DOI:
10.1039/C3BM60236B
(Paper)
Biomater. Sci., 2014,
2, 522-529
Design and use of silica-containing redox nanoparticles, siRNPs, for high-performance peritoneal dialysis†
Received
2nd October 2013
, Accepted 4th December 2013
First published on 7th January 2014
Abstract
The prevention of encapsulating peritoneal sclerosis (EPS) and the enhancement of dialysis efficiency are two important strategies that can improve the quality of life of patients undergoing peritoneal dialysis. We have thus far developed bionanoparticles that effectively scavenge reactive oxygen species (redox nanoparticles; RNPs). The objective of this study was to apply RNPs as a component of dialysate to reduce oxidative stress. Porous silica nanoparticles were combined with RNPs to enhance the effective adsorption capacity of low-molecular weight (LMW) compounds. The silica-containing RNPs (siRNPs) were confirmed to statistically decrease the level of creatinine and blood urea nitrogen in vivo. EPS model rats that underwent an intraperitoneal injection of chlorhexidine gluconate exhibited dysfunction of the peritoneal membrane. siRNP administration did not result in dysfunction of the peritoneal membrane. An LMW nitroxide compound, TEMPOL, also showed a weak peritoneal protective effect, although its efficiency was limited. No blood uptake of siRNPs was observed when they were administered into the peritoneal cavity. However, LMW-TEMPOL diffused into the blood stream, which might have decreased its effective concentration in the peritoneal cavity and led to adverse effects across the entire body. Considering these results, siRNPs are expected to be a new multi-functional nanomaterial for high performance peritoneal dialysis.
1. Introduction
Recently, technological developments in renal replacement therapy (RRT) have been used to successfully treat patients with renal failure. By the end of 2010, 2.03 million patients were undergoing RRT. Nearly 90% of these patients received hemodialysis (HD) therapy, while worldwide only 8.4–11% were treated with peritoneal dialysis (PD).1 Although HD substitutes for a portion of renal function, there still remain several issues such as (1) the continuous need for hospital attendance, which limits the social activities of patients; (2) insufficient removal of medium molecular weight uremic toxins; and (3) the removal of body fluid in a short time thereby leading to cardiac overload and vascular damage.2 These issues increase the patient risk for several serious diseases such as stroke and myocardial infarction.3 In contrast, the following reasons make PD the first choice of RRT in many countries: (1) low load in terms of medical economies;4 (2) social precedence during domiciliary treatment; and (3) medical advantages with respect to patient outcome.5 For the last reason in particular, current studies are successively revealing the advantages of the PD-first policy as they relate to residual renal function and survival rates.6 For example, dialysate can be changed by oneself. In addition, rehabilitation is easy, it maintains renal function, and the risk of stroke and myocardial dysfunction is low. Thus, PD has much potential to provide a high quality of life to patients who are undergoing RRT.
However, the long-term outcome of PD is still poorer than that of HD. Two major reasons for this are (1) the insufficiency of dialysis due to the loss of peritoneal function, which thus increases changes in dialysate; and (2) the occurrence of encapsulating peritoneal sclerosis (EPS), which is a fatal complication of PD.7 Chronic inflammation of the peritoneal membrane leads to encapsulation of the intestine, which then results in severe ileus and malnutrition in EPS patients.8 The duration of PD is intricately related to EPS, whereby more than 3–8 years of PD history remarkably enhances the risk of EPS and leads to the termination of PD and switching over to HD.9 Dialysate with a high glucose concentration causes oxidative stress to the peritoneum and results in EPS over the course of several years.10 Frequent changes in dialysate increase the risk of contracting infectious diseases.11 Resolving these would not only increase the quality of life for the patient, but also reduce medical costs worldwide. For these objectives to be met, both a decrease in oxidative stress to the peritoneal membrane and an increase in the adsorption capacity of blood wastes are required.
