luminance profiles as a function of distance from the skin surface indicate that FITC-OVA encapsulated in ER-290 accumulated in the SC layer (∼1.5 μm), and the FITC-OVA encapsulated in L-195 penetrated deep into the epidermis.
We added rhodamine-labeled dioleyl phosphatidyl choline (DOPE) to each surfactant at 0.05 wt%, and prepared S/O nanodispersions containing FITC-OVA. After the application of S/O nanodispersions consisting of L-195 to mouse auricles for 24 h, red fluorescence associated with rhodamine was observed to a greater extent at the skin surface (0 μm), whilst the green fluorescence of FITC was observed more deeply within the epidermis (∼8 μm), as shown in Fig. 2a. This observation supports a previous study using porcine skin in vitro, in which hydrophobic surfactants separated at a point between the SC and the deep epidermis, and only hydrophilic proteins penetrated into the hydrophilic space deep in the epidermis.6 In contrast, co-localization of red and green fluorescence was observed in the SC (∼2 μm) when a S/O nanodispersion consisting of ER-290 was applied, indicating that the ER-290–OVA complex was fixed, unseparated, in the SC (Fig. 2b). Fluorescence in surrounding cells was observed in the confocal microscopic images of both tissues, so apparently the intercellular space comprises the penetration pathway for the antigen–surfactant complexes.
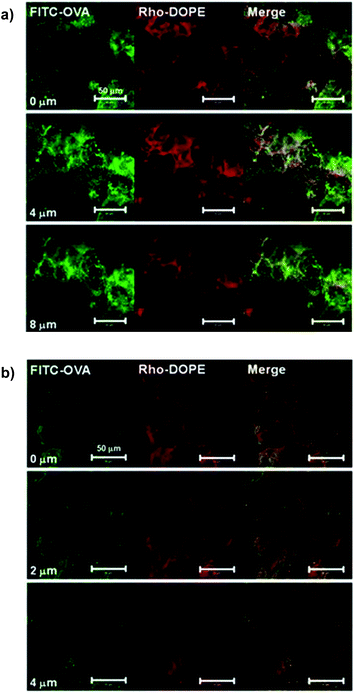 |
| Fig. 2 Confocal fluorescence images of mouse ear skin 24 h after application of the S/O nanodispersions prepared from L-195 (a) and ER-290 (b). Green and red fluorescence emissions represent FITC-OVA and rhodamine-DOPE, respectively. Scale bars = 50 μm. | |
Furthermore, we examined whether antigen capture by the Langerhans cells (LC) occurred in S/O-patched auricle skin.11 Co-localization of Cy3-OVA and LC was observed in confocal microscopic images of an epidermal sheet prepared from a mouse auricle, 24 h after applying the S/O nanodispersion patch (Fig. S3 in ESI†).12 In addition, flow cytometry analysis of the epidermal cells prepared by trypsin digestion of the epidermal sheet revealed that the proportion of OVA-capture LC was increased by the transcutaneous administration of the S/O nanodispersions (Fig. S4 in ESI†).
Transdermal immunization by OVA-loaded S/O
As shown in Fig. 3, encapsulation of OVA in the S/O nanodispersion induced anti-OVA IgG production in the ddY mouse following administration by the transcutaneous route. More importantly, the L-195 formula enhanced the induction of antigen-specific antibodies, and a decrease in surfactant concentration led to an increase in antigen-specific antibody levels (Fig. 3a). This is interesting because smaller-sized S/O nanodispersions are not markedly more effective at enhancing antigen production. The rapid release of OVA from the OVA–surfactant complex in the hydrophilic space in the epidermis caused an increase in the OVA-capture LC level, following induction of the OVA-specific immunological response. Circular dichroism spectra of the OVA released in PBS from the S/O nanodispersion was similar to that of native OVA, showing a positive peak at 197 nm and negative peaks at 207 and 222 nm,13 indicating that the encapsulation in the S/O complex did not affect the protein conformation (Fig. S5 in ESI†).14 Moreover, skin irritancy of the S/O particle was tested by a 3-(4,5-di-methylthiazol-2-yl)-2,5-diphenyltetrazolium bromide (MTT) assay using a three-dimensional culture model of human epidermal tissue, the LabCyte EPI-MODEL (Japan Tissue Engineering, Aichi, Japan).15 Consequently, sodium dodecyl sulfate showed 2.7% cell viability, while an OVA aqueous solution, a S/O nanodispersion of OVA, and IPM showed approximately 100% cell viability, exhibiting their low irritancy to the skin.
