DOI:
10.1039/C3BM60238A
(Paper)
Biomater. Sci., 2014,
2, 512-521
Covalent attachment of multilayers (CAM): a platform for pH switchable antimicrobial and anticoagulant polymeric surfaces†
Received
2nd October 2013
, Accepted 13th December 2013
First published on 6th January 2014
Abstract
These studies show covalent attachment of multilayers (CAM) to chemically alter surfaces to achieve pH switchable antimicrobial and anticoagulant properties. Polyethylene (PE), poly(tetrafluoroethylene) (PTFE), and silicon (Si) surfaces were functionalized by tethering pH-responsive “switching” polyelectrolytes consisting of poly(2-vinyl pyridine) (P2VP) and poly(acrylic acid) (PAA) terminated with NH2 and COOH groups, respectively. At pH < 2.3, the P2VP segments are protonated and expended, but at pH > 5.5, they collapse while the PAA segments are expanded. The presence of terminal NH2 or COOH moieties on P2VP and PAA, respectively, facilitated the opportunity for covalently bonding ampicillin (AMP) and heparin (HEP) to both polyelectrolyte chains. Such surfaces, when exposed to S. aureus, inhibit the growth of microbial films (AMP) as well as anticoagulant properties (HEP). Comparison of “dynamic” pH dependent surfaces developed in these studies with “static” surfaces terminated with (AMP) entities shows significant enhancement in the longevity of surface activity against microbial film formation.
Introduction
Creating surfaces on polymeric materials provides an opportunity for altering their interactions with the environment, while maintaining useful bulk properties. This is particularly critical in biomaterials which, in order to coexist with biological systems, must maintain bulk characteristics, yet exhibit desirable surface and interfacial properties not interfering with multifaceted bioactive functions. Bio-sensing devices, drug delivery systems, artificial tissues, or implants are just a few among many examples, where functionalizing surfaces in contact with a biological system is critical. On the flip side, the remarkable ability of biological systems to recognize the physical shape, surface morphology as well as chemical surface groups may often lead to undesirable adsorption processes on polymeric surfaces. Perhaps the most prominent and undesirable examples of bio-adsorption are microbial film formation and blood coagulation. In this context, surface plasma modifications of polymers have been known for a long time and commercial products (catheters, stents, etc.) are readily available; these surface modifications are typically conducted to alter adhesion or for sterilization purposes. Although numerous studies have been conducted on polymer surface modifications and summarized in various monographs,1–3 recent advances in surface and interfacial reactions4 have resulted in the development of a new class of materials that exhibit stimuli-responsive characteristics. For example, by creating switchable surfaces through the use of polyelectrolytes,5 followed by functionalizing their end groups, a given surface may adapt itself to environmental pH changes.
The majority of model surface reactions offering stimuli-responsive characteristics have focused on silicon and gold surfaces,6 but covalent attachment to polymeric substrates is challenging due to the inert nature of polymeric surfaces as well as their morphological heterogeneities. This is particularly important if responses to an external stimulus, such as temperature,7–10 ionic strength,11 UV radiation,10 or pH,5,7,8 are required. In contrast to numerous studies involving layer-by-layer deposition processes, which not only offer mechanically fragile and biochemically unstable surfaces, these studies offer the synthesis of covalently attached multilayers (CAM)12 stimuli-responsive polymeric surfaces “decorated” by cationic and anionic polyelectrolytes terminated with –COOH or –NH2 groups. Combining the CAM approach with the earlier studies,5 the advantage of oppositely charged polyelectrolytes not only facilitates the formation of hydrophilic surfaces on hydrophobic polymer substrates, but also introduces surface dynamics driven by external pH changes. Specifically, at higher pH values, cationic segments will extend, but anionic tethers will collapse, and vice versa. Fig. 1A illustrates an overall scheme of the coupling reactions facilitating the attachment of poly(2-vinyl pyridine) (P2VP) and poly(acrylic acid) (PAA). The choice of P2VP and PAA chain ends terminated with –NH2 and –COOH functionalities, respectively, also facilitating other reactions to such surfaces. Since many polymeric substrates are utilized in biomedical applications, we chose the attachment of anticoagulant heparin (HEP) (on P2VP) and antimicrobial ampicillin (AMP) (on PAA) (Fig. 1B) in an effort to obtain dynamic antimicrobial and anticoagulant pH responsive polymeric surfaces.
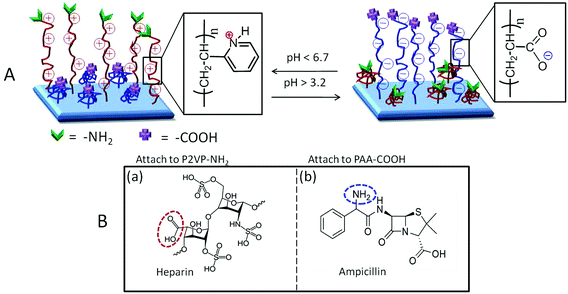 |
| Fig. 1 (A) Schematic diagram of dual stimuli-responsive polyelectrolyte surfaces terminated with –NH2 and –COOH moieties; (B) structures of (a) HEP and (b) AMP, circled moieties are available for attachment. | |
Experimental section
Covalent attachment of multilayers (CAM)
All reagents used were purchased from Sigma-Aldrich and used as received unless otherwise specified. Medical grade poly(tetrafluoroethylene) (PTFE) and ultra-high molecular weight polyethylene (PE) were purchased from McMaster-Carr Supply Co. (Atlanta, GA), cut into 1 × 1 cm squares, washed in isopropanol, and dried at room temperature in a desiccator before use. Silicon (Si) wafers were purchased from Ted Pella Inc. and washed in isopropanol before use. To obtain –COOH terminated PTFE surfaces, microwave plasma reactions were conducted in the presence of maleic anhydride (MA) under open reactor conditions, as described elsewhere.13,14 In the next step, –COOH exhibiting surfaces were placed in oxalyl chloride solution for 2 h in order to create –COCl groups followed by immediate immersion into undiluted ethylenediamine (EDN) for 4 h. α-Amino-ω-carboxy-terminated heterobifunctional poly-2-vinylpyridine (P2VP; Mn = 10
000; Polymer Source Inc.) and α-ω-dicarboxy-terminated bifunctional poly-t-butylacrylate (PtBA; Mn = 10
000; Polymer Source Inc.) were used as received. Initially, the –COOH terminal ends of 0.5 μM PtBA were activated in 10 mL oxalyl chloride for 2 h. The –NH2 functionalized PE and PTFE surfaces were placed in the activated PtBA solution for 16 h. Upon removal, the specimens were washed in chloroform and then washed in deionized (DI) water for 30 min, and dried in a desiccator. The terminal –COOH groups of 0.5 μM –COOH–NH2 terminated P2VP were converted to –COCl groups by initially dissolving in 10 mL chloroform and then adding 10 mL oxalyl chloride to the solution for 2 h before PtBA modified polymer surfaces were added to the solution and stirred for 16 h.