We have previously developed novel nanotherapeutics with redox nanoparticles (RNPs) containing nitroxide radicals as free radical scavengers for treating cerebral and renal ischemia–reperfusion, as well as brain hemorrhage.12 Typical characteristics of RNPs are (1) because nitroxide radicals are covalently conjugated to the nanoparticle backbone, they are not leaked to the outside of the nanoparticle;13 (2) the sizes of RNPs are ca. 40 nm in diameter and thus they are not internalized into healthy cells;14 and (3) as a consequence RNPs do not interfere with normal redox reactions inside the cell. These characteristics help RNPs selectively scavenge over-produced reactive oxygen species (ROS), especially outside the cell. We have so far confirmed the therapeutic effects of RNPs with respect to several disease models such as cerebral15 and renal16 ischemia–reperfusion injuries, cerebral hemorrhage,17 cancer,18 ulcerative colitis,19 and small intestinal inflammation.20
The objective of this study was to apply RNPs as one of the components in dialysate to reduce oxidative stress. Porous silica nanoparticles were combined with RNPs in order to enhance the adsorption capacity of creatinine and other LMW compounds effectively (Fig. 1). The silica-containing RNPs (siRNPs) thus prepared were confirmed to increase the adsorption capacity of uremic toxins in vivo. EPS model rats were prepared by injecting them daily with chlorhexidine gluconate (CH) intraperitoneally (i.p.) for a week. siRNPs were administered to the peritoneal cavity of the rats at the same time to confirm that they were protected against CH-induced inflammation. CH-induced dysfunction of the peritoneal membrane such as disruption of the mesothelial cell layer and vascularity of the expanded submesothelial compact zone was not observed for the siRNP treatments. These results show potential for siRNPs to be used as a new multi-functional nanomaterial in peritoneal dialysis.
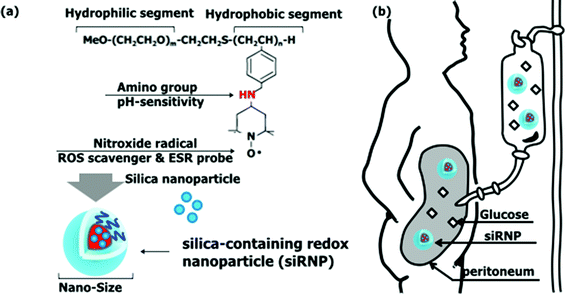 |
| Fig. 1 Schematic illustration of silica-containing redox nanoparticles (siRNPs). (a) Structure of siRNPs and (b) siRNPs for peritoneal dialysis. | |
2. Materials and methods
2.1. Materials
2,2′-Azobisisobutyronitrile (Kanto Chemical Co., Inc., Tokyo, Japan) was purified by performing recrystallization from methanol. Chloromethylstyrene (CMS) was kindly provided by Seimi Chemical Co., Ltd (Kanagawa, Japan) and purified by washing with alkaline to remove the inhibitor, dehydrating with sodium sulfate, and vacuum distilled under a nitrogen atmosphere. 4-Amino-2,2,6,6-tetramethylpiperidine-1-oxy (4-amino-TEMPO) (Aldrich Chemical Co., Inc., U.S.A.), 2-propanol, diethyl ether, dimethyl sulfoxide, N,N-dimethylformamide (DMF), and tetraethoxysilane (TEOS) (Wako Pure Chemical Industries, Ltd, Osaka, Japan) were used without further purification. Colloidal silica 10–20 nm in diameter (Snowtech-O, SiO2 = 20 wt%; pH = 2–4; viscosity = 1.0–3.0 mPa s) was kindly provided by Nissan Chemical Co., Ltd (Tokyo, Japan) and used without further purification. PEG possessing a methoxy group at the α-chain end and a sulfanyl group at the ω-chain end (MeO-PEG-SH) (NOF CORPORATION Co., Ltd, Japan), 2-methyl-6-p-methoxyphenylethynyl-imidazopyrazinone (MPEC, Atto, Corp. Tokyo, Japan), and methyl orange (MO) (Kanto Chemical, Tokyo, Japan) were used without further purification. Twenty percent chlorhexidine gluconate (CH; Wako, Tokyo, Japan) was used after dilution to 0.1% by the addition of saline.
2.2. Preparation of MeO-PEG-b-PMNT
Block copolymer (MeO-PEG-b-PMNT) composed of the hydrophilic PEG segment and the hydrophobic poly(4-methylstyrene) segment possessing nitroxide radicals as a side chain via an amine linkage was synthesized according to our previous studies.21 Briefly, poly(ethylene glycol)-b-poly(chloromethylstyrene) (MeO-PEG-b-PCMS) was synthesized by the conventional radical telomerization of CMS using MeO-PEG-SH as a telogen. The chloromethyl groups were converted to 2,2,6,6-tetramethylpiperidinyl-1-oxyls via an amination reaction of benzyl chloride in the MeO-PEG-b-PCMS block copolymer with 4-amino-TEMPO in DMF.
2.3. Preparation of siRNPs
Preparation of siRNPs was carried out by following two methods.
