DOI:
10.1039/C3BM60198F
(Paper)
Biomater. Sci., 2014,
2, 502-511
Ibuprofen-loaded electrospun fibrous scaffold doped with sodium bicarbonate for responsively inhibiting inflammation and promoting muscle wound healing in vivo
Received
22nd August 2013
, Accepted 4th November 2013
First published on 23rd December 2013
Abstract
Infection or inflammation can cause the in vivo microenvironment to become acidic, and an inappropriately excessive inflammatory response can significantly delay the healing process. A feasible acid-responsive ibuprofen-loaded poly(L-lactide) (PLLA) fibrous scaffold with doped sodium bicarbonate has been designed to prevent an excessive inflammatory response and promote regeneration. The results of the in vitro drug release study show that the acid-responsive fibrous scaffold exhibited a quick drug releasing response at pH 5.0 and a slow drug releasing response at pH 7.4. The in vivo rat muscle wound model study shows that the acid-responsive ibuprofen-loaded PLLA fibrous scaffold caused slighter inflammation and an earlier reparation. The immunohistochemical staining and quantitative real-time polymerase chain reaction analysis further showed that the scaffold caused lower levels of inflammatory factors and a higher expression of repair factors during the whole observation. Taken together, through the muscle wound healing process, the results demonstrate that the acid-responsive ibuprofen-loaded PLLA fibrous scaffold shows a better performance in preventing excessive inflammation than scaffolds that do not contain ibuprofen or are not acid-responsive. Additionally, the acid-responsive ibuprofen-loaded PLLA fibrous scaffold also has a faster healing process than others.
1. Introduction
Muscle repair is a complex process that includes three phases: (1) degeneration and inflammation, (2) muscle regeneration, and (3) fibrosis.1 The repair process is initiated immediately after injury by releasing of various growth factors and cytokines from the injured blood vessels and degranulating platelets,2 which are essential to the activation of leukocytes and the regeneration of tissues during the repair process.1,2 However, this cascade of events may become disrupted, leading to a delayed healing of acute wounds or the development of chronic nonhealing wounds/ulcers.3 Not only does failed healing have a profound local impact, but also intractable inflammation has been linked to multiple local and systemic disorders, from atherosclerosis to cancer.4 In addition, the inflamed area is characterized by acidic conditions, which are attributed partly to the increased lactate production by the anaerobic glycolysis of the inflamed cells and partly to the secretion of short-chain fatty acids from infected bacteria.5 Here we design a feasibly controllable acid-responsive release system as tissue scaffolds, which is used to intelligently regulate drug release in response to a change in the acidic microenvironment in regions where the pH is reduced below 7.4. This leads to the prevention of excessive inflammation at the early stage and promotion of tissue repair at the later stage. The combined treatment may have an effect on the prevention and treatment of infections or inflammation.
A variety of scaffolds have been fabricated based on materials ranging from naturally occurring ones to those manufactured synthetically, which could be used as combined treatment methods incorporating both drug carriers and tissue regeneration scaffolds.6,7 Recently, electrospinning has emerged as an efficient technique to produce a variety of polymeric nanofibers for tissue engineering and drug carriers.8,9 Electrospun fibers provide a high porosity and specific surface area, which enable them to be useful drug carriers for inhibiting diseased cells, and tissue engineering scaffolds for cell growth, leading to the efficient regeneration.10 Therefore, electrospun fibers are good candidates for treating lesions in the early stages as well as contributing to the repair of the tissue in the later stages. This is particularly important during muscle repair, when adjacent tissue needs support and anti-inflammatory agents need to be delivered locally for the degeneration and inflammation. Lesion tissue would also benefit from a material that could provide a drug-loaded scaffold for anti-inflammation and new cell growth.11
Most acid-responsive biodegradable polymers have been synthesized by modifying the polymers through introducing acid-liable segments,12 for example ortho ester groups,13 acetal groups,14 chitosan15 and so on. In spite of the realization of acid-responsiveness, harsh reaction conditions and multi-complicated synthetic processes were necessary, which limits their wide application. Furthermore, electrospun fibrous scaffolds quickly degrade because of the acid-responsive groups in the main chain of the polymer, which eliminated the three-dimensional (3D) scaffolds.13 Therefore, optimized acid-responsive fibers could not only act as drug carriers, but also are suitable for tissue engineering scaffolds.
Ibuprofen (IBU) is one kind of non-steroid antiphlogistic drug (NSAID) and its mechanism of action is the inhibition of prostaglandin synthesis by the prostaglandin H synthases (COX-1 and COX-2).16 Cyclooxygenase (COX) is the pivotal enzyme in prostaglandin biosynthesis. It exists in two isoforms, constitutive COX-1 (responsible for physiological functions) and inducible COX-2 (involved in inflammation).17 IBU is a well-known, effective, safe and well-tolerated anti-COX1 compound with anti-inflammatory,17 antipyretic and pain relief properties.18 The particularly active anti-inflammatory action of IBU has permitted its largely topical use in inflammatory conditions of the osteoarticular and muscles.19 Therefore we combined IBU in our acid-responsive controllable release system in order to control excessive inflammation in the early stages.
Previously, we fabricated IBU-loaded acid-responsive electrospun fibers by introducing sodium bicarbonate (SB) into poly(L-lactide) (PLLA) fibers using electrospinning technology.20 In this research, this type of acid-responsive electrospun fiber was further analysed in vivo by responsively inhibiting inflammation and promoting muscle wound healing in a mouse model (Scheme 1). This acid-responsive IBU-loaded electrospun fibrous scaffold was applied not only as a medicine carrier material for inhibiting early excessive inflammation but also as a scaffold for tissue engineering to accelerate muscle wound repair in Sprague-Dawley rats, which were inflicted with two incisions in the right and left antimere of the musculus sacrospinalis. The results may cast some light on the study and development of novel tissue-engineering materials.