Surface modifications were carried out to attach ampicillin (AMP) to PtBA tethered chains and heparin (HEP) to P2VP tethered chains. For AMP terminated surfaces, AMP was attached via carbodiimide coupling chemistry. PtBA and P2VP tethered surfaces with terminal –COOH were placed in 15 mL methylene chloride with 1.3 nM dicyclohexyl-carbodiimide (DCC) (Fluka) as a coupling agent and 0.25 mM 4-(dimethylamino)-pyridine (DMAP) as a catalyst. 0.1 g of AMP was dissolved in 5 mL methylene chloride which was then added to the DCC/DMAP flask containing the surfaces. The specimens were stirred in the mixture for 6 h in an ice water bath followed by washing in methylene chloride and then in DI water for 30 min and dried in a desiccator. HEP was attached via carbodiimide coupling chemistry by dissolving 1.3 mM 1-ethyl-3-(dimethylaminopropyl) carbodiimide (EDC) and 0.25 mM N-hydroxysuccinimide (NHS), as well as 0.5 mM HEP in a 10 mL acetate buffer (pH 4.6). P2VP and PtBA tethered surfaces were added to the mixture and stirred for 16 h, followed by washing in DI water for 30 min and drying. Once terminal chain ends of PtBA were functionalized, trifluoroacetic acid ≥99% in methanol was used to remove the t-butoxy group leading to the formation of PAA. In an effort to eliminate the possibility of physisorption of drug molecules, each specimen was placed in a boiling water bath for 20 min prior to spectroscopic analysis in order to remove physisorbed entities.
Dilute solutions of sodium hydroxide and hydrochloric acid in DI water at pH 2, 4.9, and 10 were prepared using a Thermo Orion pH meter model 350 with a glass combination electrode (Orion 9202 BN). The PTFE and PE surfaces presenting both PAA and P2VP were placed in the pH specific solutions for 1 h followed by drying at 50 °C to lock in the extended/collapsed configuration.
Surface characterization
Attenuated total reflectance Fourier transform infrared (ATR FT-IR) spectra were collected using a Bio-Rad FTS-6000 FT-IR single-beam spectrometer set at a 4 cm−1 resolution equipped with a DTGS detector and a 45° face angle Ge crystal. Each spectrum represents 200 co-added scans ratioed against a reference spectrum obtained by recording 200 co-added scans of an empty ATR cell. All spectra were corrected spectral distortions using Q-ATR software.15 Although in the past XPS has been often used in surface analysis of polymers, the use of ATR FT-IR provides unprecedented sensitivity for chemical analysis of surface functional groups.
Atomic force microscopy (AFM) measurements were conducted on a Nanoscope IIIa Dimension 3000 scanning probe microscope, Digital Instruments. A silicon probe with a 125 μm long silicon cantilever, a nominal force constant of 40 N m−1 and a resonance frequency of 275 kHz was used in tapping mode, allowing the assessment of surface topography. AFM data were analyzed using WSxM software16 to obtain RMS values, 3-D AFM images, and surface profile measurements.
Antimicrobial testing of ampicillin tethered surfaces
To determine the anti-microbial activity of AMP functionalized polyelectrolyte tethered surfaces, each specimen was exposed to cultures of Staphylococcus aureus (S. aureus) (RN 4420). S. aureus bacteria were incubated for 4 hours at 37 °C in Triptic Soy Broth (TSB). Bacterial growth was determined by optical density, measured from absorbance at 600 nm using a UV-vis spectrometer. 0.1 mL of S. aureus at a concentration of 10 μg mL−1 in TSB was placed on the sample surface and incubated for 4 h. After incubation, 10 μL of solution was removed from the surface and used to make serial dilutions. The dilutions were plated on Triptic Soy Agar (TSA) plates using a drop-plate method,17,18 and the bacterial colonies were counted.
Anticoagulant testing of heparin tethered surfaces
For the determination of anticoagulant activity, lagomorph blood was collected by venipuncture and transferred to a syringe containing 10 μL mL−1 heparin. The use of laboratory animals followed the NIH guidelines. Heparin attached samples and controls were placed into 2.5 mL vials without any additive and filled with heparinized lagomorph blood followed by incubation at 37 °C for 2 h on a hematology mixer (Fisher Scientific, Pittsburgh, PA). Samples were rinsed with DI water to remove any non-adhered blood from the surfaces. Raman spectra were obtained using a Renishaw Raman microscope-spectrometer equipped with a computer controlled three-axis encoded motorized stage, a RenCam CCD detector, and a Leica microscope (DMLM series). The 785 nm diode laser provided an excitation source with a maximum power output of 300 mW. The samples as well as the blood reference were placed on a gold slide, and each Raman spectrum was collected at 30 mW laser power at an acquisition time of 10 s. Raman imaging was carried out on each sample at 3 mW laser power for 1 min and tuned to 1620 cm−1 for detecting the presence of blood on each surface.