2.3.1. Preparation of siRNPs using commercially available colloidal silica (siRNPs(1)).
To an aqueous solution of PEG-b-PMNT under acidic conditions (pH = 3.0–4.0; 5 mg mL−1) different amounts of colloidal silica were added (1, 5, and 10 wt% against polymer weight). The mixture was stirred using a magnetic stirrer and the pH was increased to 9 by adding NaOHaq. The zeta potential and particle sizes of the siRNPs were measured by employing laser doppler velocimetry and dynamic light scattering (DLS), respectively, using a Zetasizer Nanoseries ZEN3600 (Malvern Instruments Ltd, Worcestershire, UK). Incorporation of silica into the RNPs was determined by transmission electron microscopy (TEM: JEOL JEM-100CX; acceleration voltage = 100 kV).
2.3.2. Preparation of siRNPs by a TEOS sol–gel method (siRNPs(2)).
RNP was prepared by performing dialysis, whereby 5 mg of the MeO-PEG-b-PMNT was dissolved in 1 mL of N,N-dimethylformamide (DMF; Wako Pure Chemicals, Osaka, Japan). It was then transferred into a membrane tube (Spectra/Por, molecular weight cut-off size: 3500 Da, Spectrum Laboratories Inc., Savannah, GA, USA) and then dialyzed for 24 h against 2 L of distilled water, which was changed after 2, 4, 8, 12, and 20 h. A predetermined amount of TEOS (Si/polymer ratios were in the range of 0–30 wt%) was added to the reaction mixture and it was stirred for 24 h at 80 °C under a nitrogen atmosphere. Following this, it was transferred into a membrane tube (Spectra/Por, molecular weight cut-off size: 12–14
000 Da, Spectrum Laboratories Inc., Savannah, GA, USA) and dialyzed for 48 h against 2 L of distilled water, which was changed after 1, 2, 4, 8, 20, and 32 h. The sizes, surface charge, silica content, and electron spin resonance (ESR) intensity of the obtained siRNPs were analyzed using DLS, laser Doppler viscometer, an inductively coupled plasma optical emission spectrometer (ICPS-8100 (Shimadzu, Tokyo Japan, 1.2 kW), and ESR (Burker EMXPlus, Germany, center field: 3500.00 G; sweep width: 400.000 G; MW Attenuator: 30 dB; temperature: 300.00 K; receiver gain: 1.00 × 103; modulation frequency: 100.00 kHz; modulation amplitude: 2.00 G; modulation phase: 0.00°; offset: 0.00%; time constant 20.48 ms; conversion time: 40 ms; harmonic: 1; and dual detection mode: Phase).
2.4. Animal experiments
Blood uptake tests of siRNPs and their protective effect against EPS injury were carried out using 6 weeks old male SD rats (approximately 200 g) that were purchased from Charles River Japan, Inc. (Yokohama, Japan). PD experiments using siRNPs were carried out using 6 weeks old male ICR mice (approximately 30 g) that were purchased from Charles River Japan, Inc. (Yokohama, Japan). The animals were maintained in the experimental animal facilities of the University of Tsukuba. All of the experiments were performed according to the Guide for the Care and Use of Laboratory Animals Resource Center of the University of Tsukuba.
2.5. Blood uptake of siRNPs in vivo
Three milliliters of an aqueous solution of siRNPs(1) (polymer concentration = 20 mg mL−1; 6.66 mg mL−1; 42.6 mM nitroxide radicals) or TEMPOL (6.66 mg mL−1; 42.6 mM nitroxide radicals) was administered into the peritoneal cavity of the 6 weeks old male SD rats. After predetermined time intervals, 100 μL of blood was drawn from the tip of the tail while each rat was under anesthesia induced by isoflurane (4% induction, 2% maintenance). Serum samples were obtained by carrying out centrifugation (5000 g) and the ESR intensity was analyzed after the addition of potassium ferricyanide (K3[Fe(CN)6]) (100 mM, 10 μL) to the plasma (200 μL) to re-oxidize the hydroxylamines to nitroxide radicals.
2.6. Protective effect of siRNPs against EPS injury
EPS model rats were prepared according to a prior article by Bozkurt et al.22 To 6 weeks old male SD rats, 2 mL of 0.1% CH and 15% ethanol in saline were given by i.p. administration once a day for 1 week to prepare the EPS model rats. Simultaneously, 2 mL of saline, 3 mL of siRNPs(1) (polymer concentration, 20 mg mL−1; 42.6 mM nitroxide radicals), or 3 mL of TEMPOL (6.66 mg mL−1; 42.6 mM nitroxide radicals) was given in the peritoneal cavity once a day for 1 week. After 1 week, the rats were sacrificed and the peritoneum removed. The generation of superoxide in the peritoneum was monitored by chemiluminescence using MPEC. Histological assays were carried out using the Masson trichrome (MT) staining method23 and were analyzed using an optical microscope (DM RXA2; Leica, Tokyo, Japan).