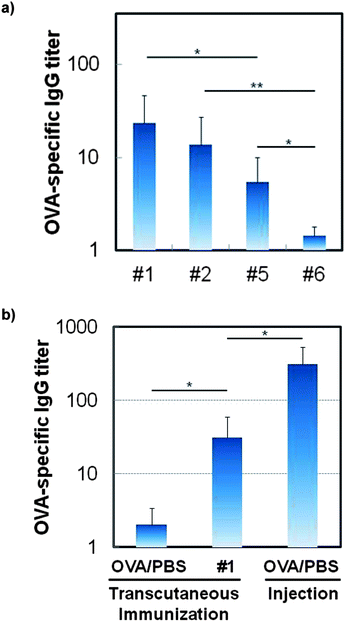 |
| Fig. 3 Mouse serum IgG responses to OVA after transcutaneous application of the S/O nanodispersions (a), and a comparison with a subcutaneous application (b). Data represent the mean ± SD of results from 5 to 11 mice. *p < 0.05, **p < 0.01. | |
Although the S/O complex was safe, and induced higher immune responses compared with an aqueous solution containing the same antigen, the antibody level produced by transcutaneous administration was still approximately one-tenth the antibody level produced by subcutaneous injection when the same amount of antigen was applied on the same schedule (Fig. 3b), implying that less antigen molecules were delivered to the immunocytes. To confirm this, we examined the effect of the number and the interval of boosters. The S/O system induced comparable levels of OVA-specific antibodies to those induced by subcutaneous injection when more than 2-fold of antigens were applied (Fig. S6 in ESI†). As previously demonstrated by using microneedles, electroporation, or jet injections, transcutaneous immunization has the possibility of inducing comparable levels of the specific antibody with a smaller amount of antigen compared to that used in subcutaneous injections.4 To exploit this advantage, the rapid release of the antigen from the S/O nanodispersion, and the targeted delivery of the antigen to the antigen-presenting cells, will be important issues for the practical application of the S/O nanodispersion system. Recent reports revealed the effects of oligoproteins and oligonucleotides on the enhanced permeability and targeted delivery of proteins to antigen-presenting cells in the epidermis.16 Encapsulation of antigens with these molecules may lead to an increased antibody induction with small amounts of antigens.
In conclusion, improved immunization was observed by using sucrose laurate as a surfactant within the S/O nanodispersion. Efficient release of the antigen from the S/O nanodispersion led to increased induction of the antigen-specific antibody. The S/O nanodispersion system is non-invasive and does not use special equipment. Transcutaneous immunization using oil-based nanodispersions of antigens thus has the potential for safe and easy vaccination.
Experimental section
Preparation of the S/O nanodispersions and particle size analysis
The S/O nanodispersions were prepared as previously described.12 Sucrose erucate (ER-290; Mitsubishi-Kagaku Foods, Tokyo, Japan), sucrose oleate (O-170; Mitsubishi-Kagaku Foods), or sucrose laurate (L-195; Mitsubishi-Kagaku Foods) were dissolved in cyclohexane to obtain a surfactant–cyclohexane solution (25 or 50 mg mL−1). One mL of an OVA (Sigma-Aldrich, St. Louis, MO, USA) aqueous solution (1.0 mg mL−1) and 2 mL of a surfactant–cyclohexane solution were mixed using a polytron homogenizer (Kinematica AG, Lucerne, Switzerland) at 26
000 rpm for 2 min to form a W/O emulsion. The emulsion was immersed in liquid nitrogen for 20 min, then lyophilized overnight with a freeze dryer (EYELA, Tokyo, Japan). To the resultant surfactant–OVA complex, isopropyl myristate (IPM; Tokyo Kasei, Tokyo, Japan) was added, and mixed thoroughly with a magnetic stirrer for 10 h. The OVA concentration in IPM was set at 1 mg mL−1. The size distribution of the nanodispersions was determined by dynamic light scattering with a Zetasizer NanoZS (Malvern, Worcestershire, UK). The viscosities and refractive indices were measured with an automatic microviscometer (Anton Parr GmbH, Graz, Austria) and with a refractive-index detector (RA-500, Kyoto Electronics Manufacturing, Kyoto, Japan), respectively.