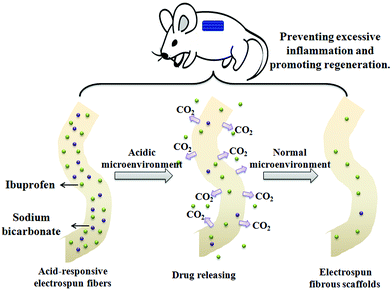 |
| Scheme 1 An illustration of the acid-responsive IBU-loaded electrospun fibrous scaffolds for inhibiting inflammatory cells and promoting tissue growth. | |
2. Materials and methods
2.1. Materials
PLLA (Mw = 100 kDa, Mw/Mn = 1.6) was purchased from Sigma. Dichloromethane (DCM), SB (NaHCO3) and N,N-dimethylformamide (DMF) were of reagent grade and were purchased from the Sinopharm Chemical Reagent Co., Ltd. All other chemicals and solvents were of reagent grade or better and were purchased from the Guo Yao Reagents Company (Shanghai, China), unless otherwise indicated.
2.2. Preparation of the electrospun fibrous scaffold
Electrospinning was carried out according to our previous report.21 PLLA (1.0 g) was completely dissolved in DCM (4 g) and DMF (2 g) for fabricating the electrospun PLLA fibers. To prepare the IBU-loaded PLLA electrospun fibrous membranes (PLLA–IBU), 1 g of PLLA and 40 mg of IBU were completely dissolved in a mixed-solvent system containing 4 g of DCM and 2 g of DMF for electrospinning. For the acid-responsive IBU-loaded electrospun fibers (PLLA–IBU–SB), 1 g of PLLA and 40 mg of IBU were dissolved in 4 g of DCM and 2 g of DMF, then 0.12 mL of NaHCO3 saturated water (96 mg mL−1) solution was added into the IBU/PLLA solution slowly with vigorous stirring for 30 min with a magnetic stirrer. The blended solvent was then put in a syringe pump that was attached to a high voltage device. A negative electrode blanketed with aluminum foil was used as the collector plate.
2.3.
In vitro drug-release study
The electrospun PLLA–IBU and PLLA–IBU–SB fibrous scaffolds (weight of 100.0 mg) were simultaneously immersed in centrifuge tubes containing 25 mL of phosphate buffered solution (PBS, pH = 7.4) or sodium acetate–acetic acid buffer solution (pH = 5.0).13 The samples were incubated at 37 °C for 48 h with mild shaking (100 rpm). At predetermined time intervals, 1 mL of buffer solution was collected and replaced with 1 mL of fresh buffer solution. The amount of released drug in the collected medium was determined by UV-vis spectroscopy at 224 nm. A standard calibration plot of IBU in the concentration range of 0–0.05 mg mL−1 was used to determine the concentration of the drug released. The percentage of the released drug was then calculated based on the initial weight of the drug incorporated in the electrospun scaffolds.
2.4. Animal and experimental design
Male 8-week-old Sprague-Dawley rats (n = 50) weighing 200–250 g were obtained from the Animal Breeding Center, Shanghai Sixth People's Hospital affiliated to Shanghai Jiao Tong University, China. The rats were housed in individual boxes maintained at a constant temperature (22 ± 2 °C) and humidity (55%). They had free access to commercial chow and tap water, and were subjected to a 12 hour light/dark cycle. All surgical procedures were performed under sterilized conditions and under the guidelines stipulated by the ethical committee of the Shanghai Jiao Tong University Laboratory Animal Center. The rats were allowed to acclimatize for one week before the beginning of the experiment.
The animals were divided into two groups (25 rats in each group) and anesthetized using ketamine (1 mL kg−1 of the body weight). The backs of the animals were shaved and sterilized with 70% EtOH. Each group received two incisions in the right and left antimere of the musculus sacrospinalis, separated by a distance of 2 cm. In group one, the right lesion was untreated as a negative control (control group) and the left lesion was treated with the electrospun fibrous scaffolds (PLLA group). In group two, the left lesion was treated with the IBU-loaded electrospun fibrous scaffolds (PLLA–IBU group) and the right lesion was treated with the acid-responsive IBU-loaded electrospun fibrous scaffolds (PLLA–IBU–SB group). The animals in each group were evaluated daily but euthanized on the 1st, 3rd, 7th, 14th and 21st day (n = 5) post wounding. The muscle tissue samples from the wound site were excised in full depth and bisected from all of the rats for morphologic observation and gene expression level tests.
2.5. Histological analysis
All wound tissue specimens were fixed in 10% formalin for at least 24 hours at room temperature, dehydrated in ethanol, cleared in xylene, and further embedding in paraffin. The above-treated specimens were then sectioned (3 mm) and stained with hematoxylin and eosin for histological observation. As part of the histological evaluation, all slides were examined by a pathologist, without knowledge of the previous treatment, under a light microscope. The cellular density of the inflamed cells and the structure of the muscle tissues were observed.
2.6. Immunohistochemical staining
The paraffin-embedded sections were deparaffinized with xylene, dehydrated in decreasing concentrations of EtOH, and then treated with 3% hydrogen peroxide in methanol for 10 minutes to block endogenous peroxidase activity. Tissue sections were processed in 10 mM citrate buffer (pH = 6.0) and heated to 100 °C for 10 minutes for antigen retrieval. The primary antibodies were diluted to 1
:
300, 1
:
200, 1
:
100, and 1
:
200 in 0.05 M PBS (pH = 7.2) for IL-6, TNF-α, TGF-β1 and VEGF, respectively, then stored at 4 °C overnight. The sections were then incubated with rabbit antibodies anti-IL-6, -TNF-α, -TGF-β1 and -VEGF for 1 hour at 37 °C. The peroxide reactions were carried out with 3,3′-diaminobenzidine tetrahydrochloride (DAB) as the chromogens, and the slides were counterstained with haematoxylin.