Results and discussion
While Fig. 1 depicted the general theme to achieve pH responsive anticoagulant and antimicrobial surfaces, Fig. 2 illustrates reaction sequences leading to the formation of covalently attached multilayers (CAM) tethered to Si, PE, and PTFE surfaces.
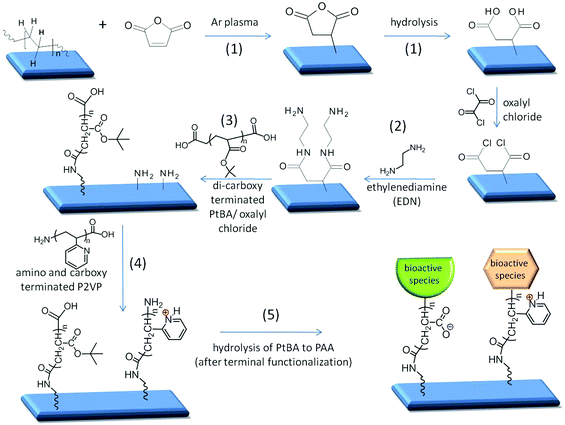 |
| Fig. 2 Reaction sequences on polymeric substrates leading to the formation of stimuli-responsive polyelectrolyte surfaces terminated with –COOH and –NH2 functionalities and ultimately exhibiting bioactive molecules. | |
In the first step (1), MA is reacted with a substrate,13 followed by hydrolysis to obtain –COOH groups, which are then converted to acid chloride. In the second step (2), EDN is reacted with –COCl groups resulting in –NH2 terminated surfaces. The next step (3) involves the attachment of dicarboxy terminated-PtBA to the –NH2 terminated surfaces via amide linkages, followed by the attachment of P2VP (4), carried out by reacting amide linkages between –COOH groups on P2VP and the –NH2 groups. Upon terminal functionalization with the desired bioactive species, PtBA is hydrolyzed under mild conditions to PAA polyelectrolyte (5). This choice of sequences with polyelectrolyte chain ends containing the –NH2 and –COOH terminal groups was deliberately utilized to achieve two objectives: the ability to attach bioactive species as well as to facilitate pH sensitive expansion/collapse responses. These studies are organized into the following sections: –COOH terminated CAM reactions; –NH2 terminated CAM reactions; switchable CAM on Si, PE, and PTFE surfaces; and bioactive CAM functionalized surfaces.
–COOH terminated CAM reactions
Following reaction sequences depicted in Fig. 2, Fig. 3a illustrates a sequence of CAM reactions leading to –COOH terminal entities. The first step involves MA reactions to a substrate, followed by EDN and PtBA (PAA) functionalization. In an effort to identify species responsible for the attachment of each subsequent layer, ATR-FTIR spectra were recorded after each step, and are illustrated in Fig. 3b–d for Si, PE, and PTFE, where Traces A, B, C, and D correspond to the reaction sequences shown in Fig. 3a.
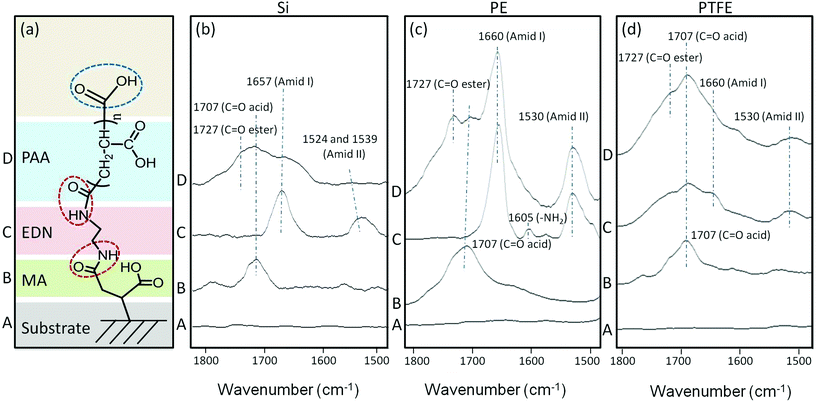 |
| Fig. 3 (a) Reaction sequences (circles represent reaction sites) on Si, PE, and PTFE surfaces leading to terminal –COOH groups; (b) ATR-FTIR spectra of A: Si, B: Si-MA, C: Si-MA-EDN, D: Si-MA-EDN-PtBA; (c) ATR-FTIR spectra of A: PE, B: PE-MA, C: PE-MA-EDN, D: PE-MA-EDN-PtBA; (d) ATR-FTIR spectra of A: PTFE, B: PTFE-MA, C: PTFE-MA-EDN, D: PTFE-MA-EDN-PtBA. | |
While Traces A of Fig. 3b–d represent the reference spectra of Si, PE, and PTFE substrates, Traces B of each figure show the band at 1707 cm−1 which corresponds to the C
O stretching vibrations of –COOH groups. Traces C and D in Fig. 3b–d illustrate the spectra recorded after amidation reactions on Si, PE, and PTFE, respectively, with the appearance of the bands at 1660 and 1530 cm−1 attributed to C
O vibrations of Amide I and II formation. PtBA is manifested by the band at 1727 cm−1 due to the C
O stretching vibrations of acrylate pendant groups along the polymer chain before their conversion to –COOH groups via hydrolysis reactions to obtain PAA. The advantage of having built-in pH sensitivity is the ability of reversible collapse as a function of pH in the range 3.2 < pH < 14, and the presence of terminal –COOH groups will facilitate a site for further reactions with bioactive species containing –NH2 moieties.
