Enhanced luminescence of CaMoO4:Eu by core@shell formation and its hyperthermia study after hybrid formation with Fe3O4: cytotoxicity assessment on human liver cancer cells and mesenchymal stem cells†
Received
15th July 2013
, Accepted 19th October 2013
First published on 23rd October 2013
Abstract
Highly water dispersible Eu3+ doped CaMoO4 nanoparticles (core) covered by CaMoO4 (shell) have been prepared using the polyol method. Significant enhancement in luminescence intensity by core@shell formation is observed due to the decrease of non-radiative rate arising from surface/defect of particles. Effect of 266 nm laser excitation (Mo–O charge transfer band) on the asymmetric ratio (A21 = intensity ratio of electric to magnetic dipole transitions) has been studied and compared with a xenon lamp source. Luminescence intensity increases with the increase of power at 532 nm laser excitation. In order to explore materials, which can show dual functionalities such as luminescence as well as magnetic properties (magnetization of ∼14.2 emu g−1), water dispersible Fe3O4–CaMoO4:Eu hybrid magnetic nanoparticles (MN) have been prepared. This shows good heating ability up to ∼42 °C (hyperthermia) and luminescence in the red region (∼612 nm), which is in a biological window (optical imaging). Biocompatibility of the synthesized Fe3O4–CaMoO4:Eu hybrid magnetic nanoparticles has been evaluated in vitro by assessing their cytotoxicity on human liver cancer cells (HepG2 cells) and hTERT cells using the MTT assay and fluorescent microscopy studies.
Insight, innovation, integration
Highly water dispersible Eu3+ doped CaMoO4 (core) covered by inactive CaMoO4 (shell) nanoparticles have been successively prepared using the polyol method. Significant enhancement in luminescence intensity by core@shell formation was observed. Fe3O4–CaMoO4:Eu hybrid magnetic nanoparticles show both luminescence and magnetic behavior (saturation of magnetization ∼14.2 emu g−1). Furthermore, hybrid magnetic nanoparticles show good heating ability up to ∼42 °C (hyperthermia) and luminescence in the red region (∼612 nm), which is in a biological window (optical imaging). Biocompatibility of the hybrid magnetic nanoparticles has been evaluated in vitro by assessing their cytotoxicity on human liver cancer cells (HepG2 cells) and hTERT cells.
|
1. Introduction
In recent years, there has been enormous interest in the synthesis of rare-earth ion (Ln3+) doped core and core@shell nanoparticles due to their wide applications.1–18 These materials can be extensively used in optoelectronic and biological applications. Moreover, core@shell and/or hybrid nanomaterials having both magnetic and luminescence properties are used in magnetic resonance imaging (MRI), in vitro and in vivo bio-labelling, drug delivery and hyperthermia applications.19–21 In general, Fe3O4 magnetic nanoparticles (MN) are used in hyperthermia applications by coating the surface of nanoparticles using different surfactants, like, oleic acid (OA), dimercaptosuccinic acid (DMSA), chitosan (CS), O-carboxymethyl chitosan (OCMCS) and poly-ethylene glycol (PEG), etc.20,22–24 However, it is difficult to prepare material, which can show luminescence as well as magnetic properties. Generally, luminescence intensity decreases with an increase of ferromagnetic species in the material.1 For this, it is necessary to optimize the concentration of magnetic and luminescence materials to be used. Common techniques are (i) formation of the shell of Ln3+ doped inorganic host on a surface modified Fe3O4 nanoparticles (core@shell structures), (ii) mixing of proper amounts of Fe3O4 nanoparticles with inorganic phosphor nanomaterials (hybrid structures) and (iii) a MN-inert shell/organic ligand–phosphor system (e.g., liposome/vesicle in which MN is in the core (water dispersible) and phosphor is in the lipid layer (oil dispersible). Recently, some nanocomposites such as Fe3O4@Y2O3:Eu3+,25 Fe3O4@YVO4:Eu3+,26 Fe3O4@YPO4:Eu/Tb3+,27etc. were prepared and their luminescence, magnetic and induction heating properties were studied. The methods used for the preparation of such nanoparticles needed a long time, and particles were inhomogeneous in shape. Usually, Fe3O4 magnetic nanoparticles were prepared by a co-precipitation method, and these were used for a hyperthermia application due to their high biocompatibility.23 In particular, nanoparticles with surface functionalization are more useful in biological applications.28–30 Gd based nanoparticles are used for cancer therapy through hyperthermia as well as a contrast agent in MRI.31
In most cases, CaMoO4:Eu particles are not dispersible in a polar medium. This makes them less suitable for biological and polymer based display applications. In order to make them dispersible, there are a few ways: (i) particle size should be <50 nm and (ii) surface functionalization. Enhanced luminescence intensity for dispersed particles is important, and thus it can be achieved by use of a functionalized ligand having a lower number of O–H and C–H and core@shell formations. Luminescence intensity is also dependent on the type of solvent where nanoparticles are dispersed, excitation wavelength and power density. These are not discussed much in the literature. In this paper, significant enhancement in luminescence of Eu3+ doped CaMoO4 nanoparticles (core) is achieved by forming inactive CaMoO4 (shell) on the surface of the core. The power density dependent luminescence using a 532 nm Nd:YAG laser is studied so that the desired luminescence intensity can be obtained. Fe3O4@CaMoO4:Eu hybrid nanoparticles are prepared for hyperthermia applications. To the best of our knowledge, for the first time these hybrid nanoparticles with excellent dispersibility, heating ability up to ∼42 °C and luminescence properties have been prepared. Bio-compatibility of hybrid nanoparticles on cancerous and normal cells was studied.
2. Experimental section
2.1 Preparation
2.1.2 Preparation of Fe3O4 magnetic nanoparticles.
The superparamagnetic Fe3O4 nanoparticles (Fe3O4-MN) were prepared using the co-precipitation method discussed earlier.22 Fe3O4-MN nanoparticles were prepared by ferric chloride (FeCl3·6H2O) and ferrous sulphate (FeSO4·7H2O) in a NH3 medium. In order to prepare dispersible particles in water, 4 g of PEG was dissolved in 50 mL of distilled water and treated with the Fe3O4-MN. It was kept for ultrasonication for 30 min to get homogeneous mixing.
2.1.3 Preparation of hybrid MN (Fe3O4–CaMoO4:Eu).
For the preparation of the Fe3O4–CaMoO4:Eu hybrid MN (1
:
4 mole ratio), 28.94 mg of Fe3O4 and 100 mg CaMoO4:Eu were dispersed in 4 mL of distilled water containing 1 g of polyethylene glycol (PEG) and followed by ultrasonication for 30 min. The resulting hybrid nanoparticles dispersed in PEG were used for hyperthermia experiments. The hybrid nanoparticles were collected after centrifugation and allowed to dry in an ambient atmosphere for 4 days. This powder was used to record luminescence of the sample.
2.1.4 Culture and maintenance of HepG2 and hTERT cell lines.
The HepG2 hepatoblastoma cells and hTERT human mesenchymal cells were maintained between 10% and 90% confluency and propagated in 90% Eagle's Minimal Essential Medium (EMEM) (ATCC) and RPMI 1640, respectively. Media were supplemented with 10% fetal bovine serum (FBS) (ATCC) and 1% penicillin/streptomycin (10
000 I.U mL−1–10
000 μg mL−1) (ATCC). Cells were cultured as adherent monolayers (i.e., cultured at ∼70% to 80% confluence) and maintained at 37 °C in a humidified atmosphere of 5% CO2. Cells were harvested after brief trypsinization.