2.7. RNA preparation and reverse transcription
The total RNA was extracted from frozen tissues with Trizol (Invitrogen, Carlsbad, CA), according to the manufacturer's instructions and quantified at a wavelength of 260 nm by NanoDrop spectrophotometry (Eppendorf, Hamburg, Germany).22 The integrity of the RNA was verified by the optical density absorption ratio OD260 nm/OD280 nm between 1.8 and 2.0. To obtain the cDNA, 2 μg of the total RNA was reverse transcribed using M-MLV reverse transcriptase (Promega, Madison, USA), followed by amplification on an Applied Biosystems ViiA™ 7 system (Applied Biosystems Inc., Foster City, CA).
2.8. Quantitative real-time PCR
A quantitative real-time polymerase chain reaction (qRT-PCR) using an Applied Biosystems ViiA™ 7 system (Applied Biosystems Inc., Foster City, CA) was applied for detecting the relative IL-6, TNF-α, TGF-β1 and VEGF mRNA levels in rat muscle. The relative expression levels of those gene transcripts were normalized to RNA loading for each sample using b-actin mRNA. Specific primers for all genes were designed by bioTNT (Shanghai, China) and manufactured by Invitrogen (Carlsbad, CA). The sequences of sense and antisense primers for target genes and the reference gene (b-actin) are shown in Table 1. The qRT-PCR reactions were performed in a final volume of 20 μL containing 1 μL of cDNA. The reactions were performed with the following conditions: 5 min of pre-incubation at 95 °C, followed by 40 cycles for 5 s at 95 °C and 30 s at 60 °C. All qRT-PCR reactions were performed in triplicate. A reaction without cDNA was performed in parallel as a negative control. Relative quantification was performed according to the comparative 2−ΔΔCt method, as described previously.23 The result for the gene expression was given as a dimensionless value using the formula 2−ΔΔCt.
Table 1 Different gene specific primers
|
Sense |
Antisense |
IL-6 |
5′-T ATG AAC AGC GAT GAT G-3′ |
5′-C CAG GTA GAA ACG GAA CTC-3′ |
TNF-α |
5′-T CTG TGT CCT TCT AAC T-3′ |
5′-C TAC TTC AGC GTC TCG TGT-3′ |
TGF-β1 |
5′-GGA CCT GGG TTG GAA GT-3′ |
5′-G GTT GTG TTG GTT GTA GAG-3′ |
VEGF |
5′-GCG TCT ATC TGT AGG TG-3′ |
5′-GGT GTC TGG GGG ATG TTC-3′ |
b-Actin |
5′-CCT CTA TGC CAA CAC AGT-3′ |
5′-AGC CAC CAA TCC ACA CAG-3′ |
2.9. Statistical analysis
All data were recorded as the mean ± the standard deviation. The differences between the tested groups and control group were analyzed using the unpaired t-test (SAS 9.1 for Windows). Moreover, the differences among multiple groups and control group were analyzed using ANOVA. A P value <0.05 was statistically considered to be significant. All experiments were performed at least three times.
3. Results
3.1. Fibrous scaffold characterization
Fig. 1 shows the morphology and fiber diameter distributions of the electrospun fibrous scaffolds. It can be seen that there were no beads in the fibrous structure and the fibers were uniform in size, and randomly interconnected. The diameters of fibers were 1.29 ± 0.35, 1.35 ± 0.28 and 1.13 ± 0.42 μm for the PLLA, PLLA–IBU and PLLA–IBU–SB electrospun fibrous scaffolds, respectively.
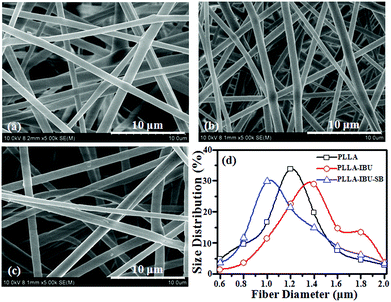 |
| Fig. 1 SEM photographs of the electrospun PLLA (a), PLLA–IBU (b), and PLLA–IBU–SB (c) fibers; the fiber diameter distributions (d) of the three samples. | |
3.2.
In vitro drug release study
The actual loading content of IBU was determined to be close to the theoretic value for the IBU-loaded electrospun fibers. Drug loading contents of 3.91 ± 0.22% and 3.87 ± 0.31% were detected for the PLLA–IBU and PLLA–IBU–SB fibers with an initial addition of 4.0% (IBU/PLLA, w/w). The values were not significantly different for the two groups. The in vitro release profile of the IBU-loaded electrospun fibrous scaffolds in pH 7.4 and pH 5.0 buffer solutions are shown in Fig. 2. The total amounts released were around 18.6% and 32.1% for the PLLA–IBU and PLLA–IBU–SB fibers, respectively, after 48 h of incubation in pH 7.4 buffer solutions (Fig. 2a). As shown in Fig. 2b, the amount of drug released from the PLLA–IBU–SB fibers at pH 5.0 was significant higher than from the PLLA–IBU fibers. The total amounts of released IBU were approximately 30.6% and 78.2% for the PLLA–IBU and PLLA–IBU–SB fibers, respectively, after incubation for 48 h in pH 5.0 buffer solutions. The release rate of the loaded IBU was accelerated with NaHCO3 in the fibers in the acidic solution.