2.7. Effect of siRNPs on peritoneal dialysis
2.7.1. Adsorption profile of siRNPs in vitro.
To a 990 μL of MO solution 10 μL of sample solution was added and the solution was stirred for 1 h at r.t. (initial concentrations of MO and siRNPs(2) were 9.82 μg mL−1 and 1.7 mg mL−1 (2.8 mM nitroxide radicals), respectively). This was followed by performing centrifugal filtration of the mixture (MWCO: 300
000; 2580 g; 10 min; 25 °C). The filtrate was monitored using a UV/Vis apparatus at 466 nm (Shimadzu UV2500).
2.7.2. Decrease in blood creatinine and blood urea nitrogen by siRNPs in vivo.
Renal failure mice were prepared by ischemic treatment of both kidneys. After 6-week-old ICR mice were anesthetized with pentobarbital sodium (40 mg kg−1), both renal pedicles were bound with sutures to produce renal ischemia model mice. After the peritoneum was sewed up, 2.4 mL of 4.25 wt%/vol% glucose solution containing siRNPs(2) (100 mg mL−1) was introduced into the peritoneal cavity and blood was drawn via cardiocentesis into a tube using a heparinized syringe after predetermined time intervals. Plasma samples were obtained by performing centrifugation (2000 g), and creatinine and blood urea nitrogen (BUN) levels were analyzed using a Fuji DRI-CHEM 3500 (Fuji Film, Tokyo, Japan).
2.8. Statistical analysis
All data are expressed as the mean ± S.E.M. from 8 to 10 animals per group. Statistical analysis using SPSS (IBM Corp., NY, USA) was performed by one-way analysis of variance followed by Tukey's post-hoc test.
3. Results and discussion
3.1. Preparation of siRNPs
Preparation of siRNPs was carried out according to Scheme 1. MeO-PEG-b-PMNT was synthesized by a two-step reaction starting from MeO-PEG-SH. The molecular weight and composition of the obtained PEG-b-PMNT are described in the ESI.† The incorporation of silica into the core of the redox nanoparticles was carried out by following two methods. Commercially available silica nanoparticles are negatively charged due to the dissociation of silanols on their surface. Because the PMNT segment in PEG-b-PMNT possesses repeating amino groups as side chains, it causes electrostatic interaction with silica particles. After PEG-b-PMNT was dissolved in acidic media along with silica nanoparticles followed by increasing the pH to neutral, about half of the amino groups in the PMNT segment deprotonate to form core–shell type polymer micelles.13Fig. 2 shows DLS and TEM data. Average size of the micelles was approximately 40 nm (silica content of 5 wt%), which was slightly increased compared with empty RNPs (22 nm, silica content of 0 wt%). From the TEM analysis, silica particles were observed in the micelle core and their number increased with increasing initial ratio (silica/polymer). For example, 5 and 15 average number of silica in the core of RNPs were observed for 1 and 5 wt% of the initial silica dose, respectively. More than 5 wt% silica content tended to coagulate the particles as shown in Fig. 2.