2.1.5 MTT assay.
Cytotoxicity was determined by the yellow 3-(4,5-dimethyl thiazol-2-yl)-2,5-diphenyltetrazolium bromide (MTT) assay.32 The MTT assay is based on the ability of viable cells to reduce soluble MTT into an insoluble, blue formazan product. In brief, around 105 cells per well were plated in 96-well microtiter plates in a 100 μL of EMEM medium. After 24 h the medium was changed, and cells were treated with Fe3O4–CaMoO4:Eu hybrid magnetic nanoparticles at different concentrations (0, 5, 10, 20, 40, 80, 160, 320, 640 μg mL−1) for 24 h. After this, the medium was aspirated off and 100 μL MTT (0.5 mg mL−1) was added and incubated for 4 h. The liquid from each well was aspirated and discarded. The formazan crystals formed during incubation with MTT was solubilised by adding 100 mL of dimethyl sulfoxide (DMSO) to each well. The plates were placed on a shaker for 15 min for complete solubilization of crystals, and the optical density of each well was determined. The quantity of formazan product as measured by the amount of 545 nm absorbance is directly proportional to the number of living cells in culture. The cell viability (%) related to control wells containing cell culture medium without Fe3O4–CaMoO4:Eu hybrid magnetic nanoparticles as a vehicle was calculated by: | Viability (%) = [A]test/[A]control × 100 | (1) |
where [A]test is the absorbance of the test sample and [A]control is the absorbance of control sample. IC50 was also calculated. Triplicate experiments were carried out.
To examine the morphological changes in the nucleus and the cytoplasm of cells after addition of Fe3O4–CaMoO4:Eu hybrid magnetic nanoparticles into HepG2 cells and hTERT, fluorescent microscopy was performed. The HepG2 and hTERT cells, approximately 1 × 105, were cultured in 6 well plates in a medium made up of EMEM and treated with IC50 and the highest concentration (640 μg mL−1) of Fe3O4–CaMoO4:Eu hybrid magnetic nanoparticles, respectively, for 24 h with technical triplicates. Untreated control and treated cells were observed under an inverted microscope (Carl Zeiss, Deutschland). For each experiment, nuclei from 10 random fields of wells were examined at 200 times magnification. DAPI, a fluorescent dye was used for examining the treatment of HepG2 cells with Fe3O4–CaMoO4:Eu hybrid magnetic nanoparticles.
4′-6-Diamidino-2-phenylindole (DAPI) is known to form fluorescent complexes with natural double-stranded DNA, showing fluorescence specificity for adenine–thymine (AT), and adenine–uracil (AU) clusters. Because of this property, DAPI is a useful tool in various cytochemical investigations. When DAPI binds to DNA, its fluorescence is strongly enhanced. Also, there is also evidence that DAPI binds to the minor groove, stabilized by hydrogen bonds between DAPI and acceptor groups of AT and AU base pairs. The membrane permeable fluorescent dye DAPI (2 μg mL−1) (Sigma Aldrich), which binds to the chromatin of cells, was added to the fixed cells.
2.2 Characterization of magnetic and luminescence nanoparticles
X-ray diffraction (XRD) patterns of the powder samples were examined at room temperature using a PANalytical X'Pert X-ray diffractometer equipped with Ni filtered Cu Kα (λ = 1.54056 Å) radiation as the X-ray source. Raman spectra was recorded on a Jobin Yvon Horiba HR800, UV Raman microscope using a HeNe laser emitting at 632.8 nm. Excitation and emission spectra of all the samples were recorded using an Edinburgh Instrument FLS920 having 450 W xenon lamp. The emission spectra were also recorded using 266 nm excitation from a Nd:YAG laser and 532 nm excitation from laser (Verdi V5). A Horiba Jobin Yvon iHR320 spectrometer was used to detect the luminescence signal.
Induction heating of hybrid magnetic nanoparticles was performed in a 1.5 mL micro centrifuge tube, which was placed at the centre of a 6 cm diameter copper coil coated with Teflon® (Easy Heat 8310, Ambrell, UK). In a typical measurement, 5 mg of hybrid nanoparticles were dispersed in 1 mL of distilled water, which was inside a 1.5 mL microcentrifuge tube. AC magnetic field at different currents and fixed at 265 kHz was applied. Heat generated within the sample was recorded using an optical temperature sensor. Temperature was measured with an accuracy of ±0.01 °C. The resultant magnetic field at the centre of the 6 cm copper coil was calculated using the following equation:
|  | (2) |
where
H is the magnetic field (measured in Oe),
n, number of turns in a coil,
i, the applied current and
L, the diameter of a turn in cm.
H values are found to be 168, 251 and 335 Oe (equivalent to 13, 20 and 27 kA m
−1) for 200, 300 and 400 A applied currents, respectively. The room temperature magnetizations of these samples were determined using a Vibrating Sample Magnetometer (VSM).
3. Results and discussion
3.1 XRD and TEM studies
Crystal structures of as-prepared core (3 at% Eu3+ doped CaMoO4) and core@shell (shell: CaMoO4) nanoparticles have been examined by an X-ray diffraction (XRD) method. Fig. 1 shows the XRD patterns of core and core@shell samples. As-prepared samples are well crystalline with tetragonal structure, and all peaks are matched with JCPDS card no. 29-0351. No extra impurity phases are detected. The standard diffraction pattern peaks are indexed to data with corresponding (hkl) planes between 15–80°. The average crystallite sizes calculated using Scherrer equation for as-prepared core and core@shell nanoparticles are found to be 24 and 27 nm, respectively. The unit cell parameters are found to be a = 5.219 Å, c = 11.439 Å and V = 311.60 Å3 for core and a = 5.219 Å, c = 11.439 Å and V = 311.62 Å3 for core@shell nanoparticles. These parameters are well matched with JCPDS card no. 29-0351 (a = 5.226 Å, c = 11.430 Å and V = 312.1 Å3). There is no significant change in lattice parameters and XRD patterns when an active core (Eu3+ doped) is covered with inactive shell (Eu3+ un-doped), i.e., CaMoO4:Eu3+@CaMoO4. The micro-strain that is acting on both core and core@shell nanoparticles was analysed by the Williamson–Hall method.3,33 The Williamson–Hall equation can be represented as shown below: | 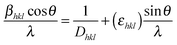 | (3) |
where βhkl is the full width at half maxima (FWHM) of XRD patterns, θ is the diffraction angle, λ is the wavelength of the X-rays, Dhkl is the effective crystallite size and εhkl is the micro-strain. The instrumental broadening (εinst) is removed by using the Si standard, discussed in our earlier report.4Fig. 1(b and c) shows the plot between (βhklcos(θ))/λ and sin(θ)/λ for the as-prepared core and core@shell samples. It is observed that the (βhklcos (θ))/λ value increases with sin(θ)/λ. The data points are fitted with a linear equation. The micro-strain (εhkl) and effective crystallite (Dhkl) sizes calculated are shown in the figure itself. The average effective crystallite sizes calculated from the Williamson–Hall equation are 32 and 35 nm for core and core–shell samples, respectively, which are slightly more than those of Scherrer equation. However, we do not find any significant difference in εhkl (0.003) calculated for both core and core@shell within the span of the error bar. The effective crystallite size of the core@shell sample is slightly higher than a core sample. Positive slope values (εhkl) for both core and core@shell indicates tensile strain acting on the particle. Patra et al. recently reported tensile strain (positive slope) for SnO2 nanoparticles and compressive strain (negative slope) for nanorods.34 Sharma and co-workers recently reported a variation in strain (0.001–0.03) in Dy3+ doped CaMoO4. It is found that strain almost increases with an increase in Dy3+ concentration, and it is relaxed by co-doping of K+ ions in CaMoO4.35 TEM images of CaMoO4:Eu3+@CaMoO4 and Fe3O4–CaMoO4:Eu are shown in Fig. 2. The particles are found to be spherical and sizes of 30–40 nm are observed.