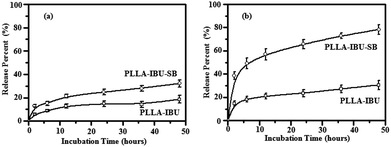 |
| Fig. 2 The in vitro IBU release profiles of the electrospun PLLA fibrous scaffolds in pH 7.4 (a) and pH 5.0 (b) buffer solutions. | |
Fig. 3 shows the SEM morphologies of the electrospun PLLA–IBU and PLLA–IBU–SB fibrous scaffolds after incubation for 48 h at pH 7.4 and pH 5.0. The diameters of the fibers increased to 1.83 ± 0.31, 1.97 ± 0.33 and 1.30 ± 0.47 μm in pH 7.4 PBS, and 1.96 ± 0.41, 2.11 ± 0.38 and 1.41 ± 0.51 μm in pH 5.0 PBS for the PLLA, PLLA–IBU and PLLA–IBU–SB electrospun fibrous scaffolds, respectively. Compared with the original formation (Fig. 1), the structures of the electrospun fibrous scaffolds after incubation for 48 h at pH 7.4 and pH 5.0 maintained a 3D fibrous structure, even though all the fibers were swollen slightly compared with the original formation. Therefore, all the electrospun fibrous scaffolds could act as tissue engineering scaffolds and could contribute to the proliferation of normal cells after the treatment of lesions.
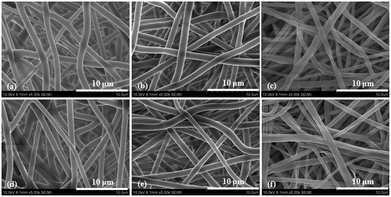 |
| Fig. 3 SEM images of the electrospun PLLA (a and d), PLLA–IBU (b and e), and PLLA–IBU–SB (c and f) fibrous scaffolds after the in vitro release in pH 7.4 (first row) and pH 5.0 (second row) buffer solutions for 48 h. | |
3.3. Histological analysis of wound healing
Histologic analysis was performed to determine the cellular effects on wounds following the topical application of the different fibrous scaffolds. As shown in Fig. 4, in the control group, there was a trend toward greater inflamed cells on day 3. At this stage we noticed lots of inflamed cells infiltrated in the intermuscular septum, which led to the derangement of the intermuscular septum. In the PLLA group, burst inflamed cells appeared on day 7, and this phenomenon in both the control group and the PLLA group continued to day 14. In the PLLA–IBU–SB group and the PLLA–IBU group, the greatest inflamed cell infiltration appeared on day 3, but to a smaller degree compared to the control group and the PLLA group. Especially for the PLLA–IBU–SB group, the control of the inflammatory reaction was better than the PLLA–IBU group. On day 7 the inflamed cell infiltration became less in the PLLA–IBU–SB and PLLA–IBU groups, and the structure of the intermuscular septum became obvious. In the control group and the PLLA group, the muscle fibers had a normal morphology and a regular arrangement on day 21, while in the PLLA–IBU–SB and PLLA–IBU groups this happened on day 14 and the muscle tissues became completely normal on day 21.
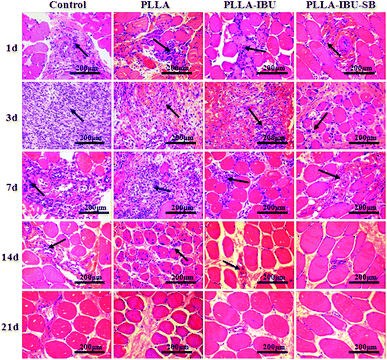 |
| Fig. 4 Histology of wounded muscle tissues: H&E staining after 1–21 days. | |
3.4. Changes in the immunohistology characteristics of wound healing
In the immunohistochemical experiments, the method of assessment is mainly to observe the changes in the organizational structures. The positive immunohistochemical cells displayed a brown-yellow stain within the cytoplasm. The morphometric analysis of the inflammatory response, which was stained by IL-6 and TNF-α, is shown in Fig. 5 and 6. As shown in Fig. 5, in the control group, the expression of IL-6 had an increased trend from day 1 to day 3, and began to decline from day 7. In the PLLA group, the level of IL-6 increased from the first day to the seventh day, and then decreased weekly. In the PLLA–IBU and PLLA–IBU–SB groups, the expression of IL-6 was burst on day 3, but it was to a smaller degree compared to the control group and the PLLA group. Especially in the PLLA–IBU–SB group, the level of IL-6 began to dramatically decline from day 7. The detection of TNF-α, as shown in Fig. 6, indicated that the level of TNF-α had an increased trend from day 1 to day 3 in all groups, while from day 7 the expression became different. In the control group, the level of TNF-α was still high on day 7 and lasted until day 14, while in the other three groups the expression of TNF-α decreased weekly from day 7, and within these three groups, the PLLA–IBU–SB group had the most significant decline.
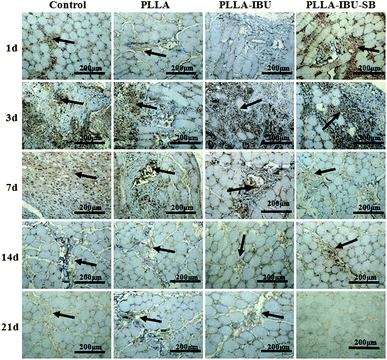 |
| Fig. 5 Immunohistochemical detection of wounded muscle tissues by stained IL-6. | |
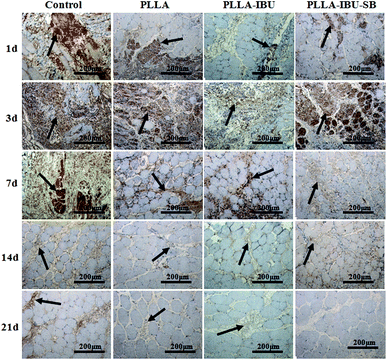 |
| Fig. 6 Immunohistochemical detection of wounded muscle tissues by stained TNF-α. | |
The morphometric analysis of the tissue repair, which was stained by VEGF and TGF-β1, is shown in Fig. 7 and 8. As shown in Fig. 7, the wounded tissues stained by VEGF demonstrated an increased trend in the number of blood vessels in all groups from day 1 to day 14, and the expression of VEGF in the PLLA–IBU–SB group displayed the highest level during the whole observation. On the 21st day after the operation, the positive expression cells of VEGF in all the groups weakened, but were still present. The stain for TGF-β1, as shown in Fig. 8, showed that on the 1st and 3rd days after the operation there was no manifest of positive expression of TGF-β1 in the tissues of all groups. On the 7th and 14th days after the operation, in the PLLA–IBU–SB group, distinct positive-expression cells were found in the spatium intermusculare. On the 21st day after the operation, the positive-expression cells of TGF-β1 in the PLLA–IBU–SB group decreased. In the other three groups, this trend was also seen, but the expressions of TGF-β1 were lower than that in the PLLA–IBU–SB group at every corresponding time.