–NH2 terminated CAM reactions
The first step involves MA reactions to a substrate, followed by EDN and P2VP attachment, and Traces A, B, C, and D correspond to the CAM sequence shown in Fig. 4a. Reactions of P2VP to polymer surfaces facilitate pH responsiveness in the pH range of 1 < pH < 6.7 as well as the opportunity for terminating each tether with –NH2 functionalities for further reactions. Fig. 4a illustrates a sequence of CAM reactions leading to –NH2 terminal groups, whereas spectroscopic evidence for their formation on Si, PE, and PTFE is provided in Fig. 4b–d, respectively.
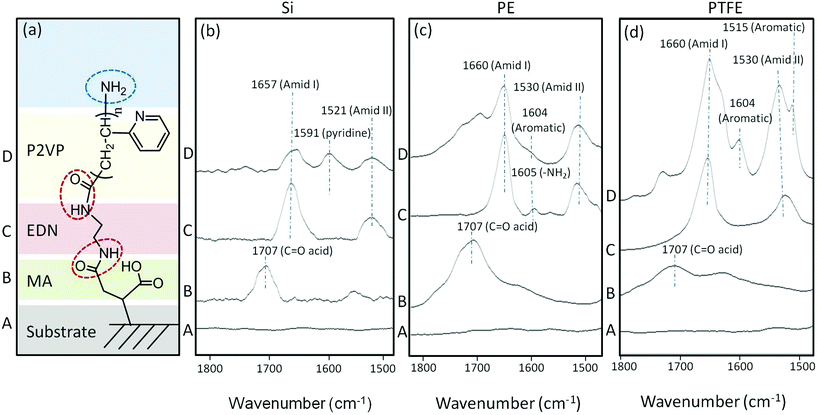 |
| Fig. 4 (a) Reaction sequences (circles represent reaction sites) on Si, PE, and PTFE surfaces leading to terminal –NH2 groups (circled); (b) ATR-FTIR spectra of A: Si, B: Si-MA, C: Si-MA-EDN, D: Si-MA-EDN-P2VP; (c) ATR-FTIR spectra of A: PE, B: PE-MA, C: PE-MA-EDN, D: PE-MA-EDN-P2VP; (d) ATR-FTIR spectra of A: PTFE, B: PTFE-MA, C: PTFE-MA-EDN, D: PTFE-MA-EDN-P2VP. | |
While Traces A in Fig. 4b–d represent Si, PE, and PTFE substrate spectra, respectively, the 1707 cm−1 band in Traces B of each figure corresponds to the C
O vibrations of –COOH groups due to maleic anhydride reactions and its subsequent hydrolysis. Traces C and D in Fig. 4b–d represent the spectra recorded after amidation reactions on Si, PE, and PTFE, respectively, where the C
O bands at 1660 and 1530 cm−1 manifest the Amide I and II formations, and covalent attachment of P2VP is represented by the band at 1620 cm−1 due to aromatic amine pendant groups along the polymer chain.
Switchable CAM on Si, PE, and PTFE surfaces
The simultaneous presence of PPA and P2VP provides an opportunity for creating switchable surfaces that respond to pH variations. While the formation of –COOH and –NH2 terminal groups facilitates attachment of other entities,‡ the switchability of the CAM reacted PAA and P2VP chains is attributed to the presence of side groups capable of protonation and deprotonation. Controlled by pH values, the response will deviate from the isoelectric point at pH 4.9,5 thus causing the PAA and P2VP surface chains to extend or collapse in response to solution ionic strength changes. For that reason we reacted PAA and P2VP polyelectrolytes with PTFE-MA-EDN surfaces and measured surface responsiveness as a function of pH. ATR-FTIR spectra of PAA and P2VP tethered surfaces recorded as a function of pH are shown in Fig. 5.
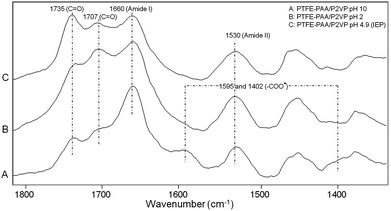 |
| Fig. 5 ATR-FTIR spectra of PTFE-MA-EDN-PAA/P2VP switching behavior observed at (A) pH 10, (B) pH 2, and (C) pH 4.9 (isoelectric point). | |
Trace A illustrates an ATR-FTIR spectrum of the PTFE-MA-EDN-PAA/P2VP surface at pH 10, where the presence of the bands at 1595 and 1402 cm−1 due to deprotonated –COO− H+ species is detected. When pH = 2, the disappearance of these bands is detected in Trace B. The band at 1707 cm−1 due to C
O stretching vibrations of –COOH groups also increases under acidic conditions, indicating that the PAA chains are protonated. Trace C illustrates the spectrum of the surface at pH 4.9 which was collected at the isoelectric point.2 However, the band at 1735 cm−1 due to C
O stretching vibrations of residual acrylate ester groups of unhydrolyzed PtBA is detected at all pH values, showing that the PtBA to PAA hydrolysis is incomplete. The bands at 1660 and 1530 cm−1 due to C
O stretching and N–H bending modes of Amide I and II groups, respectively, remain intact under all pH conditions, indicating their insensitive nature to pH variations.