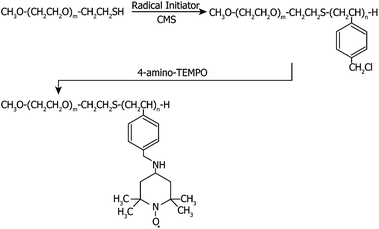 |
| Scheme 1 Synthetic scheme for nitroxide radical containing block copolymer, PEG-b-PMNT. | |
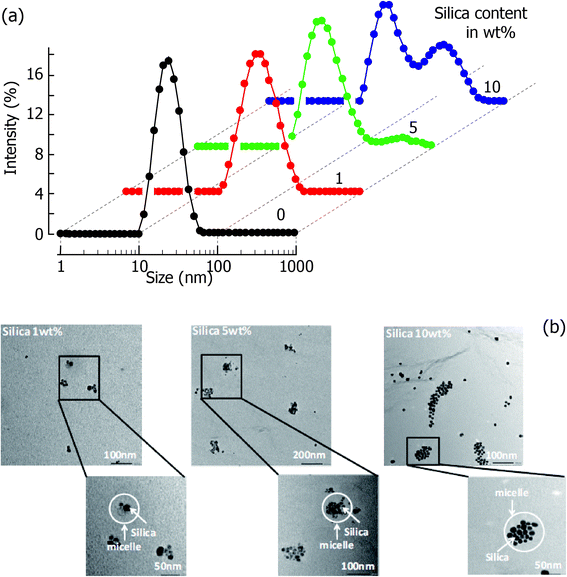 |
| Fig. 2 Size distributions of silica-containing redox nanoparticles (siRNPs)(1) prepared by different silica/PEG-b-PMNT ratios determined using dynamic light scattering (a) and transmission electron microscopy (TEM) data (b). | |
Because the core of RNPs is hydrophobic under neutral conditions, hydrophobic compounds can be solubilized. When TEOS was added to the RNP solution, the solution became completely transparent due to the solubilization of TEOS in the core of the RNPs. This was followed by a base-mediated hydrolysis–condensation reaction that took place to form silica nanoparticles in the core of RNPs, which was catalyzed by the amino groups located in it. It is interesting to note that the stable siRNPs with much higher silica content could be prepared by this sol–gel method in contrast to those that were prepared using commercially available silica (data not shown). The DLS data are shown in the ESI.† Silica contents in the nanoparticle increased in a TEOS dose dependent manner, which is summarized in Table S2.† Though the high amount of silica was entrapped in the nanoparticle, the surface charge of the obtained nanoparticle (siRNP(2)(Si/polymer = 20 wt%)) was ca. −4.03 mV, which is similar to that of siRNP(1) as shown in Table S1.† The shielding of the surface charge of siRNP(2) denotes confinement of silica in the core of the nanoparticle.
3.2. Blood uptake of siRNPs
Since the size of the prepared siRNPs in this study was ca. 40 nm, the solution was completely transparent as shown in the insert of Fig. 3. This is a better characteristic of an additive of dialysate. However, if siRNPs are absorbed into the blood via the peritoneum, adverse effects to the entire body have to be taken into consideration. After siRNPs were administered into the peritoneal cavity, the ESR signal of nitroxide radicals in the serum was monitored as a function of time. When low-molecular weight TEMPOL was administered, a typical triplet signal based on nitroxide radicals was observed at 10 min after administration and observed until 30 min as shown in Fig. 3. In contrast, no ESR signal was observed in the blood stream in the case of siRNPs(1) and siRNP(2). It was reported that the threshold of the peritoneum is ca. 30 kDa.24 Thus, a 40 nm size prevents penetration through the peritoneum membrane. Considering these results, specifically the transparent nanoparticle solution preventing blood-stream adsorption, it can be concluded that siRNPs are highly safe as an additive of peritoneal dialysate.
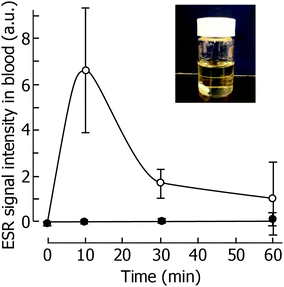 |
| Fig. 3 Blood uptake of TEMPOL (open circles) and silica-containing redox nanoparticles (siRNPs)(1) (closed circles, SiO2 = 5 wt%) as a function of time. The samples were administered into the peritoneal cavity (3 mL of siRNPs) at 6.66 mg mL−1 or TEMPOL (6.66 mg mL−1). The concentration of the nitroxide radicals was 42.6 mM for both cases. The insert is a photo of the siRNP(1) solution (20 mg mL−1). | |
3.3. Protective effect of siRNPs against EPS injury
We have so far confirmed that our RNPs effectively suppress oxidative stress by scavenging ROS, which shows their remarkable therapeutic effects against several diseases as stated above. If the siRNPs prepared in this study also work effectively as ROS scavengers, they may prevent EPS because the oxidative stress is believed to be an inducer of EPS.25 EPS model rats were prepared by administering CH. As can be seen in Fig. 4, following the administration of CH into the peritoneal cavity for 1 week, the ROS level significantly increased. When siRNPs(1) were administered, the ROS level was the same as that of the control. The administration of low molecular weight TEMPOL also decreased the ROS level but not to the same level as that of siRNPs. Rapid adsorption of TEMPOL into the blood stream (which may cause adverse effects across the entire body) might decrease the effective concentration in the peritoneal cavity.