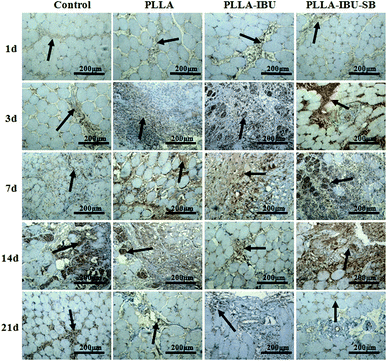 |
| Fig. 7 Immunohistochemical detection of wounded muscle tissues by stained VEGF. | |
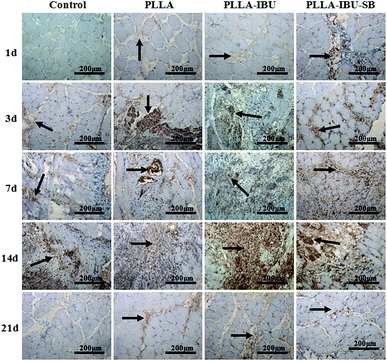 |
| Fig. 8 Immunohistochemical detection of wounded muscle tissues by stained TGF-β1. | |
3.5. Pro-inflammatory and -regeneration factors gene expressions in wound healing
The relative expression of IL-6 was significantly different in the four groups (P < 0.05), as shown in Fig. 9. In the control group, the level of IL-6 had the highest expression on days 1 and 3 compared to the others, and then decreased weekly. In the PLLA group, the level of IL-6 had the burst expression on day 7, and was even higher than the control group on day 3. As for the PLLA–IBU–SB group and the PLLA–IBU group, the expression of IL-6 was lower at every observed time. Combined with Fig. 9 and the statistical data, we found that the control group and the PLLA group were different compared to the PLLA–IBU–SB and PLLA–IBU groups. There was also a difference between the PLLA–IBU–SB group and the PLLA–IBU group, while no difference between the control group and the PLLA group was observed. As shown in Fig. 10, the concentration of TNF-α increased in a time-dependent manner during the first 14 days in the control group, while this trend stopped on day 7 in the PLLA and PLLA–IBU groups, and in the PLLA–IBU–SB group the highest expression was on day 3 with the lowest level among all the peak values (P < 0.05). These results indicated that the animals treated with the acid-responsive IBU-loaded electrospun fibrous scaffolds showed significantly lower levels of IL-6 and TNF-α compared with the other groups, which means that the inflammatory response in the PLLA–IBU–SB group was lower than the other groups.
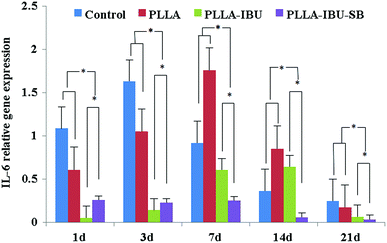 |
| Fig. 9 The qRT-PCR analysis of the IL-6 expression in the different treatment groups after wound formation. Each bar represents the mean value ± SE from experiments performed in triplicate. P < 0.05 represents a significant difference between different groups. | |
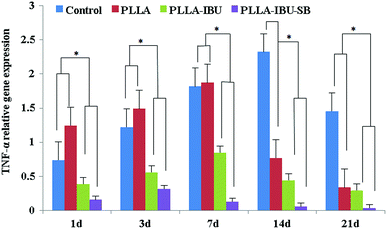 |
| Fig. 10 The qRT-PCR analysis of the TNF-α expression in the different treatment groups after wound formation. Each bar represents the mean value ± SE from experiments performed in triplicate. P < 0.05 represents a significant difference between different groups. | |
As for the pro-regeneration factors, from Fig. 11, we can see that the expression of VEGF increased smoothly till day 14 in all groups, and declined on the 21st day. However, the expression of VEGF in the PLLA–IBU–SB group was at a significantly higher level than the others from the 7th day and kept this superiority till the 21st day. In the PLLA–IBU and PLLA groups, the expression of VEGF became higher than the control group from the 3rd day till the last observation, and on day 3 the expression of VEGF in the PLLA–IBU group was the highest. Therefore, there was a significant difference between the four groups and at different times (P < 0.05). As shown in Fig. 12, the expression of TGF-β1 ascended in sequence from the control group, PLLA group, PLLA–IBU group, to PLLA–IBU–SB group, starting from the 1st day after the operation. On the 7th day, the expression of TGF-β1 increased dramatically in the PLLA–IBU and PLLA–IBU–SB groups, and maintained this advanced expression till the 21st day. The key point is that the expression of TGF-β1 in the PLLA–IBU–SB group was constantly higher than the others during the whole observation (P < 0.05). These results suggest that the animals treated with the acid-responsive IBU-loaded electrospun fibrous scaffolds showed significantly higher levels of VEGF and TGF-β1 compared with the other groups, which means that the tissue repair process in the PLLA–IBU–SB group was earlier and faster than in the other groups.