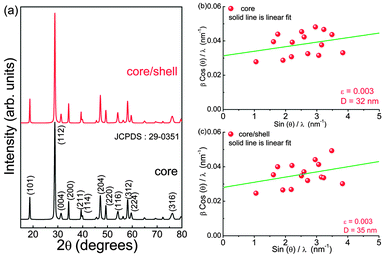 |
| Fig. 1 (a) XRD patterns of the core (3 at% Eu3+ doped CaMoO4) and core@shell (shell: CaMoO4) as-prepared nanoparticles. The (hkl) planes are represented in the figure itself. The Williamson–Hall plots for the core (b) and core@shell (c) samples. Solid green line represents the linear fit to the experimental data. The micro-strain (εhkl) and effective crystallite sizes (Dhkl) are shown in the corresponding figures itself. | |
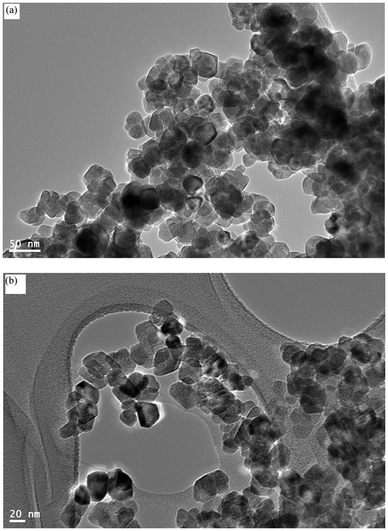 |
| Fig. 2 TEM images of (a) CaMoO4:Eu3+@CaMoO4 and (b) Fe3O3–CaMoO4:Eu. | |
3.2 Raman study
Fig. 3 shows the Raman scattering spectra of the core (3 at% Eu3+ doped CaMoO4) and core@shell (shell: CaMoO4) as-prepared nanoparticles recorded at room temperature. The Scheelite structure shows 26 modes of vibrations (3Ag, 5Au, 5Bg, 3Bu, 5Eg, 5Eu) in which 13 modes are Raman active (3Ag, 5Bg, 5Eg) and (4Au, 4Eu) are IR active.36,37 The Fourier transform infrared (FTIR) spectra of the core are discussed in our earlier study.4 The observed Raman peaks are given in Table 1 and compared with the reported values. Among all Raman peaks, the peak at ∼876 cm−1 shows the highest intensity having (full width at half maximum) FWHM ∼12 cm−1. The four Raman bands below 267 cm−1 are due to external modes (lattice modes) whereas the five bands below 910 cm−1 correspond to internal/optical modes (within MoO42−).38 We do not find significant changes in Raman peak positions of the core sample as compared to the core@shell sample. The ratio of integrated intensities of external mode (199 cm−1) to internal mode (320 cm−1) is found to be 0.42 and 0.46 for core and core@shell samples, respectively. The Raman spectra of both core and core@shell samples are normalised to their maximum peak intensity at 876 cm−1. It is found that the Raman modes at ∼320, 389 and 791 cm−1 show a significant change in emission intensity as compared to other Raman modes. On clear observation, the integrated intensity of the ∼320 cm−1 peak for the core sample is ∼1.1 times that of the core@shell sample and peaks are symmetric with Lorentzian fitting. In the case of the ∼791 cm−1 peak, the intensity of the core sample is 0.98 times that of the core@shell sample.
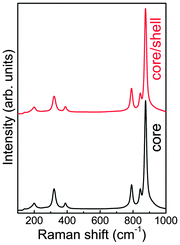 |
| Fig. 3 Raman spectra of core (3 at% Eu3+ doped CaMoO4) and core–shell (shell: CaMoO4) as-prepared nanoparticles. | |
Table 1 The Raman peak assignment of the as-prepared core and core@shell nanoparticles
Raman modes |
Observed peak position (cm−1) |
Reported peak position (cm−1) |
Core |
Core@shell |
Ref. 36
|
Ref. 38
|
Bg |
— |
86 |
83 |
— |
Eg |
115 |
112 |
110 |
111.5 |
Eg |
141 |
141 |
141 |
143 |
Eg |
199 |
199 |
201 |
189.5 |
Ag |
320 |
321 |
322 |
321.5 |
Bg |
389 |
390 |
390 |
391 |
Eg |
791 |
791 |
793 |
792 |
Bg |
844 |
844 |
845 |
845 |
Ag |
876 |
876 |
878 |
877 |
3.3 Luminescence study
3.3.1 Emission and excitation studies.
Fig. 4 shows the excitation spectra of the as-prepared 3 at% Eu3+ doped CaMoO4 core@shell nanoparticles by monitoring emission at ∼612 nm. A xenon lamp (450 W) was used as the excitation source. The broad absorption peak appeared in between 220 to 350 nm having a peak maximum at ∼300 nm and the full width at half maximum (FWHM) of ∼37 nm is assigned to O → Mo charge transfer band (Mo–O CTB).7,39 The Mo–O CTB is asymmetric. After deconvolution, two peaks were found at 275 and 301 nm. The former is related to the Eu–O CTB band and the latter one is related to Mo–O CTB. The two sharp peaks at ∼394 and 464 nm can be assigned to the 7F0 → 5L6 and 7F0 → 5D2 transitions of Eu3+, respectively.4 Typically, the intensity of Mo–O CTB is ∼228 times stronger than that of the 7F0 → 5L6 transition. The formation of a shell over a core significantly enhances the absorption intensity of Mo–O CTB.
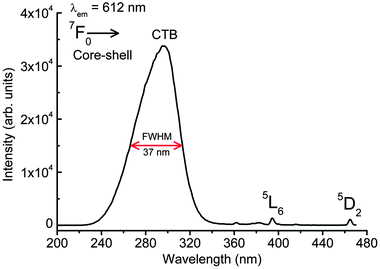 |
| Fig. 4 Excitation spectrum of the as-prepared 3 at% Eu3+ doped CaMoO4 core–shell nanoparticles by monitoring emission at 612 nm. Excitation source is from a xenon lamp (450 W). | |
Fig. 5(a) shows the emission spectra of Eu3+ doped CaMoO4 (Eu3+ = 1, 3, 5, 7, 10, 15, 20 and 30 at%) as-prepared samples. The excitation wavelength is 266 nm (corresponding to Eu–O CTB) with a Nd-YAG laser. It consists of four characteristic emission peaks at 591, 612, 652 and 700 nm which are assigned to the 5D0 → 7F1, 5D0 → 7F2, 5D0 → 7F3 and 5D0 → 7F4 transitions of the Eu3+ ion, respectively.40 The highest intensity peak is found at 612 nm (5D0 → 7F2), and this can be assigned to the electric dipole allowed transition (Δj = 2). The transition observed at 591 nm (5D0 → 7F1) is due to the magnetic dipole allowed transition of the Eu3+ ion (Δj = 1). The electric dipole transition 5D0 → 7F2 is hypersensitive whereas magnetic dipole transition 5D0 → 7F1 is insensitive to the crystal field environment around the Eu3+ ion. Magnetic dipole transition is dominant when Eu3+ ions occupy the site with inversion symmetry. In the other case electric dipole transition will be dominating according to Judd–Ofelt theory.41 Based on the crystal structure of CaMO4 (tetragonal structure with space group I41/a), the Eu3+ ion has 8 O2− ions to form a EuO8 polyhedron, in which there are two different Eu–O bond lengths and therefore, EuO8 is asymmetric. It is expected that the luminescence intensity for the electric dipole transition will be more than that of the magnetic dipole transition.42 The change in Ca/Eu–O bond length with Eu3+ ion dopant concentration was confirmed by Rietveld refinement of the XRD data in our earlier work.4 The luminescence intensity of the transition/curve is calculated after fitting with the Gaussian distribution function
| 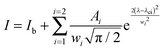 | (4) |
where
I is the intensity,
Ib is the background intensity,
wi is the FWHM of the curve,
Ai is the area under the curve,
λ is the wavelength and
λci is the mean value corresponding to the transition. These are fitted in the range 583–600 and 600–628 nm for the
5D
0 →
7F
1 and
5D
0 →
7F
2 transitions, respectively. A typical fitting to the electric and magnetic dipole transitions data for the as-prepared 1 at% Eu
3+ doped CaMoO
4 is shown in Fig. S1 (see ESI
†). The fitting has been carried out after the baseline correction (
Ib = 0). The parameters obtained after fittings are given in the figure itself with goodness of a parameter (
χ2 = 0.99205). In the case of the as-prepared samples, the integrated luminescence intensity of the electric dipole transition
5D
0 →
7F
2 (
A2) decreases with the increase of Eu
3+ concentration due to concentration quenching effect (
Fig. 6a). The variation of FWHM (
w2) with Eu
3+ concentration is shown in
Fig. 6b. The
w2 increases with Eu
3+ concentrations up to 10 at%.