In an effort to confirm the physical switchability of PAA and P2VP polyelectrolyte containing polymeric surfaces and correlate spectroscopic changes with surface morphologies that develop during pH exposure to CAM reacted surfaces, atomic force microscopy was employed. Fig. 6 illustrates 3-D AFM phase images for Si (A), PE (B), and PTFE (C) with a sequence of images depicting switchability of the tethered polyelectrolyte surfaces at a pH range from 2 to 10, respectively. While in Fig. 6, A1, B1, and C1 represent AFM images of Si, PE, and PTFE, respectively, a sequence of Fig. 6A′1, B′1 and C′1 represents the surface profiles obtained from AFM images. As we recall, the first reaction step involved MA attachment via microwave plasma reactions, and the AFM images of the reacted surfaces are depicted in Fig. 6A2, B2 and C2. Upon functionalization with P2VP and PAA polyelectrolyte chains, the same surfaces exposed to variable pH conditions change morphologies. However, as illustrated in the AFM images in Fig. 6A3, B3 and C3, upon exposure to pH 2, for Si-MA-P2VP, PE-MA-P2VP, and PTFE-MA-P2VP surfaces, P2VP segments are extended due to the protonation of the pyridine pendant groups on the polyelectrolyte chains. This behavior is reversed when the surfaces are exposed to pH 10, which causes the P2VP chains to collapse, as manifested by the AFM images in Fig. 6A4, B4 and C4. Images A5, B5, and C5 of Fig. 6 illustrate AFM of Si-MA-PAA, PE-MA-PAA, and PTFE-MA-PAA with collapsed PAA surface chains upon exposure to pH 2 solutions in which the –COOH groups of PAA are protonated. Fig. 6A6, B6 and C6 depict AFM images of the same surfaces exposed to solutions of pH 10 in which the PAA chains become extended due to the deprotonation of the –COOH-groups-to-form-carboxylate-ions. In an effort to illustrate the magnitude of surface morphology changes resulting from pH changes, Fig. 6A′, B′ and C′ show the surface profiles of the respective AFM images which correspond to the height of the surface-features. As seen, the magnitude of extend/collapse varies from a few to 40 nm which corresponds to the polyelectrolyte chains with Mn = 10
000.
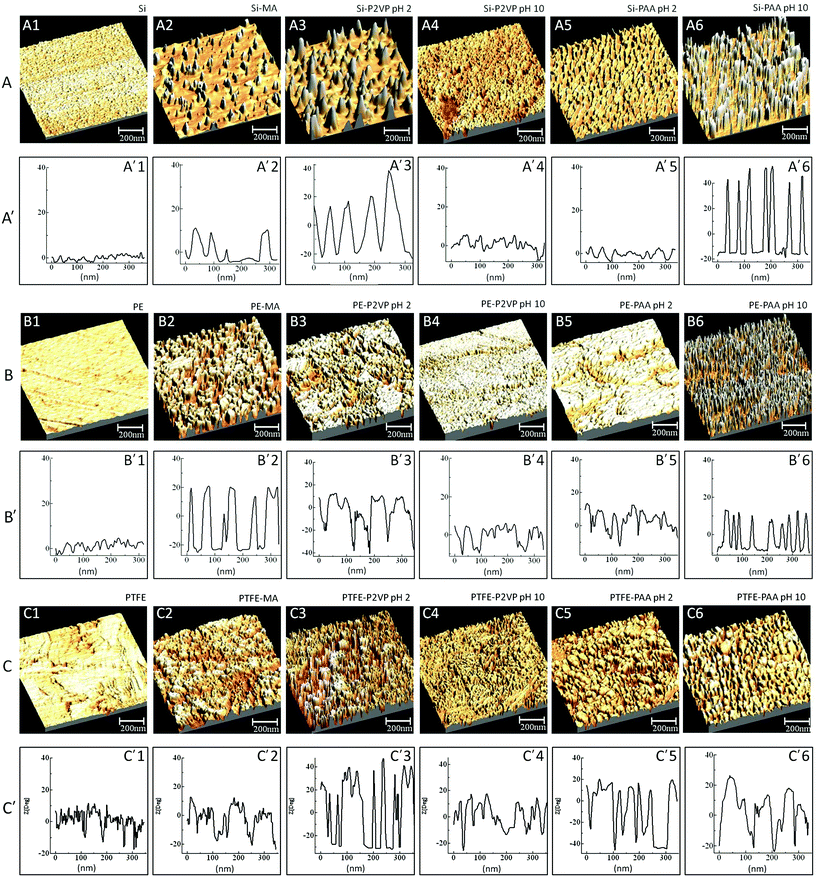 |
| Fig. 6 3D AFM phase images (A, B, and C) and corresponding surface profiles (A′, B′, and C′) of silica (A series), polyethylene (PE) (B series), and polytetrafluoroethylene (PTFE) (C series) surfaces: A series – Si substrate: A1 – Si surface, A2 – Si-MA, A3 – Si-MA-P2VP at pH 2, A4 – Si-MA-P2VP at pH 10, A5 – Si-MA-PAA at pH 2, A6 – Si-MA-PAA at pH 10; B series PE substrate: B1 – PE surface, B2 – PE-MA, B3 – PE-MA-P2VP at pH 2, B4 – PE-MA-P2VP at pH 10, B5 – PE-MA-PAA at pH 2, B6 – PE-MA-PAA at pH 10; C series PTFE substrate: C1 – PTFE, C2 – PTFE-MA, C3 – PTFE-MA-P2VP at pH 2, C4 – PTFE-MA-P2VP at pH 10, C5 – PTFE-MA-PAA at pH 2, and C6 – PTFE-MA-PAA at pH 10. Corresponding surface profiles A′, B′, and C′ represent surface roughness (nm) plotted as a function horizontal distance along the surface (nm). | |
Functionalization of CAM surfaces with bioactive species
As pointed out above, –COOH and –NH2 terminal groups on PE-MA-EDN-PAA, PTFE-MA-EDN-PAA, PE-MA-EDN-P2VP, and PTFE-MA-EDN-P2VP surfaces provide an opportunity for further reactions. Since antimicrobial and anticoagulant attributes are highly critical in many applications, we reacted AMP with PE-MA-EDN-PAA and PTFE-MA-EDN-PAA, as well as HEP with PE-MA-EDN-P2VP and PTFE-MA-EDN-P2VP surfaces. Fig. 7 illustrates the results of spectroscopic analysis of these reactions. Fig. 7a illustrates structural features resulting from the sequence of reactions, whereas Fig. 7b and c show ATR-FTIR spectra of surfaces after each step for PE and PTFE, respectively. All spectra in Fig. 7b and c contain amide linkages which are represented by the C
O band at 1660 and the N–H band at 1530 cm−1 indicating Amide I and II formations. As shown in Fig. 7b and c, amide linkages are manifested by the bands at 1660 and 1530 cm−1 due to C
O and N–H functionalities, indicating the formation of Amide I and II groups, respectively. At the same time, the β-lactam moiety of ampicillin is observed by the band at 1768 cm−1 due to C
O stretching vibrations. The HEP attachment shown in Fig. 7b′ and c′ is confirmed by the appearance of the bands at 1608 cm−1 due to –OH groups of HEP.