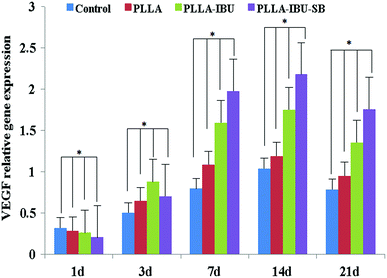 |
| Fig. 11 The qRT-PCR analysis of the VEGF expression in the different treatment groups at 1, 3, 7, 14 and 21 days after wound formation. Each bar represents the mean value ± SE from experiments performed in triplicate. P < 0.05 represents a significant difference between different days. | |
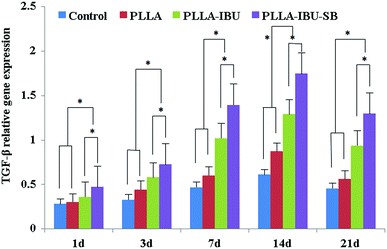 |
| Fig. 12 The qRT-PCR analysis of the TGF-β1 expression in the different treatment groups at 1, 3, 7, 14 and 21 days after wound formation. Each bar represents the mean value ± SE from experiments performed in triplicate. P < 0.05 represents a significant difference between different days. | |
4. Discussion
Autogenous tissues are generally preferred as the first choice in reconstructive surgery. However, they may have some drawbacks such as a limited tissue source, difficulty in molding, donor site morbidity, and unpredictable long term resorption rates. These shortcomings have made alloplastic materials valuable alternative sources in reconstructive procedures.24 An improved material can enhance both the rapidity of the healing process and the quality of the outcome, including reducing infection, pain, and scarring.25 In general, an ideal material for wound healing would have the following properties: (1) be a cytocompatible natural or artificial material; (2) have minimal inflammatory effects, disease transmission and host immune response; and (3) have an optimal micro-architecture to promote efficient cell infiltration and attachment.26 In this paper, we report a simple way to fabricate acid-responsive electrospun fibrous scaffolds as drug carriers that are effective for the early treatment of lesion tissue due to the pH-responsiveness of the fibers, and also contribute to the repair of the tissue as scaffolds for cell growth in the later period. Compared with existing drug carriers, our proposed drug carriers have the following advantages. Firstly, they can realize an acid-responsive release through a simple process, without the need for specific responsive groups or synthesis steps. Secondly, they not only act as a drug carrier to release drugs for the treatment of lesion tissue in the early stage, but they also play the role of cell scaffolds in the later stage because of the integrity of the 3D fibrous structure.
The key component of these drug carriers is SB, which can be incorporated into the electrospun fibers with an anti-inflammatory drug using emulsion electrospinning. Once the acidic solution (pH 5.0) entered the polymer bulk, NaHCO3 reacted with the acid, which caused the generation of CO2 from the fibers. This, in turn, caused the loaded-IBU to be removed from the polymer bulk and released from the fibers. As shown in Fig. 2, in acidic conditions, such as inflammation, the drug release rate from the PLLA–IBU–SB fibers was higher than from the PLLA–IBU fibers. Comparing the release profiles in pH 7.4 and 5.0 buffer solutions, the electrospun PLLA–IBU fibrous scaffolds released approximately 30.6% at pH 5.0, which was 12.0% higher than the drug released at pH 7.4. Approximately 78.2% of the total loaded IBU was released after 48 h from the electrospun PLLA–IBU–SB fibrous scaffolds at pH 5.0, which was almost 2.4 times as much as the drug released at pH 7.4, with an initial burst of approximately 57.2% in the first 12 h. Therefore, as the PLLA–IBU–SB group had a higher IBU release burst than the PLLA–IBU group at pH 5.0, the inflammatory reaction was controlled better in the PLLA–IBU–SB group than in the others. The high initial burst and the acid-responsive release profile of IBU from the PLLA–IBU–SB fibers may be explained by the effect of NaHCO3. This is the great advantage over other drug delivery systems, which cannot release drugs responsively according to the surrounding microenvironment, though they can result in anti-inflammation and accelerate wound repair as well.27,28
As we know, inflammation is a complex defence mechanism characterized by leukocyte migration from the vasculature into the damaged tissues, and the subsequent deposition of extracellular matrix for tissue repair.29 An excessive deposition of extracellular matrix can lead to overgrowth, hardening, and/or scarring of tissues.30 As for IBU, it has specific activity against neutrophils, including the inhibition of neutrophil migration, which has been demonstrated in previous studies.31,32 Moreover, high concentrations of IBU inhibit the activation of NF-kB and AP-1, two important proinflammatory transcription factors.33 Thus IBU should be considered as an anti-inflammatory agent, much like corticosteroids. What is more, due to its fewer adverse effects compared to corticosteroids, IBU is more suitable for long-term use. From the in vivo results, we found that there was no significant difference in the inflammation process between the control group and the PLLA group, which means that the electrospun fibrous scaffolds cannot reduce the inflammatory reaction (Fig. 4). On the other hand, the good aspect is that the electrospun fibrous scaffolds do not aggravate infection or the inflammatory reaction. The attractive result in our study is that the PLLA–IBU–SB group had a lighter inflammatory reaction than the PLLA–IBU group, owing to the NaHCO3 causing a high initial burst of IBU from the PLLA–IBU–SB fibers in the acidic environment.