For the fluorescence microscopic observation of S/O nanodispersions in the mouse auricle skin, S/O nanodispersions were prepared from FITC-labeled OVA (2.0 mg mL−1 in IPM), rhodamine-DOPE (0.1 mg mL−1 in IPM), and a surfactant (L-195 or ER-290, 100 mg mL−1 in IPM). See ESI† for the preparation of the FITC-OVA.
OVA-release study in PBS
The S/O nanodispersion (0.5 mL) and PBS (2 mL) were mixed at 37 °C with a magnetic stirrer. After 1, 3, 6 and 24 h, aliquots (20 μL) were withdrawn and the protein concentration in the water phase was analyzed using a bicinchoninic acid kit (Sigma-Aldrich) according to the manufacturer's protocol.
Transcutaneous immunization of mice
Animal experiments were approved by the Ethics Committee for Animal Experiments (Kyushu University) and carried out in accordance with the Guide for the Care and Use of Laboratory Animals (Science Council of Japan). Male 7-week-old ddY mice (Kyudo, Saga, Japan) of average weight 35–40 g were used for the immunization study.
A total of 100 μg of OVA was applied onto the intact auricle skin (50 μg on each ear) as previously described.7 Tissue paper impregnated with samples was patched onto each intact mouse ear for 24 h. For control experiments, mice were subcutaneously injected into the abdomen with 50 μL of a PBS solution containing OVA (2.0 mg mL−1). Samples were administered twice, 1 week apart, and sera were collected 1 week after the last administration.
Measurement of anti-OVA antibody titers
Specific anti-OVA IgG titers were determined using an enzyme-linked immunosorbent assay following a previously described method.7 To a 96-well polystyrene plate (Nunc MaxiSorp, Thermo Fisher Scientific, Kanagawa, Japan), 100 μL of an OVA aqueous solution (5.0 mg mL−1) was added per well and incubated overnight at 4 °C. Following five repeat washes of the plate with PBS-T (0.1% Tween-20 in PBS), 200 μL of PBS containing 2.0 wt% of BSA was added per well and incubated for 2 h at 37 °C. The plate was again washed five times with PBS-T, and then incubated for 2 h at 37 °C with serial dilutions of serum samples in PBS containing 2.0 wt% of BSA (20 μL per well). After washing the plate five times with PBS-T, 100 μL of a 20
000 dilution (in PBS containing 2.0 wt% of BSA) of horseradish peroxidase-labeled rabbit anti-mouse IgG (Rockland) was added per well and incubated for 2 h at 37 °C. Following five repeat washes with PBS-T, 3,3′,5,5′-tetramethyl benzidine solution (Sigma-Aldrich) was added to each well (100 μL). The colorimetric reaction was stopped after 30 min by adding 1 M hydrogen chloride (50 μL per well). Optical density (O.D.) at 450 nm was measured using a microplate reader (BioTek instruments, Winooski, VT, USA).
The antibody titers were defined as the inverse dilutions at which the O.D. of the serum obtained from the immunized mouse was equal to the O.D. of a 500-fold dilution of the serum obtained from a non-immunized mouse. The software package Prism-5 (GraphPad, San Diego, CA, USA) was used to evaluate approximate curves for titers.
Histology
Tissue paper impregnated with fluorescence-labeled S/O nanodispersions was patched onto the mouse auricle skin (50 μg each). After 24 h, the patches were removed and the auricle tissue was washed with 99% ethanol, following a wash with PBS. The auricle tissues were then immersed in 4% paraformaldehyde for 6 h, and mounted in Entellan New (Merc, Darmstadt, Germany) medium. Fluorescence images were observed using a confocal laser scanning microscope LSM 700, equipped with a 64× objective lens and the software ZEN 2011 (Carl Zeiss, Oberkochen, Germany). FITC and rhodamine were excited at 488 and 555 nm, respectively.