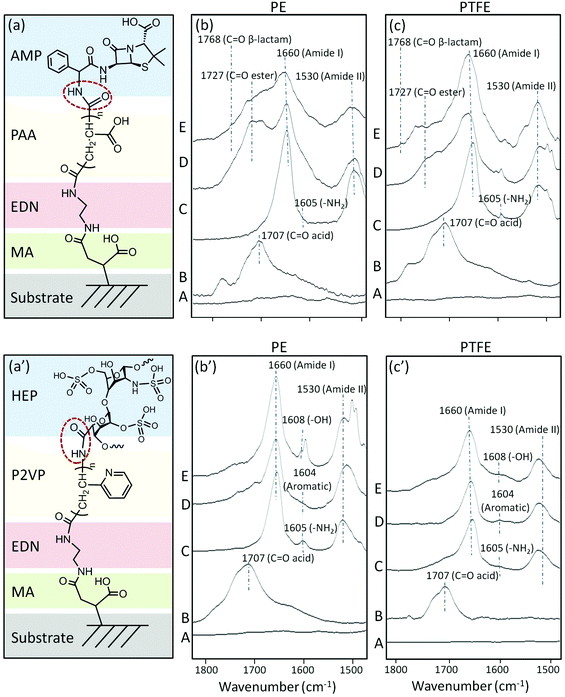 |
| Fig. 7 a and a′: Surface reactions on PE and PTFE surfaces leading to covalent bonding of AMP to PE-MA-EDN-PAA and PTFE-MA-EDN-PAA (a) and HEP to PE-MA-EDN-P2VP and PTFE-MA-EDN-P2VP (a′) surfaces (circles represent terminal NH2 and COOH reaction sites to which AMP and HP were reacted); b/b′ and c/c′ represent a series of ATR FT-IR spectra recorded from PE-MA-EDN-PAA-AMP (b), PTFE-MA-EDN-PAA-AMP (c), PE-MA-EDN-P2VP-HEP (b′), and PTFE-MA-EDN-P2VP-HEP (c′). Traces A–E represent ATR FT-IR spectra recorded from PE and PTFE substrates (Traces A), PE and PTFE surfaces with covalently attached MA (Traces B), PE and PTFE surfaces with covalently attached MA-EDN (Traces C), PE and PTFE surfaces with covalently attached MA-EDN-PAA (Traces D in b and c) and MA-EDN-P2VP (Traces D in b′ and c′), PE and PTFE surfaces with covalently MA-EDN-PAA-AMP (Traces E in b and c) and MA-EDN-PAA-HEP (Traces E in b′ and c′). | |
Bioactivity of functionalized CAM surfaces
PE-MA-EDN-PAA-AMP and PTFE-MA-EDN-PAA-AMP surfaces were tested for antimicrobial activity against Staphylococcus aureus and compared to non-pH sensitive surfaces. Fig. 8 illustrates the colony forming units (CFU) per cm2 enumerated using a drop plate method for PE/PTFE (a), PE-MA/PTFE-MA (b), PE-MA-EDN-PAA/PTFE-MA-EDN-PAA (c), and PE-MA-EDN-PAA-AMP/PTFE-MA-EDN-PAA-AMP (d) surfaces. Although the analysis of the data indicates that, in general, PE exhibits better antimicrobial resistance than PTFE, it is apparent that covalent attachment of AMP facilitates a major enhancement of antimicrobial activities. This is illustrated in Fig. 8d which shows a significant drop in CFUs from 4000–5000 to 10–40.
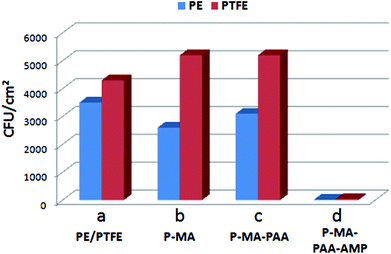 |
| Fig. 8 Antimicrobial activity (CFU cm−2) against S. aureus: (a) PE (blue) and PTFE (red) unmodified surfaces; (b) PE-MA and PTFE-MA surfaces; (c) PE-MA-EDN-PAA and PTFE-MA-EDN-PAA surfaces; (d) PE-MA-EDN-PAA-AMP and PTFE-MA-EDN-PAA-AMP surfaces. | |
In order to determine the longevity of surface antimicrobial activity of pH sensitive PE-MA-EDN-PAA-AMP and PTFE-MA-EDN-PAA-AMP surfaces, antimicrobial activity was tested against Staphylococcus aureus and compared to non-pH sensitive PE-MA-PEG-AMP and PTFE-MA-PEG-AMP surfaces. Fig. 9a, c and e illustrate the results of bacterial count represented by CFU cm−2 for pH-sensitive PE-MA-EDN-PAA-AMP and PTFE-MA-EDN-PAA-AMP surfaces. Each specimen was exposed to S. aureus for 4 hours at 37 °C (Fig. 9a), followed by washing, and two additional exposures to S. aureus for 4 hours (Fig. 9c and e), respectively. For comparison, Fig. 9b, d and f illustrate the results of bacterial count for non-pH sensitive PE-MA-PEG-AMP and PTFE-MA-PEG-AMP surfaces. Although initially both pH-sensitive and non-pH sensitive specimens are highly effective, as illustrated by the results shown in Fig. 9c-d and e-f, surfaces that are pH sensitive are more effective. As shown in Fig. 9, the observed antimicrobial activities in the presence of PAA spacers compared to PEG are enhanced. Since more stable amide linkages are formed upon attachment of ampicillin to PAA, these species will not readily hydrolyze. In contrast, the ability of PEG to hydrolyze results in even great differences in antimicrobial activities between the P-MA-PAA and P-MA-PEG spacers terminated with AMP upon multiple washes. It should be pointed out that the controls shown in Fig. 8 are in the range of 4000–5000 CFU cm−2, while the data in Fig. 9 are in the range of 0–400 CFU cm−2.