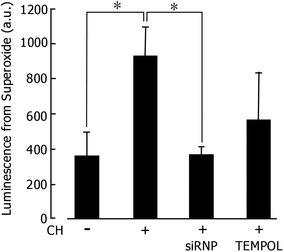 |
| Fig. 4 Amount of superoxide anion in the peritoneum due to treatment via PD. (20 mg mL−1 of silica-containing redox nanoparticles (siRNPs) or 6.66 mg mL−1 of TEMPOL was added to the dialysate.). | |
Fig. 5 shows the results of MT staining after the treatments. The CH-treated rats showed dysfunction of the peritoneum such as disruption of the mesothelial cell layer and vascularity of the expanded submesothelial compact zone (Fig. 5b), while the normal rats did not exhibit these symptoms (Fig. 5a). For example, the thickness became 269 ± 48 μm when CH was intraperitoneally administered, while for the normal rats it was only 29 ± 13 μm. When siRNPs were administered, the thickness was 42 ± 18 μm, which indicates its extremely strong therapeutic effect (Fig. 5c). We again observed a limited effect for low molecular weight TEMPOL (thickness was 87.8 ± 34.1 μm) as shown in Fig. 5d.
 |
| Fig. 5 Histological assessments by performing Masson trichrome (MT) staining of peritoneum treated with saline (2 mL) (a), chlorhexidine gluconate (CH) (2 mL at 0.1%) (b), CH + silica-containing redox nanoparticles (siRNP(1), SiO2 = 5 wt%) (CH: 2 mL at 0.1%; siRNP(1): 3 mL of 20 mg mL−1 solution) (c) and CH + TEMPOL (CH: 2 mL at 0.1%; and TEMPOL: 3 mL of 6.66 mg mL−1 solution) (d). These solutions were administered into the peritoneal cavity once a day for 1 week. The arrows indicate the thickness of the peritoneum. Scale bars = 200 μm. | |
3.4. Effect of siRNPs on peritoneal dialysis
3.4.1. Adsorption profile of siRNPs in vitro.
In vitro adsorption capacity of siRNPs was evaluated using MO. Because RNPs possess a hydrophobic core, the maximum adsorption of MO was 6.4 wt%. As can be seen in Fig. 6, the adsorption capacity increased with the increasing amount of silica and attained 9.2 wt% for siRNPs(2) (silica content 2.3%). siRNPs thus increased 50% in the adsorption capacity compared with that of RNPs in vitro.
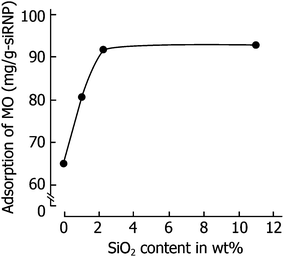 |
| Fig. 6 Adsorption capacity of silica-containing redox nanoparticles (siRNPs)(2) in vitro. The horizontal axis denotes the silica content of the siRNPs(2). The initial concentrations of methyl orange (MO) and siRNPs(2) were 9.82 μg mL−1 and 1.7 mg mL−1, respectively. | |
3.4.2. Decrease in blood creatinine and BUN by siRNPs in vivo.
Since siRNPs(2) showed high adsorption of low molecular weight compounds, they were anticipated to improve the therapeutic effects in PD when mixed in the dialysate. After siRNPs-containing dialysate was administered to the peritoneal cavities of acute renal failure model mice, blood levels of creatinine and BUN were monitored (Fig. 7). Both the creatinine and BUN levels significantly increased for the renal failure model mice after 6 and 9 h, respectively. A 4.25 vol%/wt% glucose solution decreased these levels to some extent for the complete bilateral renal ischemia. When RNPs or siRNPs(2) were mixed with the dialysate, the blood levels of creatinine and BUN decreased significantly. In particular, BUN after 6 h and creatinine after 9 h showed a strong effect for siRNPs(2). The increased adsorption capacity of siRNPs(2) worked effectively in this case.