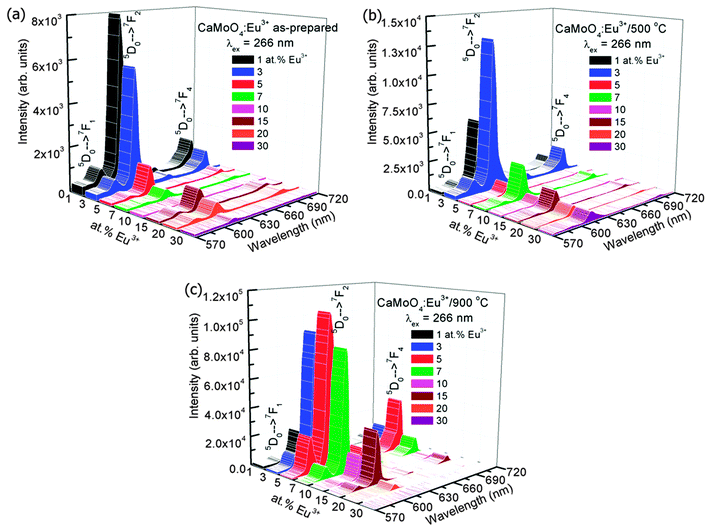 |
| Fig. 5 Emission spectra of Eu3+ doped CaMoO4 (Eu3+ = 1, 3, 5, 7, 10, 15, 20 and 30 at%) under 266 nm excitation of (a) as-prepared, (b) 500 and (c) 900 °C annealed nanoparticles. Excitation source is from a Nd-YAG laser. | |
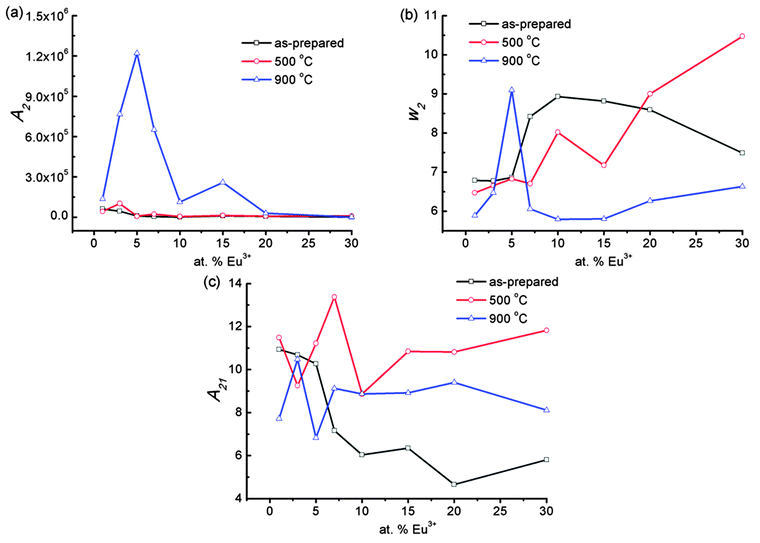 |
| Fig. 6 (a) Integrated area of the 5D0 → 7F2 transition (∫5D0 → 7F2) and (b) the corresponding FWHM of Eu3+ doped CaMoO4 (Eu3+ = 1, 3, 5, 7, 10, 15, 20 and 30 at%) under 266 nm excitation of as-prepared, 500 and 900 °C annealed nanoparticles. (c) Their corresponding asymmetric ratio (A21 = ∫5D0 → 7F2/∫5D0 → 7F1). Excitation source is from a Nd-YAG laser. | |
The structural distortion around the Eu3+ ion is estimated by the ratio of an integrated area of electric dipole transition to magnetic dipole transition, which is known as the asymmetric ratio (A21):
|  | (5) |
The
A21 of as-prepared sample decreases from 11 to 5.6 with an increase of Eu
3+ concentration up to 30 at% (
Fig. 6c).
The emission spectra of samples annealed at 500 °C after excitation at 266 nm is shown in Fig. 5b. The A2 increases as Eu3+ concentration increases from 1 to 3 at% and then decreases significantly with increasing Eu3+ concentration. The optimum intensity is more than that of the as-prepared sample due to the extent of reduction of non-radiative channels on annealing (Fig. 6a). The w2 increases with increasing Eu3+ concentration (Fig. 6b). The A21 is found to be in range of 8.8–13.3 (Fig. 6c).
The samples annealed at 900 °C show intense emission on excitation at 266 nm (Fig. 5c). The A2 value increases as Eu3+ concentration increases from 1 to 5 at% and then decreases with a further increase of Eu3+ concentration (Fig. 6a) and is more than that of the as-prepared and 500 °C annealed samples due to the extent of reduction of a non-radiative process with annealing. The surface of the particles is contributing to a non-radiative probability. In the as-prepared samples, particle size is small and the surface has H2O and EG, which act as a quencher for luminescence. When the samples are annealed at 500 °C, the decomposition of EG occurs. Most carbon impurities and H2O are removed. Furthermore, the particle size increases. This gives an enhancement in luminescence intensity. A significant improvement in luminescence can be observed for the 900 °C annealed sample because the quenching centres H2O and surface dangling bonds are minimum (Fig. 5c). The w2 increases initially up to 5 at% Eu3+ and decreases with increasing Eu3+ concentration (Fig. 6b) and is less than that of the as-prepared or 500 °C annealed sample. The A21 is found to be in range of ∼7.7–10.5 (Fig. 6c), which is still quite high. Reported A21 values are found to be in the range of ∼7.4–7.9 on excitation to Mo–O/Eu–O CTB in Eu3+ doped CaMoO4 (260 nm, xenon lamp excitation).43 We also found A21 values to be in the range of 7–9 under xenon lamp excitation of 150 W.39 A higher asymmetric ratio indicates the increase of occupancy of Eu3+ in Ca2+ sites without inversion symmetry. In addition, Raju and co-workers7 reported a high asymmetric ratio ∼10 (CaMoO4:Eu). This may be due to the polarization effect of the MoO4 cluster in the CaMoO4 host.39 To understand the effect of pump power on A21, 900 °C annealed 3 at% Eu3+ doped CaMoO4 samples were excited with 532 nm laser excitation (5D1 level, Fig. 7a).