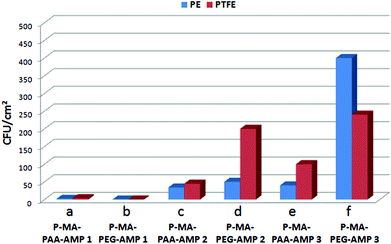 |
| Fig. 9 Antimicrobial activity (CFU cm−2) against S. aureus: PE-MA-EDN-PAA-AMP (blue) and PTFE-MA-EDN-PAA-AMP (red) surfaces (a, c, and e); PE-MA-PEG-AMP (red) and PTFE-MA-PEG-AMP (blue) surfaces (c, d, and f) after first (1), second (2), and third (3) 4 hour exposure to S. aureus. | |
Anticoagulant activity of PE-MA-P2VP and PTFE-MA-P2VP surfaces functionalized with HEP was determined using Raman imaging in the presence of whole lagomorph blood. Raman spectra (supporting documents) were collected before and after the exposure to whole blood and the band at 1620 cm−1 due to N–H stretching vibrations attributed to hemoglobin protein constituents19 was used to determine the degree of coagulation. In an effort to identify surface coagulation across the entire surface, Raman imaging from a 10 × 10 μm area was utilized. Raman images collected by tuning to the 1620 cm−1 band of the specimens obtained from incubating each surface in lagomorph blood are illustrated in Fig. 10, in which PE (a), PE-MA (b), PE-MA-EDN-P2VP (c), and PE-MA-EDN-P2VP-HEP (d) are Raman images of the substrates before blood exposure. As shown by color scale bars, red color represents the high intensity of the 1620 cm−1 band, which corresponds to 100% coagulation. As coagulation decreases, the color changes to blood which ideally represents 0% coagulation. Upon exposure, PE (a′) and PE-MA (b′) specimens do not exhibit anticoagulant activity, although PE-MA-EDN-P2VP (c′) shows some decrease and PE-MA-EDN-P2VP-HEP (d′) greatly minimizes clotting. For comparison, Fig. 10e shows the Raman image of dried lagomorph blood at 1620 cm−1. Similarly, Fig. 11 illustrates Raman images of PTFE (a), PTFE-MA (b), PTFE-MA-EDN-P2VP (c), and PTFE-MA-EDN-P2VP-HEP (d) substrates before blood exposure. As shown, PTFE (a′), PTFE-MA (b′), and PTFE-MA-EDN-P2VP (c′) do not exhibit anticoagulant activity, whereas PTFE-MA-EDN-P2VP-HEP (d′) minimizes clotting. For comparison, Fig. 11e shows the Raman image of dried lagomorph blood at 1620 cm−1. The effectiveness of HEP covalently attached to PE-MA-EDN-P2VP and PTFE-MA-EDN-P2VP surfaces against blood coagulation is apparent.
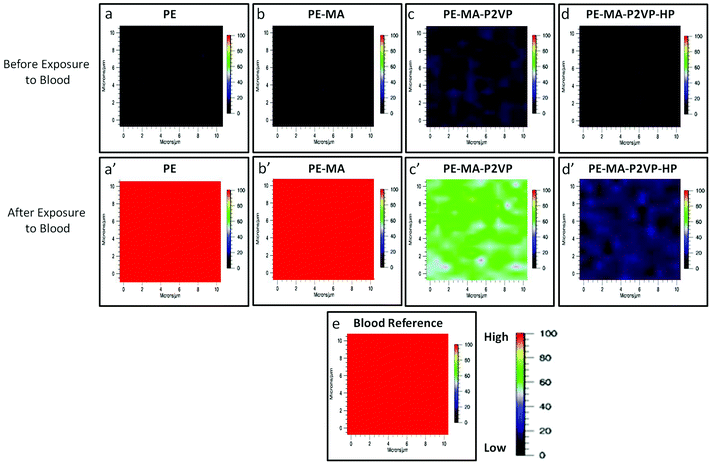 |
| Fig. 10 Raman images of the 1620 cm−1 band before/after exposure to whole blood to (a/a′) PTFE; (b/b′) PTFE-MA; (c/c′) PTFE-P2VP; (d/d′) PTFE-P2VP-HEP; (e) blood reference. Each image represents a 10 × 10 μm area. | |
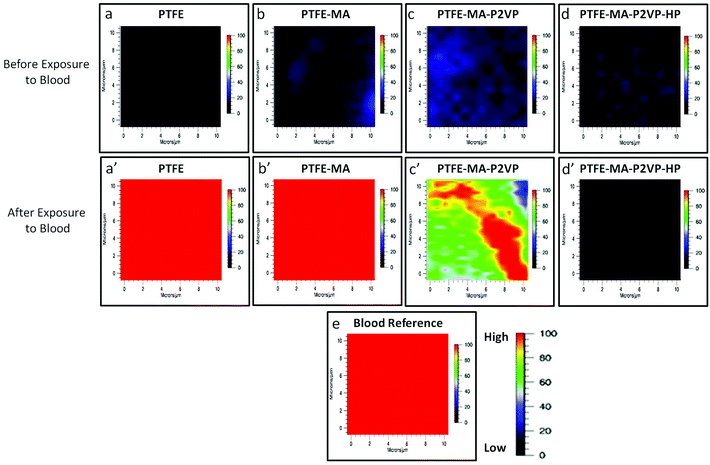 |
| Fig. 11 Raman images of the 1620 cm−1 band before/after exposure to whole blood to (a/a′) PE; (b/b′) PE-MA; (c/c′) PE-P2VP; (d/d′) PE-P2VP-HEP; (e) blood reference. Each image represents a 10 × 10 μm area. | |
Conclusions
In these studies we developed stimuli-responsive PE, PTFE, and Si surfaces using the covalent attachment of multilayers (CAM) approach that facilitated tethering of pH-responsive “switching” polyelectrolytes consisting of poly(2-vinyl pyridine) (P2VP) and poly(acrylic acid) (PAA) terminated with NH2 and COOH groups. Upon pH changes, these surfaces are able to collapse or extend. When pH < 2.3, P2VP segments are protonated and extended, while PAA segments are collapsed. When pH > 5.5, PAA segments are in an extended state, whereas P2VP segments are collapsed. The presence of terminal NH2 and COOH moieties facilitated the attachment of bioactive species, such as AMP and HEP, manifested by bacterial growth inhibition (AMP) and anticoagulant (HEP) properties. Compared to static surfaces, the presence of built-in pH sensitive dynamic surfaces also enhanced antimicrobial and anticoagulant properties, which is believed to be attributed to continuous surface morphology changes as well as the ability of AMP and HEP molecules to actively intercept microbial film formation and blood coagulation at early stages.