Previous studies have reported that IBU has been loaded in polyvinyl alcohol (PVA) hydrogel microspheres to inhibit inflammatory reactions.27 IBU-loaded alginate gel,28 solvent-casting PELA film34 and hyaluronic acid gel35 have also been used as physical barriers and drug delivery systems to reduce tissue adhesions and inhibit inflammation. However, these materials rapidly degrade and have limited use for muscle repair because they are not stable 3D structure scaffolds. The present study focuses on treating muscle wounds with acid-responsive IBU-loaded PLLA fibrous scaffolds. Our histological examination showed that the infiltration of inflamed cells happened to a smaller degree and with a quicker recession in the PLLA–IBU–SB group compared to the other three groups. The immunohistochemical staining, which was verified by qRT-PCR analysis, showed that the expressions of the pro-inflammatory factors, IL-6 and TNF-α, were significantly decreased in the PLLA–IBU–SB-treated group compared with the other groups. The results are consistent with other studies.1 As for the pro-regeneration factors, VEGF and TGF-β1, there were some similarities in their behavior, namely, the expressions increased gradually until the 14th day in all groups, and then declined on the 21st day. However, Lee et al.2 reported the significantly decreased expressions of TGF-β1 and VEGF in an alginate-treated group compared with the control group. Park et al.36 demonstrated that the expression levels of TGF-β1 and VEGF decreased when treated with microbial cellulose, which can reduce the initial inflammatory reaction and accelerate wound healing, compared with the control group on day 14 after skin excision. However, one study reported an increased VEGF expression at days 7 and 14,37 which corroborates with our findings. Additionally, another study confirmed that on the 7th day after the wound was formed, the expression of TGF-β1 significantly increased in the group with the astragaloside IV gel, compared with the blank control.38 According to Khanna et al.39 angiogenesis plays a central role in wound healing and the VEGF plays an important role in the stimulation of angiogenesis in wounds. As far as the TGF-β1 is concerned, it is well known that cytokine initiates the wound healing phases of inflammation, angiogenesis, reepithelialization, and connective tissue regeneration, and it can up-regulate the VEGF as well.38 In view of the important roles of the VEGF and TGF-β1 in wound repair, and the inconsistent results from different studies, the expressions and functions of the VEGF and the TGF-β1 in various wounds need further research.
In this study, the morphology and molecular biology analysis demonstrates that the acid-responsive IBU-loaded PLLA fibrous scaffolds not only act as drug carriers to prevent excessive inflammation but also act as tissue scaffolds to improve muscle regeneration. The relatively simple technological process and the cheaper cost of production, combined with the great biocompatibility and biodegradability, makes the acid-responsive IBU-loaded PLLA fibrous scaffolds a good choice for healing muscle wounds in this research.
In this paper, we resolve the initial problem of controlling excessive inflammation responsively. However, some problems still exist in our study, such as the degradation of the PLLA scaffolds themselves can create acidic conditions since the degradation products will be lactic acid molecules and they may enhance the inflammation in the later stages. Hence, we will design further experiments to resolve this problem in our future work.
5. Conclusion
A feasible acid-responsive electrospun fibrous scaffold was easily fabricated based on NaHCO3 for curing muscle wounds. The in vitro drug release results indicated that there was a quicker drug releasing response for the PLLA–IBU–SB fibers than for the PLLA–IBU fibers in pH 5.0 PBS, and all fibers maintained their stable fibrous structures. Combined with the results from the histological and immunohistochemical analyses and the qRT-PCR data, the in vivo rat muscle wounds showed that: (1) both the IBU-loaded groups had a lower inflammatory response compared to the non-IBU-loaded groups. Specifically, the PLLA–IBU–SB group had the better performance in this aspect than the PLLA–IBU group; (2) with respect to their use as drug carriers and cell scaffolds, the electrospun fibrous scaffolds showed good biocompatibility because there was no significant difference in inflammatory response between the PLLA group and the negative control in the qRT-PCR results; (3) the repair factors in the control group were lower than that in the other three groups, and the expressions of the VEGF and TGF-β1 in the PLLA–IBU and PLLA–IBU–SB groups were significantly higher than that in the other groups from the 7th day and this was maintained until the 21st day. Particularly, the expressions of the VEGF and TGF-β1 in the PLLA–IBU–SB group were even higher than that in the PLLA–IBU group. In one sentence, the acid-responsive IBU-loaded electrospun fibrous scaffolds can prevent excessive inflammation and speed up the healing process, and can be considered as a new type of tissue-engineering artificial drug carrier and cell scaffold.
Acknowledgements
This work was supported by the National Natural Science Foundation of China (51373112, 51003058 and 81272935) and the Program for SMC-Young Scholars of Shanghai Jiao Tong University (SJTU). We thank Miao Wang (Shanghai International Studies University) for helpful language revision.
Notes and references
- R. A. Mesquita-Ferrari, M. D. Martins, J. A. Silva Jr., T. D. da Silva, R. F. Piovesan and V. C. S. Pavesi,
et al.
, Lasers Med. Sci., 2011, 26, 335–340 CrossRef PubMed
.
- W. R. Lee, J. H. Park, K. H. Kim, S. J. Kim, D. H. Park and M. H. Chae,
et al.
, Wound Repair Regener., 2009, 17, 505–510 CrossRef PubMed
.
- A. Techasen, N. Namwat, W. Loilome, P. Bungkanjana, N. Khuntikeo and A. Puapairoj,
et al.
, Med. Oncol., 2012, 29, 3083–3091 CrossRef CAS PubMed
.
- M. Schäfer and S. Werner, Nat. Rev. Mol. Cell Biol., 2008, 9, 628–638 CrossRef PubMed
.
- A. Lardner, J. Leukoc. Biol., 2001, 69, 522–530 CAS
.
- S. Zhong, Y. Zhang and C. Lim, Wiley Interdiscip. Rev., 2010, 2, 510–525 CAS
.
- G. Sun and J. J. Mao, Nanomedicine, 2012, 7, 1771–1784 CrossRef CAS PubMed
.
- S. Liu, C. Hu, F. Li, X. Li, W. Cui and C. Fan, Tissue Eng. A, 2012, 19, 529–537 CrossRef PubMed
.