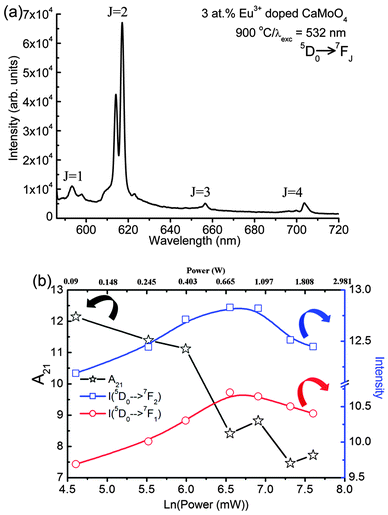 |
| Fig. 7 (a) Typical emission spectrum of 900 °C annealed 3 at% Eu3+ doped CaMoO4 nanoparticles under 532 nm laser excitation, (b) variation in A21, electric and magnetic dipole transition intensities of 900 °C annealed 3 at% Eu3+ doped CaMoO4 nanoparticles with different powers (P) of a 532 nm laser. | |
Fig. 7b shows the variation in A21, integrated intensity of electric and magnetic dipole transitions at different pump powers of a 532 nm laser. The A21 value decreases from 12 to 7 as the power increases from 0.1 to 2 W, whereas the integrated intensity of the peaks increases up to an optimum power of 1 W. The decrease in emission intensity at a higher power density above 1 W may be due to temperature generated in the sample. An increase in temperature in the sample will increase the fluctuation in luminescence intensity. However, the variation of A21 values with the intensity of excitation photons has not been reported to the best of authors' knowledge.
Fig. 8(a and b) shows the emission spectra of the as-prepared 3 at% Eu3+ doped CaMoO4 core and core@shell nanoparticles under different excitation wavelengths (Xe-lamp source, 450 W). Here the shell is inactive (CaMoO4). Core nanoparticles show optimum emission near 266 nm excitation whereas the core@shell nanoparticles show optimum emission at ∼300 nm excitation. This is due to a shift of Mo–O CTB to a higher wavelength region. A similar observation of an increase of the V–O CTB (CTB = charge transfer band) to the higher wavelength after core–shell formation (core: YVO4:Eu and core–shell: YVO4:Eu@YVO4) have been reported.44 The luminescence spectra of core and core@shell nanoparticles show similar nature between 570–700 nm having a strong emission peak at ∼615 nm (5D0 → 7F2). Typical emission intensity of core@shell nanoparticles at 300 nm excitation is ∼10 times higher than core nanoparticles. This is due to the removal of dangling bonds on the surface of the active core by the inactive shell. Moreover, these nanoparticles are highly dispersible in water. This may be due to the interaction of EG present on the surface of nanoparticles with H2O.
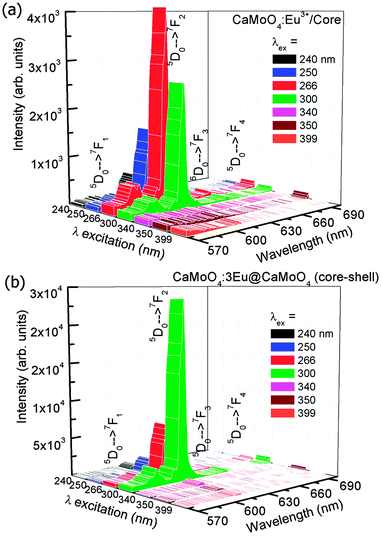 |
| Fig. 8 Emission spectra of Eu3+ doped CaMoO4 (Eu3+ = 1, 3, 5, 7, 10, 15, 20 and 30 at%) of the as-prepared (a) core and (b) core@shell nanoparticles under different excitation wavelengths. Excitation source is from a xenon lamp (450 W). | |
3.3.2 Effect of host sensitizer.
CaMoO4 nanoparticles show a broad absorption band in ∼200–350 nm. This is related to Mo–O CTB of host (CaMoO4). Upon excitation at 260–300 nm, it shows the emission spectrum in 320–650 nm.7,39 Also, absorption band of Eu–O CTB occurs in 230–260 nm in most hosts doped with Eu3+.45,46 Thus excitation at 266 nm is related to Mo–O/Eu–O CTB. This broad-band light emitted from host (CaMoO4 or Eu–O CTB) in 320–650 nm is absorbed by Eu3+ ions in the ground state (7F0 → 5L6 at 395 nm and 7F0 → 5D2 at 465 nm) and thereby enhancement of Eu3+ emission occurs.47,48 The energy transfer from the host (as sensitizer) to an activator will depend on the overlapping of an energy band of the host (CaMoO4) emitting light with the activator (Eu3+) absorption. This is schematically shown in Fig. 9.
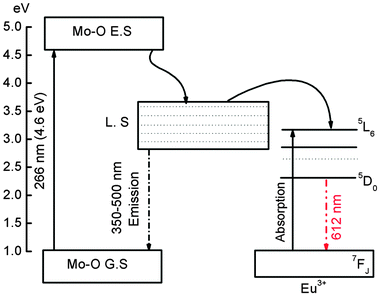 |
| Fig. 9 Schematic energy diagram of absorption, emission bands of Mo–O CT and Eu3+ and the energy transfer (ET) process. | |
3.3.3 Induction heating of hybrid MN.
The heat-generation from hybrid Fe3O4–CaMoO4:Eu MN is studied using an induction heating setup on applying different input currents 200, 300 and 400 A. 1 mL of dispersed hybrid MN in water is placed in an induction heating setup coupled with temperature sensing optical fibre thermocouple. Fig. 10(a) shows the digital photograph of induction heating coil used in the experiment, and Fig. 10(b) shows their temperature kinetics curves of the sample (32 mg/1 mL). Here PEG solution (100 mg of polyethylene 6000 in 100 mL distilled water) is used as the medium. It is shown that temperature rises with an increase in time and current. Hybrid MN achieves ∼42 °C temperature in 9 min for 300 A and 4.3 min for 400 A applied current, whereas temperature attained is below 36 °C for 200 A current in 10 min. The PEG coated over the surface of hybrid nanoparticles makes the nanoparticles more dispersible. In general pure Fe3O4 MN (10 mg mL−1, medium is sodium carbonate) shows high induction heating ability (∼60 °C in 10 min at 265 kHz frequency and 400 A (i.e., 335 Oe)), reported in our earlier study.49 The rise in temperature is also controlled by the amount of Fe3O4 nanoparticles as well as the applied field at a particular frequency. The temperature required for hyperthermia application for killing the cancer tissues is ∼42 °C.49 The specific absorption rate (SAR) of hybrid MN is calculated by using following relationship: | SAR = C(ΔT/Δt)(1/mmag) | (6) |
where C is the sample-specific heat capacity and ΔT/Δt is the initial slope of the time-dependent temperature curve. The value of mmag is considered as the amount of magnetite or Fe per total amount of magnetite or Fe and water. The value of ΔT/Δt is calculated in the range 0–5 min. Here the volume of water was 1 mL, which is equal to 1 g. 1 mL of Fe3O4–CaMoO4:Eu contains 7.2 mg (Fe3O3) and 25 mg of CaMoO4:Eu. The SAR values are found to be 10, 19 and 26 W g−1 for 200, 300 and 400 A applied current, respectively. These SAR values are comparable with reported values.49 Fe3O3–CaMoO4:Eu hybrid nanoparticles are effectively used for hyperthermia applications. Fig. 11 gives the magnetization vs. magnetic field (H) of hybrid (Fe3O4–CaMoO4) at 300 K. There is no coercivity indicating superparamagnetism. Magnetization at 2 Tesla is ∼14.2 emu g−1. This value includes CaMoO4 also. Ratio of CaMoO4 to Fe3O4 is 4
:
1. Thus magnetization per 1 g of Fe3O4 is ∼63 emu. Magnetization of pure Fe3O4 is ∼60 emu g−1.22 Enhancement in magnetization is related to an increase in crystalline anisotropic (K) in the hybrid due to the addition of 4f orbitals of Eu3+ in the hybrid.