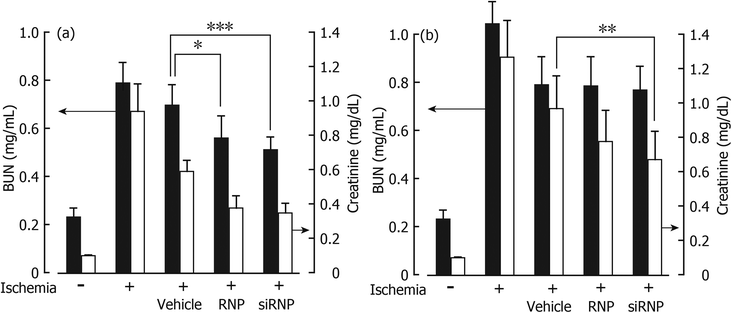 |
| Fig. 7 Therapeutic effect of silica-containing redox nanoparticles (siRNPs(2)) as an additive of peritoneal dialysis (PD)–dialysate against renal failure model mice. Vehicle: 2.4 mL of 4.25 wt%/vol% glucose solution; RNP: 2.4 mL of 4.25 wt%/vol% glucose solution containing RNPs (100 mg mL−1) and siRNPs (2, SiO2 = 3.77 wt%): 2.4 mL of 4.25 wt%/vol% glucose solution containing siRNPs(2) (2.4 m of 100 mg mL−1) for 6 h (a) and 9 h (b). *p < 0.05; **p < 0.01; ***p < 0.005. | |
4. Conclusions
We have improved the peritoneal dialysis system using a newly designed siRNP. Silica particles 10–20 nm in diameter were incorporated into RNPs in order to improve the colloidal dispersion stability of silica in the physiological environment and the adsorption capacity of low molecular weight compounds present as waste in blood. When siRNPs were administered into the peritoneal cavity, no blood adsorption was observed, which was in sharp contrast to a low molecular weight antioxidant. Negligible or no blood uptake of siRNPs would likely not be detrimental to the entire body. The ROS scavenging characteristic of the siRNPs prevented oxidative damage of the peritoneum in EPS model rats. The addition of siRNPs to dialysate also improved the therapeutic efficiency of acute renal failure model mice. These results suggest that siRNPs are suitable nanobiomaterials for patient friendly peritoneal dialysis.
Acknowledgements
This work was partially supported by a Grant-in-Aid for Scientific Research on Innovative Areas “Fusion Materials” (#25107707) from the Ministry of Education, Culture, Sports, Science and Technology of Japan (MEXT), a Grant-in-Aid for Scientific Research(C) from the JSPS (#24591224), and a grant from the Japanese Association of Dialysis Physicians (JADP2012-09).
References
- A. Grassmann, S. Gioberge, S. Moeller and G. Brown, ESRD patients in 2004: global overview of patient numbers, treatment modalities and associated trends, Nephrol. Dial. Transplant., 2005, 27, 2587–2593 CrossRef PubMed
.
- F. Locatelli, A. Cavalli and B. Tucci, The growing problem of intradialytic hypertension, Nat. Rev. Nephrol., 2010, 6, 41–48 CrossRef PubMed
.
- M. K. Shamseddin and P. S. Parfrey, Sudden cardiac death in chronic kidney disease: epidemiology and prevention, Nat. Rev. Nephrol, 2011, 7, 145–154 CrossRef PubMed
.
- K. Sennfält, M. Magnusson and P. Carlsson, Comparison of hemodialysis and peritoneal dialysis–a cost-utility analysis, Perit. Dial. Int., 2002, 22, 39–47 Search PubMed
.
- S. P. MacDonald, M. R. Marshall, D. W. Johnson and K. R. Polkinghorne, Relationship between Dialysis Modality and Mortality, J. Am. Soc. Nephrol., 2009, 20, 155–163 CrossRef PubMed
.
- T. C. Lin, M. T. Kao, M. N. Lai and C. C. Huang, Mortality difference by dialysis modality among new ESRD patients with and without diabetes mellitus, Dial. Trasplant., 2006, 35, 234 CrossRef
.
- D. W. Johnson, Y. Cho, B. E. R. Livingston, C. M. Hawley, S. P. McDonald and F. G. Brown,
et al., Encapsulating peritoneal sclerosis: incidence, predictors, and outcomes, Kidney Int., 2010, 77, 904–912 CrossRef PubMed
.
- H. Kawanishi, Encapsulating peritoneal sclerosis, Nephrology, 2005, 10, 249–255 CrossRef PubMed
.
- M. C. Brown, K. Simpson, J. J. Kerssens and R. A. Mactier, Encapsulating Peritoneal Sclerosis in the New Millennium, A National Cohort Study, Clin. J. Am. Soc. Nephrol., 2009, 4, 1222–1229 CrossRef PubMed
.
- M. A. Glomb and V. M. Monnier, Mechanism of Protein Modification by Glyoxal and Glycolaldehyde, Reactive Intermediates of the Maillard Reaction, J. Biol. Chem., 1995, 270, 10017–10026 CrossRef CAS PubMed
.