Statistical evaluation
Statistical significance was evaluated by Student's t-test using Prism-5 software. The data were expressed as the mean ± standard deviation of the mean.
Acknowledgements
This work was supported by a Grant-in-Aid for Scientific Research (S)24226019 from the Ministry of Education, Culture, Sports, Science, and Technology of Japan (to M. G. and N. K.). We thank Prof. Takuro Niidome and Prof. Yoshiki Katayama for their assistance with animal experiments.
Notes and references
- S. Mitragotri, Nat. Rev. Immunol., 2005, 5, 905 CrossRef CAS PubMed.
- D. T. O'Hagan and R. Rappuoli, Adv. Drug Delivery Rev., 2006, 58, 29 CrossRef CAS PubMed.
- Z. Shi, D. T. Curiel and D.-C. Tang, Vaccine, 1999, 17, 2136 CrossRef CAS.
- G. M. Glenn, D. N. Taylor, X. Li, S. Frankel, A. Montemarano and C. R. Alving, Nat. Med., 2000, 6, 1403 CrossRef CAS PubMed; D. Mishra, V. Dubey, A. Asthana, D. K. Saraf and N. K. Jain, Vaccine, 2006, 24, 4847 CrossRef PubMed; S. Frankenburg, I. Grinberg, Z. Bazak, L. Fingerut, J. Pitcovski, R. Gorodetsky, T. Peretz, R. M. Spira, Y. Skornik and R. S. Goldstein, Vaccine, 2007, 25, 4564 CrossRef PubMed.
- H. Piao, N. Kamiya, F. Cui and M. Goto, Int. J. Pharm., 2011, 420, 156 CrossRef CAS PubMed.
- Y. Tahara, S. Honda, N. Kamiya, H. Piao, A. Hirata, E. Hayakawa, T. Fujii and M. Goto, J. Controlled Release, 2008, 131, 14 CrossRef CAS PubMed.
- Y. Tahara, K. Namatsu, N. Kamiya, M. Hagimori, S. Kamiya, M. Arakawa and M. Goto, Chem. Commun., 2010, 46, 9200 RSC.
- M. Martins, N. G. Azoia, A. Ribeiro, U. Shimanovich, C. Silva and A. Cavaco-Paulo, Colloids Surf., B, 2013, 108, 271 CrossRef CAS PubMed.
- H. Yoshiura, M. Hashida, N. Kamiya and M. Goto, Int. J. Pharm., 2007, 338, 174 CrossRef CAS PubMed.
- H. Yoshiura, Y. Tahara, M. Hashida, N. Kamiya, A. Hirata, T. Fujii and M. Goto, Biochem. Eng. J., 2008, 41, 106 CrossRef CAS PubMed.
- Y. Ishii, T. Nakae, S. Nakagawa and N. Okada, J. Controlled Release, 2008, 131, 113 CrossRef CAS PubMed.
- I. C. Mackenzie and C. A. Squier, Br. J. Dermatol., 1975, 92, 523 CrossRef CAS.
- R. Townend, T. F. Kumosinski, S. N. Timasheff, G. D. Fasman and B. Davidson, Biochem. Biophys. Res. Commun., 1966, 23, 163 CrossRef CAS.
- H. Sah, J. Pharm. Sci., 1999, 88, 1320 CrossRef CAS PubMed.
- M. Katoh, F. Hamajima, T. Ogasawara and K. Hata, J. Toxicol. Sci., 2009, 34, 327 CrossRef CAS.
- Y. Tahara, S. Honda, N. Kamiya and M. Goto, Med. Chem. Commun., 2012, 3, 1496 RSC; J. B. Rothbard, S. Garlington, Q. Lin, T. Kirschberg, E. Kreider, P. L. McGrane, P. A. Wender and P. A. Khavari, Nat. Med., 2000, 6, 1253 CrossRef CAS PubMed; M. P. M. Schutze-Redelmeier, S. Kong, M. B. Bally and J. P. Dutz, Vaccine, 2004, 22, 1985 CrossRef PubMed.
Footnote |
† Electronic supplementary information (ESI) available: Detailed experimental methods and figures are provided. See DOI: 10.1039/c3md00164d |
|
This journal is © The Royal Society of Chemistry 2014 |