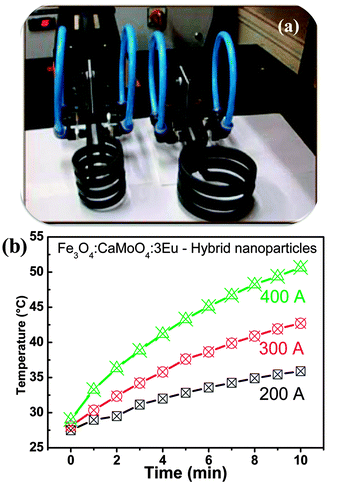 |
| Fig. 10 (a) Digital photograph of induction heating system and (b) temperature attained vs. time at different alternative currents (200, 300 and 400 A) for Fe3O4–CaMoO4:3Eu hybrid magnetic nanoparticles in the induction coil. | |
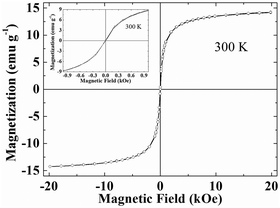 |
| Fig. 11 Magnetization (M) versus applied magnetic field (H) curve for Fe3O4–CaMoO4:3Eu hybrid magnetic nanoparticles. | |
3.4 Luminescence of hybrid nanoparticles
Fig. 12 shows the emission spectrum of Fe3O3–CaMoO4:Eu (3 at%) hybrid nanoparticles under 250 nm excitation (xenon lamp 450 W) and 398 nm excitation (not shown). It shows strong red emission at ∼612 nm which is assigned to the 5D0 → 7F2 transition of the Eu3+ ion. Moreover, the emission intensity of hybrid nanoparticles is slightly less than the pure CaMoO4:Eu sample. This may be due to the presence of less number of Eu3+ ions per unit mass of sample and also magnetic nanoparticles quench luminescence intensity.
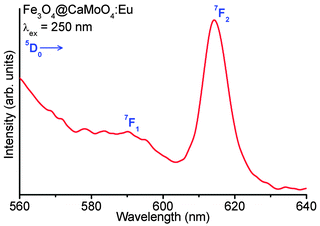 |
| Fig. 12 Luminescence spectrum of Fe3O4@CaMoO4:3Eu hybrid magnetic nanoparticles under 250 nm excitation. | |
3.5
In vitro cytotoxicity (MTT assay) of Fe3O4–CaMoO4:Eu hybrid nanoparticles
To provide comprehensive results about the potential applications of synthesized Fe3O4–CaMoO4:Eu (3 at%) hybrid magnetic nanoparticles for non-invasive imaging purposes, it is essential to measure the cytotoxicity of the synthesized nanoparticles. The cellular cytotoxicity profiles of the Fe3O4–CaMoO4:Eu hybrid magnetic nanoparticles are determined on two type cell lines, human hepatoblastoma (HepG2) and hTERT cells, using a MTT assay. As shown in Fig. 13 and 14, the cells are treated with different concentrations of Fe3O4–CaMoO4:Eu hybrid magnetic nanoparticles for 24 h, to determine the effect of concentration of the nanoparticles. The viability of non-treated cells is assumed to be 100%. On incubation of the HepG2 cells with the Fe3O4–CaMoO4:Eu hybrid magnetic nanoparticles for 24 h at a concentration of 5 μg mL−1 it shows little change in cell viability. Whereas the cells exposed to increasing concentrations (5, 10, 20, 40, 80, 160, 320 and 640 μg mL−1) of Fe3O4–CaMoO4:Eu hybrid magnetic nanoparticles for 24 h show a noticeable dose-dependent decrease in their relative cell viability. The IC50 value (Fe3O4–CaMoO4:Eu inhibits the 50% cell viability) of the Fe3O4–CaMoO4:Eu hybrid magnetic nanoparticles is calculated to be approximately 193.26 μg mL−1.
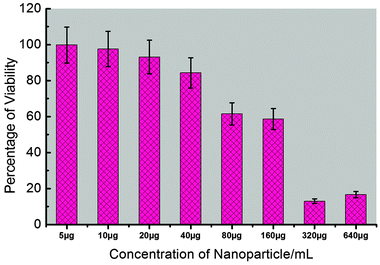 |
| Fig. 13 Cytotoxicity of Fe3O4–CaMoO4:Eu hybrid magnetic nanoparticles against HepG2 cells determined by MTT assay after 24 h of treatment. IC50 was determined as 193.26 μg mL−1. Error bars represent standard deviation (n = 3). | |
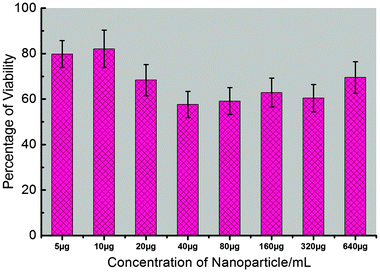 |
| Fig. 14 Cytotoxicity of Fe3O4–CaMoO4:Eu hybrid magnetic nanoparticles against hTERT 20 cells determined by MTT assay after 24 h of treatment. IC50 was not achieved by the tested doses range (5–640 μg mL−1). Error bars represent standard deviation (n = 3). | |
The toxic effect of Fe3O4–CaMoO4:Eu hybrid magnetic nanoparticles on cell viability was also assessed on a second cell line, hTERT cells (human mesenchymal stem cells). This assay is used to assess the viability of the hTERT cell line after exposure of Fe3O4–CaMoO4:Eu hybrid magnetic nanoparticles at various concentrations for 24 h. As is evident from Fig. 14, in general, the Fe3O4–CaMoO4:Eu hybrid magnetic nanoparticles show no significant dose-dependent cytotoxic effects even at higher concentrations. About 60–80% cells are viable when compared to the control. These results confirmed the non-toxic nature of Fe3O4–CaMoO4:Eu hybrid magnetic nanoparticles in the in vitro model. In other words, these composite nanoparticles had better biocompatibility in in vitro cell assays. The results of this study are in agreement with the current fact that the most often used biocompatible material for the preparation of magnetic particles is the iron oxide magnetite. Interestingly, Fe3O4–CaMoO4:Eu exert a mild toxicity (75% viability at the highest concentration) on a normal cell line hTERT, whereas HepG2 cells (a cancerous cell line) show their sensitivity against the same hybrid-nanocomposite. Therefore, it is suggested that the acute cytotoxicity primarily is originated from the cellular internalization of Fe3O4–CaMoO4:Eu hybrid magnetic nanoparticles rather than physical damage on the cellular membrane. Moreover, it has been proven by previous studies that nanoparticles can cross the cell membrane and enter the cytoplasm through several different routes.50,51 A recent study demonstrated that the cellular response of human foreskin fibroblasts is different for the different morphologies of composite nanoparticles, with spherical particles exhibiting no cytotoxicity, rod-like particles increasing cell proliferation, and platelet particles being markedly cytotoxic.50 Once a metal present in the particle has penetrated the cells, metal ions can leach from the particle and generate reactive oxygen species (ROS) in the cell interior leading to oxidative stress to cells, in what is called a ‘‘Trojan horse’’ mechanism.52,53