- G. Abraham, E. Savin, A. Ayiomamitis, S. Izatt, S. I. Vas and R. E. Mathews,
et al., Natural history of exit-site infection (ESI) in patients on continuous ambulatory peritoneal dialysis (CAPD), Perit. Dial. Int., 1988, 8, 211–216 Search PubMed
.
- Y. Nagasaki, Nitroxide radicals and nanoparticles: a partnership for nanomedicine radical delivery, Therapeut. Deliv., 2012, 3, 165–179 CrossRef CAS
.
- T. Yoshitomi, R. Suzuki, T. Mamiya, H. Matsui, A. Hirayama and Y. Nagasaki, pH-Sensitive Radical-Containing-Nanoparticle (RNP) for the L-Band-EPR Imaging of Low pH Circumstances, Bioconjugate Chem., 2009, 20, 1792–1798 CrossRef CAS PubMed
.
- M. Shimizu, T. Yoshitomi and Y. Nagasaki, J. Clin. Biochem. Nutr. Search PubMed
, in press.
- A. Marushima, K. Suzuki, Y. Nagasaki, T. Yoshitomi, K. Toh and H. Tsurushima,
et al., Newly Synthesized Radical-containing Nanoparticles (RNP) Enhance Neuroprotection after Cerebral Ischemia-reperfusion Injury, Neurosurgery, 2011, 68, 1418–1426 Search PubMed
.
- T. Yoshitomi, A. Hirayama and Y. Nagasaki, The ROS scavenging and renal protective effects of pH-responsive nitroxide radical-containing nanoparticles, Biomaterials, 2011, 32, 8021–8028 CrossRef CAS PubMed
.
- P. Chonpathompikunlert, C. H. Fan, Y. Ozaki, T. Yoshitomi, C. K. Yeh and Y. Nagasaki, Redox nanoparticle treatment protects against neurological deficit in focused ultrasound-induced intracerebral hemorrhage, Nanomedicine, 2012, 7, 1029–1043 CrossRef CAS PubMed
.
- T. Yoshitomi, Y. Ozaki, S. Thangavel and Y. Nagasaki, Redox nanoparticle therapeutics to cancer — increase in therapeutic effect of doxorubicin, suppressing its adverse effect, J. Controlled Release, 2013, 172, 137–143 CrossRef CAS PubMed
.
- L. B. Vong, T. Tomita, T. Yoshitomi, H. Matsui and Y. Nagasaki, An Orally Administered Redox Nanoparticle That Accumulates in the Colonic Mucosa and Reduces Colitis in Mice, Gastroenterology, 2012, 143, 1027–1036 CrossRef CAS PubMed
.
- S. Sha, L. B. Vong, P. Chonpathompikunlert, T. Yoshitomi, H. Matsui and Y. Nagasaki, Suppression of NSAID-induced small intestinal inflammation by orally administered redox nanoparticles, Biomaterials, 2013, 34, 8393–8400 CrossRef CAS PubMed
.
- T. Yoshitomi, D. Miyamoto and Y. Nagasaki, Design of Core–Shell-Type Nanoparticles Carrying Stable Radicals in the Core, Biomacromolecules, 2009, 10, 596–601 CrossRef CAS PubMed
.
- D. Bozkurt, E. Hur, B. Ulkuden, M. Sezak, H. Nar and O. Purclutepe,
et al., Can N-acetylcysteine preserve peritoneal function and morphology in encapsulating peritoneal sclerosis, Perit. Dial. Int., 2009, 29, S202–S205 CAS
.
- C. M. Hoff, Experimental animal models of encapsulating peritoneal sclerosis, Perit. Dial. Int., 2005, 25, S57–S66 Search PubMed
.
- B. Rippe, D. Venturoli, O. Simonsen and J. de Arteaga, Fluid and electrolyte transport across the peritoneal membrane during CAPD according to the three-pore model, Perit. Dial. Int., 2004, 24, 10–27 CAS
.
- H. Terawaki, Y. Hayashi, W. J. Zhu, Y. Matsuyama, T. Terada and S. Kabayama,
et al., Transperitoneal administration of dissolved hydrogen for peritoneal dialysis patients: a novel approach to suppress oxidative stress in the peritoneal cavity, Med. Gas. Res., 2013, 3, 14 CrossRef PubMed
.
Footnote |
† Electronic supplementary information (ESI) available. See DOI: 10.1039/c3bm60236b |
|
This journal is © The Royal Society of Chemistry 2014 |