Acknowledgements
The authors thank the National Science Foundation (DMR 0215873), the J.E. Sirrine Foundation at Clemson University and Center for Optical Materials Science and Engineering Technologies (COMSET) at Clemson University for partial support of these studies. Prof. Elasri and his research group (USM; Department of Biological Sciences) are acknowledged for their help in conducting antimicrobial and anticoagulant tests. This work was partially conducted at the University of Southern Mississippi.
References
-
Biomaterials: Interfacial Phenomena and Applications, ed. S. L. Cooper and N. A. Peppas, American Chemical Society, Washington, DC, 1982; and references therein Search PubMed.
- J. H. Lee, H. B. Lee and J. D. Andrade, Prog. Polym. Sci., 1995, 20, 1043–1079 CrossRef CAS ; and references therein.
- W. Senaratne, L. Andruzzi and C. K. Ober, Biomacromolecules, 2005, 6, 2427–2448 CrossRef CAS PubMed ; and references therein.
- J. M. Goddard and J. H. Hotchkiss, Prog. Polym. Sci., 2007, 32(7), 698–725 CrossRef CAS PubMed.
- N. Houbenov, S. Minko and M. Stamm, Macromolecules, 2003, 36, 5897 CrossRef CAS.
- L. Ionov, N. Houbenov, A. Sidorenko and M. Stamm, Langmuir, 2004, 20, 9916 CrossRef CAS PubMed.
- F. Liu and M. W. Urban, Prog. Polym. Sci., 2010, 35, 3 CrossRef CAS PubMed.
- F. Liu and M. W. Urban, Macromolecules, 2008, 41, 6531 CrossRef CAS.
- F. Liu and M. W. Urban, Macromolecules, 2008, 41, 6531 CrossRef CAS.
- F. Liu, D. Ramachandran and M. W. Urban, Adv. Funct. Mater., 2010, 20, 3163 CrossRef CAS.
- Z. D. Hua, Z. Y. Chen, Y. Z. Li and M. P. Zhao, Langmuir, 2008, 24, 5773 CrossRef CAS PubMed.
- N. Aumsuwan, H. Y. Sang, W. R. Wagner and M. W. Urban, Langmuir, 2011, 27, 11106 CrossRef CAS PubMed.
- S. R. Gaboury and M. W. Urban, Langmuir, 1993, 9, 3225 CrossRef CAS.
- N. Aumsuwan, S. Heinhorst and M. W. Urban, Biomacromolecules, 2007, 8, 3525–3530 CrossRef CAS PubMed.
-
M. W. Urban, ATR Spectroscopy of Polymers - Theory and Practice, ACS, Washington D.C., 1996 Search PubMed.
-
I. Horcas, R. Fernandez, J. M. Gomez-Rodriguez, J. Colchero, J. Gomez-Herrero and A. M. Baro, WSXM: A Software for Scanning Probe Microscopy and a Tool for Nanotechnology Search PubMed.
- B. Herigstad, M. Hamilton and J. Heersink, J. Microbiol. Methods, 2001, 44, 121 CrossRef CAS.
-
N. Zelver, M. Hamilton, B. Pitts, D. Goeres, D. Walker, P. Sturman and J. Heersink, Measuring antimicrobial effects on biofilm bacteria: From laboratory to field, Academic Press, San Diego, 1999 Search PubMed.
- W. R. Premasiri, J. Lee and L. D. Ziegler, J. Phys. Chem. B, 2012, 166, 9376 CrossRef PubMed.
Footnotes |
† Electronic supplementary information (ESI) available. See DOI: 10.1039/c3bm60238a |
‡ It should be noted that the reason for the higher surface density of CAM on PE, compared to PTFE, is that the initial surface concentrations of COOH during microwave plasma reactions on PE is higher. As a result, subsequent steps involving CAM will be affected; the net result – slightly lower PTFE antimicrobial resistance. |
|
This journal is © The Royal Society of Chemistry 2014 |