The toxicological effects of Fe3O4–CaMoO4:Eu hybrid magnetic nanoparticles are also monitored by analyzing the morphology of cells. The Fe3O4–CaMoO4:Eu hybrid magnetic nanoparticles are treated with HepG2 and hTERT cell lines. An inverted optical microscope is used to examine the alteration in cell morphology between treated and non-treated hybrid magnetic nanoparticles (Fig. 15). The cells are grown with normal colonies-like morphologies after co-labelling with the Fe3O4–CaMoO4:Eu hybrid magnetic nanoparticles and DAPI for cell nuclei counterstaining. The fluorescence images demonstrate that the Fe3O4–CaMoO4:Eu hybrid magnetic nanoparticles are clearly adsorbed onto the membrane and internalized into the cytoplasm of the cells, particularly with relatively light blue (Fig. 15) luminescence. It is found that the control cells are healthy and start to proliferate and gradually grew to form small colonies (Fig. 15(a and c)), however, the treated hTERT cells are rounded off and formed clusters (Fig. 15(d)). It shows no significant cell death and no change in cell morphology as observed on microscopic examinations of cells during the period of study. These results indicate that the viability of the hTERT cells is not affected by the presence of the above samples, suggesting that they are quite biocompatible. In addition, there is no nuclear fragmentation (HepG2 cells formed colonies) when cells are treated with Fe3O4–CaMoO4:Eu hybrid magnetic nanoparticles (Fig. 15(b)). It is seen that the control cells do not show any fluorescence whereas the cells treated with Fe3O4–CaMoO4:Eu hybrid magnetic nanoparticles show strong luminescence, which shows its potential to label hTERT cells for bioimaging. The present study is to give an overall toxic response idea of the Fe3O4–CaMoO4:Eu hybrid magnetic nanoparticles. Interestingly, Fe3O4–CaMoO4:Eu hybrid magnetic nanoparticles are found potentially toxic for a cancer cell line (HepG2) and to a large extend non-toxic for a normal cell line (hTERT). It advocates the potentiality of the nanoparticles to be used as an anticancer drug vehicle. However, the confocal fluorescence microscopy imaging, genotoxic response experiments and their detailed toxic potentiality with other cancer and normal cell lines are currently underlying. These will be the subject of future investigations.
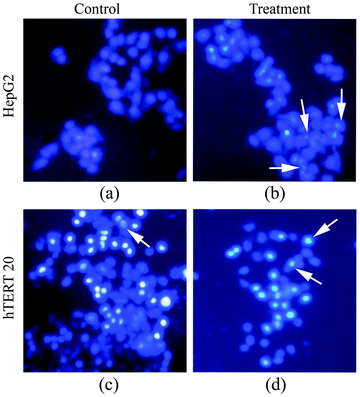 |
| Fig. 15 Microscopic visualizations of HepG2 and hTERT 20 cells after staining with fluorescent (DAPI) dye, (a & c) are untreated controlled cells of HepG2 and hTERT 20 cells and (b & d) are treated with Fe3O4–CaMoO4:Eu hybrid magnetic nanoparticles. | |
4. Conclusions
CaMoO4:Eu3+ ions (Eu3+ = 1, 3, 5, 7, 10, 15, 20 and 30 at%) and 3 at% Eu3+ doped CaMoO4 core@shell nanoparticles have been prepared using urea hydrolysis in ethylene glycol medium at 150 °C. The emission intensity of Eu3+ doped CaMoO4 (core) nanoparticles is significantly enhanced by forming an inactive shell (CaMoO4) on the core. In the case of the as-prepared samples, the emission intensity of core@shell nanoparticles is ∼10 times higher than that of core nanoparticles on 300 nm excitation. The effect of pump power on the asymmetric ratio (A21) has been studied. It is found that A21 values under laser excitation are slightly higher than xenon lamp excitation as a source. Also, increase in pump power enhances the A21 up to optimum power and then decreases. The prepared nanoparticles show high dispersibility in water. The hybrid Fe3O4–CaMoO4:Eu MN can attain hyperthermia temperature (42 °C) and give luminescence in the red region, which lies in the biological window. The cell viability and proliferation test on hTERT cells indicated that Fe3O4–CaMoO4:Eu hybrid nanoparticles are less toxic or antiproliferative effects in the cell cultures, whereas the cell viability on HepG2 cells are reduced and showed the toxic effects. So they can be a potential candidate for optical imaging, biological, hyperthermia and optoelectronic applications.
Acknowledgements
This work has been sponsored by the University Grants Commission (UGC) under Dr D. S. Kothari Postdoctoral Fellowship Scheme (No. F.4-2/2006(BSR)/13-309/2008(BSR)) to Dr A. K. Parchur. AKP and RSN thank Dr S. M. Yusuf, Solid State Physics Division, BARC, Mumbai for providing Magnetic data.
References
-
R. S. Ningthoujam, in Enhancement of luminescence by rare earth ions doping in semiconductor host, ed. S. B. Rai and Y. Dwivedi, Nova Science Publishers Inc, USA, 2012, ch. 6, pp. 145–182 Search PubMed.
- P. Ghosh, J. Oliva, E. D. Rosa, K. K. Haldar, D. Solis and A. Patra, J. Phys. Chem. C, 2008, 112, 9650 CAS.
- A. Kar, A. Datta and A. Patra, J. Mater. Chem., 2010, 20, 916 RSC.
- A. K. Parchur and R. S. Ningthoujam, Dalton Trans., 2011, 40, 7590 RSC.
- C. N. R. Rao, H. S. S. R. Matte, R. Voggu and A. Govindaraj, Dalton Trans., 2012, 41, 5089 RSC.
- A. A. Ansari, M. Alam, J. P. Labis, S. A. Alrokayan, G. Shafi, T. N. Hasan, N. A. Syed and A. A. Alshatwi, J. Mater. Chem., 2011, 21, 19310 RSC.
- G. S. R. Raju, E. Pavitra, K. Y. Hwan and Y. J. Su, J. Mater. Chem., 2012, 22, 15562 RSC.
- A. A. Ansari, S. P. Singh, N. Singh and B. D. Malhotra, Spectrochim. Acta, Part A, 2012, 86, 432 CrossRef CAS PubMed.
- S. Vidya, S. Solomon and J. K. Thomas, Phys. Status Solidi A, 2012, 209, 1067 CrossRef CAS.
- J. H. Chung, J. H. Ryu, S. W. Mhin, K. M. Kim and K. B. Shim, J. Mater. Chem., 2012, 22, 3997 RSC.
- L. Tong, J. Shi, D. Liu, Q. Li, X. Ren and H. Yang, J. Phys. Chem., 2012, 116, 7153 CAS.
- A. K. Parchur, A. I. Prasad, A. A. Ansari, S. B. Rai and R. S. Ningthoujam, Dalton Trans., 2012, 41, 11032 RSC.
- C. W. Raubach, M. Z. Krolow, M. F. Mesko, S. Cava and M. L. Moreira, CrystEngComm, 2012, 14, 393 RSC.
- F. Wang, R. Deng, J. Wang, Q. Wang, Y. Han, H. Zhu, X. Chen and X. Liu, Nat. Mater., 2011, 10, 1 CrossRef PubMed.