- S. Liu, M. Qin, C. Hu, F. Wu, W. Cui and T. Jin,
et al.
, Biomaterials, 2013, 34, 4690–4701 CrossRef CAS PubMed
.
- S. Liu, J. Zhao, H. Ruan, T. Tang, G. Liu and D. Yu,
et al.
, Biomacromolecules, 2012, 13, 3611–3619 CrossRef CAS PubMed
.
- C. Hu and W. Cui, Adv. Healthcare Mater., 2012, 1, 809–814 CrossRef CAS PubMed
.
- R. Salehi, M. Irani, M. Rashidi, A. Aroujalian, A. Raisi and M. Eskandani,
et al.
, Des. Monomers Polym., 2013, 1–13 Search PubMed
.
- M. Qi, X. Li, Y. Yang and S. Zhou, Eur. J. Pharm. Biopharm., 2008, 70, 445–452 CrossRef CAS PubMed
.
- W. Cui, M. Qi, X. Li, S. Huang, S. Zhou and J. Weng, Int. J. Pharm., 2008, 361, 47–55 CrossRef CAS PubMed
.
- R. Cheng, F. Meng, C. Deng, H. Klok and Z. Zhong, Biomaterials, 2013, 34, 3647–3657 CrossRef CAS PubMed
.
- K. Rainsford, Inflammopharmacology, 2009, 17, 275–342 CrossRef CAS PubMed
.
- M. Milani and P. Iacobelli, Obstet. Gynecol., 2012, 2012, 673131 Search PubMed
.
- C. A. Pierce and B. Voss, Ann. Pharmacother., 2010, 44, 489–506 CrossRef CAS PubMed
.
- H. G. Elizabeth, Evidence-Based Nurs., 2009, 12, 25 CrossRef PubMed
.
- J. Zhao, S. Liu, B. Li, H. Yang, C. Fan and W. Cui, Macromol. Biosci., 2013, 13, 885–892 CrossRef CAS PubMed
.
- W. Cui, X. Li, X. Zhu, G. Yu, S. Zhou and J. Weng, Biomacromolecules, 2006, 7, 1623–1629 CrossRef CAS PubMed
.
- K. J. Livak and T. D. Schmittgen, Methods, 2001, 25, 402–408 CrossRef CAS PubMed
.
- S. Givvimani, C. Munjal, N. Tyagi, U. Sen, N. Metreveli and S. C. Tyagi, PLoS One, 2012, 7, e32388 CAS
.
- T. Cavusoglu, I. Vargel, I. Yazici, M. Cavusoglu and A. C. Vural, Ann. Plast. Surg., 2010, 64, 41–46 CrossRef CAS PubMed
.
- H. U. Zaman, J. Islam, M. A. Khan and R. A. Khan, J. Mech. Behav. Biomed. Mater., 2011, 4, 1369–1375 CrossRef CAS PubMed
.
- L. Baoyong, Z. Jian, C. Deng and L. Min, Burns, 2010, 36, 891–896 CrossRef PubMed
.
- M. Wassef, J. P. Pelage, E. Velzenberger, J. Namur, I. Schwartz-Cornil, R. R. Taylor, A. L. Lewis and A. Laurent, J. Biomed. Mater. Res., Part B, 2008, 86, 63–73 CrossRef PubMed
.
- S. H. Oh, J. K. Kim, K. S. Song, S. M. Noh, S. H. Ghil, S. H. Yuk and J. H. Lee, J. Biomed. Mater. Res., Part A, 2005, 72, 306–316 CrossRef PubMed
.
- M. Aller, J. Arias, M. Nava and J. Arias, Exp. Biol. Med., 2004, 229, 170–181 CAS
.
- A. Silini, O. Parolini, B. Huppertz and I. Lang, Curr. Stem Cell Res. Ther., 2013, 8, 6–14 CrossRef CAS
.
- M. W. Konstan, K. M. Vargo and P. B. Davis, Am. J. Respir. Critic. Care Med., 1990, 141, 186–192 CrossRef CAS PubMed
.
- M. W. Konstan, J. E. Krenicky, M. R. Finney, H. L. Kirchner, K. A. Hilliard and J. B. Hilliard,
et al.
, J. Pharmacol. Exp. Ther., 2003, 306, 1086–1091 CrossRef CAS PubMed
.
- J. F. Chmiel and M. W. Konstan, Clin. Chest Med., 2007, 28, 331–346 CrossRef PubMed
.
- J. H. Lee, A. K. Go, S. H. Oh, K. E. Lee and S. H. Uk, Biomaterials, 2005, 26, 671–678 CrossRef CAS PubMed
.
- J. A. Miller, R. L. Ferguson, D. L. Powers, J. W. Burns and S. W. Shalaby, J. Biomed. Mater. Res., 1997, 38, 25–33 CrossRef CAS
.
- S. U. Park, B. K. Lee, M. S. Kim, K. K. Park, W. J. Sung and H. Y. Kim,
et al.
, Int. Wound J., 2012, 5 DOI:10.1111/j.1742-481X.2012.01035.x
.
- W. S. Pessoa, L. R. Estevão, R. S. Simões, M. E. Barros, Fde. S. Mendonça and L. Baratella-Evêncio,
et al.
, Acta Cir. Bras., 2012, 27, 655–670 CrossRef PubMed
.
- L. H. Peng, X. Chen, L. Chen, N. Li, W. Q. Liang and J. Q. Gao, Biol. Pharm. Bull., 2012, 35, 881–888 CAS
.
- S. Khanna, M. Venojarvi, S. Roy, N. Sharma, P. Trikha and D. Bagchi,
et al.
, Free Radical Biol. Med., 2002, 33, 1089–1096 CrossRef CAS
.
Footnote |
† These authors contributed equally to this work. |
|
This journal is © The Royal Society of Chemistry 2014 |