- A. K. Parchur and R. S. Ningthoujam, RSC Adv., 2012, 2, 10854 RSC.
- X. Ju, X. Li, Y. Yang, W. Li, C. Tao and W. Feng, J. Solid State Chem., 2012, 187, 109 CrossRef CAS PubMed.
- I. L. V. Rosa, L. H. Oliveira, E. Longo and J. A. Varela, J. Fluoresc., 2011, 21, 975 CrossRef CAS PubMed.
- Z.-L. Wang, R. Guo, G.-R. Li, L.-X. Ding, Y.-N. Ou and Y.-X. Tong, RSC Adv., 2011, 1, 48 RSC.
- S. Zhou, Q. Chen, X. Hu and T. Zhao, J. Mater. Chem., 2012, 22, 8263 RSC.
- X. L. Liu, H. M. Fan, J. B. Yi, Y. Yang, E. S. G. Choo, J. M. Xue, D. D. Fan and J. Ding, J. Mater. Chem., 2012, 22, 8235 RSC.
- L. Zhou, J. Yuan and Y. Wei, J. Mater. Chem., 2011, 21, 2823 RSC.
- R. Ghosh, L. Pradhan, Y. P. Devi, S. S. Meena, R. Tewari, A. Kumar, S. Sharma, N. S. Gajbhiye, R. K. Vatsa, B. N. Pandey and R. S. Ningthoujam, J. Mater. Chem., 2011, 21, 13388 RSC.
- M. Jeun, S. Lee, J. K. Kang, A. Tomitaka, K. W. Kang, Y. I. Kim, Y. Takemura, K.-W. Chung, J. Kwak and S. Bae, Appl. Phys. Lett., 2012, 100, 092406 CrossRef.
- T. T. T. Mai, P. T. Ha, H. N. Pham, T. T. H. Le, H. L. Pham, T. B. H. Phan, D. L. Tran and X. P. Nguyen, Adv. Nat. Sci.: Nanosci. Nanotechnol., 2012, 3, 015006 CrossRef.
- L. Tong, D. Liu, J. Shi, X. Yang and H. Yang, J. Mater. Sci., 2012, 47, 132 CrossRef CAS.
- H. Peng, G. Liu, X. Dong, J. Wang, J. Xu and W. Yu, J. Alloys Compd., 2011, 509, 6930 CrossRef CAS PubMed.
- W. Wang, M. Zou and K. Chen, Chem. Commun., 2010, 46, 5100 RSC.
- K. Ariga, T. Mori and J. P. Hill, Adv. Mater., 2012, 24, 158 CrossRef CAS PubMed.
- K. Ariga, A. Vinu, Y. Yamauchi, Q. Ji and J. P. Hill, Bull. Chem. Soc. Jpn., 2012, 85, 1 CrossRef CAS.
- K. K. Haldar, T. Sen, S. Mandal and A. Patra, ChemPhysChem, 2012, 13, 3989 CrossRef CAS PubMed.
- P. Drake, H.-J. Cho, P.-S. Shih, C.-H. Kao, K.-F. Lee, C.-H. Kuo, X.-Z. Lin and Y.-J. Lin, J. Mater. Chem., 2007, 17, 4914 RSC.
- T. Mossman, J. Immunol. Methods, 1983, 65, 55 CrossRef.
- S. Ishihara, N. Iyi, Y. Tsujimoto, S. Tominaka, Y. Matsushita, V. Krishnan, M. Akada, J. Labuta, K. Deguchi, S. Ohki, M. Tansho, T. Shimizu, Q. Ji, Y. Yamauchi, J. Hill, H. Abe and K. Ariga, Chem. Commun., 2013, 49, 3631 RSC.
- A. Kar, S. Kundu and A. Patra, J. Phys. Chem. C, 2011, 115, 118 CAS.
- S. Dutta, S. Som and S. Sharma, Dalton Trans., 2013, 42, 9654 RSC.
- V. S. Marques, L. S. Cavalcante, J. C. Sczancoski, A. F. P. Alcântara, M. O. Orlandi, E. Moraes, E. Longo, J. A. Varela, M. S. Li and M. R. M. C. Santos, Cryst. Growth Des., 2010, 10, 4752 CAS.
- L. S. Cavalcante, V. M. Longo, J. C. Sczancoski, M. A. P. Almeida, A. A. Batista, J. A. Varela, M. O. Orlandi, E. Longo and M. S. Li, CrystEngComm, 2012, 14, 853 RSC.
- E. Sarantopoulou, C. Raptis, S. Ves, D. Christofilos and G. A. Kourouklis, J. Phys.: Condens. Matter, 2002, 14, 8925 CrossRef CAS.
- A. K. Parchur, R. S. Ningthoujam, S. B. Rai, G. S. Okram, R. A. Singh, M. Tyagi, S. C. Gadkari, R. Tewari and R. K. Vatsa, Dalton Trans., 2011, 40, 7595 RSC.
- N. K. Sahu, R. S. Ningthoujam and D. Bahadur, J. Appl. Phys., 2012, 112, 014306 CrossRef.
- A. K. Parchur and R. S. Ningthoujam, RSC Adv., 2012, 2, 10859 RSC.
- A. Xie, X. Yuan, S. Hail, J. Wang, F. Wang and L. Li, J. Phys. D: Appl. Phys., 2009, 42, 105107 CrossRef.
- H. Wu, Y. Hu, W. Zhang, F. Kang, N. Li and G. Ju, J. Sol-Gel Sci. Technol., 2012, 62, 227 CrossRef CAS PubMed.
- R. S. Ningthoujam, L. Robindro Singh, V. Sudarsan and S. D. Singh, J. Alloys Compd., 2009, 484, 782 CrossRef CAS PubMed.
- S. Gago, M. Pillinger, R. A. Ferreira, L. D. Carlos, T. M. Santos and I. S. Gonçalves, Chem. Mater., 2005, 17, 5803 CrossRef CAS.
- A. I. Prasad, A. K. Parchur, R. R. Juluri, R. S. Ningthoujam and R. K. Vatsa, Dalton Trans., 2013, 42, 4885 RSC.
- T. Förster, Ann. Phys., 1948, 437, 55 CrossRef.
- D. L. Dexter, J. Chem. Phys., 1953, 21, 836 CrossRef CAS.
- N. V. Jadhav, A. I. Prasad, A. Kumar, R. Mishra, S. Dhara, K. R. Babu, C. L. Prajapat, N. L. Misra, R. S. Ningthoujam, B. N. Pandey and R. K. Vatsa, Colloids Surf., B, 2013, 108, 158 CrossRef CAS PubMed.
- M. Geiser, B. Rothen-Rutishauser, N. Kapp, S. Schurch, W. Kreyling, H. Schulz, M. Semmler, V. I. Hof, J. Heyder and P. Gehr, Environ. Health Perspect., 2005, 113, 1555 CrossRef.
- H. Yang, C. Liu, D. Yang, H. Zhang and Z. Xi, J. Appl. Toxicol., 2009, 29, 69 CrossRef CAS PubMed.
- T. Andelman, S. Gordonov, G. Busto, P. V. Moghe and R. E. Riman, Nanoscale Res. Lett., 2009, 5, 263 CrossRef PubMed.
- E. Engelhaupt, L. Thrall, B. Booth and R. Chaterjee, Environ. Sci. Technol., 2007, 41, 3788 CrossRef CAS.
Footnote |
† Electronic supplementary information (ESI) available. See DOI: 10.1039/c3ib40148k |
|
This journal is © The Royal Society of Chemistry 2014 |
Click here to see how this site uses Cookies. View our privacy policy here.