DOI:
10.1039/C3BM60188A
(Paper)
Biomater. Sci., 2014,
2, 233-241
The osteoblast and osteoclast responses to phosphonic acid containing poly(ε-caprolactone) electrospun scaffolds
Received
5th August 2013
, Accepted 24th September 2013
First published on 10th October 2013
Abstract
We describe a novel polymer containing phosphonic acid moieties which has been shown to increase the osteoblast response and decrease osteoclast activity. A 3D scaffold comprising of poly(ε-caprolactone) (PCL) functionalised with poly(vinyl phosphonic acid-co-acrylic acid) (PVPA-AA) was fabricated using electrospinning. PVPA-AA is hypothesised to mimic the action of bisphosphonates, a group of drugs used to treat osteoporosis, and likely to affect bone turnover by increasing the activity of osteoblasts and reducing osteoclast activity. The expression of collagen type I, osteocalcin and alkaline phosphatase has been found to be significantly higher (p ≤ 0.001) on PCL/PVPA-AA scaffolds when compared to PCL and tissue culture plastic (TCP). In addition, after 21 days there was a significant increase (p ≤ 0.001) in mineralisation on PCL/PVPA-AA substrates. The PCL/PVPA-AA scaffold has been reported to significantly (p ≤ 0.001) decrease osteoclast viability, with comparable results to Alendronate, a commercially available bisphosphonate. For the first time, we describe a novel active synthetic bone graft substitute, which has been shown to increase osteoblast proliferation and matrix deposition as well as reducing the number of osteoclast cells by locally induced apoptosis. The combined affects of the novel PVPA-AA polymer on osteoblasts and osteoclasts may lead to active bone repair and healing.
1. Introduction
Repair of bone defects in conditions such as osteoporosis and Paget's disease are very challenging and complex surgical intervention is often required.1 There is often poor healing and the risk of fracture is greatly increased. The low bone stock can also make revision surgery difficult. There is a clinical need for a synthetic bone graft substitute that could be used at sites of surgical intervention to promote bone formation by enhancing formation or reducing resorption, or both acting simultaneously.
A novel polymer, poly(vinyl phosphonic acid-co-acrylic acid) (PVPA-AA) has been identified to mimic the action of bisphosphonates, a group of drugs used to treat osteoporosis.2–5 Bisphosphonates are hypothesised to inhibit bone resorption by binding to the mineral surfaces of bone where they are internalised by resorbing osteoclasts.6 Bisphosphonates are structurally similar to pyrophosphate, therefore it is likely that once internalised, bisphosphonates interfere with biological processes that involve pyrophosphate-containing compounds.7–9 Bisphosphonates are characterised into two groups; nitrogen containing and non-nitrogen containing. PVPA-AA is likely to mimic the action of bisphosphonate Alendronate and non-nitrogen containing bisphosphonates such as Clodronate and Etidronate.
Osteoclast cells are most likely to internalise bisphosphonates as opposed to other cell types. This is due to bisphosphonates binding to bone mineral, which is then degraded under the action of proteolytic enzymes.10 Osteoclast cells attach to bone mineral and are therefore most likely to be exposed to the highest concentration of free, non-mineral bound bisphosphonate drug.11 The mechanism by which bisphosphonates are internalised is hypothesised to be endocytosis; osteoclasts are highly endocytic cells. In vivo studies have demonstrated the uptake of bisphosphonates by osteoclasts into intracellular vacuoles and other subcellular compartments.6,10
Once internalised, non-nitrogen containing bisphosphonates are metabolically incorporated into nonhydrolysable methylene-containing (AppCp type) analogues of ATP.12–14 These resulting metabolites contain a P-C-P moiety instead of the β, ϒ-phosphate groups of ATP.15,16 The AppCp metabolites accumulate in high concentrations in the cytosol of osteoclasts and other cell types that can effectively internalise bisphosphonates.17 It has been suggested that accumulation of these metabolites intracellularly within the osteoclast cell suppresses osteoclast function and can lead to apoptosis.18 The exact mechanism by which AppCp analogues disrupt cell metabolism is still unclear however it is suggested that the action of ATP-dependent enzymes such as adenine nucleotide translocase is inhibited which leads to loss of cell function and eventual cell death.19,20
Indeed, a number of recent studies have shown that bisphosphonates have stimulatory effects on osteoblast cells in vitro.21,22 A study comparing two bisphosphonates, alendronate and risendronate, demonstrated both bisphosphonates stimulated cell proliferation in a dose dependent manner. Cell proliferation peaked at a drug concentration of 10−8 M, however increased doses of the drug (>10−9 M), led to a decrease in osteoblast proliferation.23 Although there is a decrease in cell proliferation, there is an increase in total cellular protein, alkaline phosphatase activity and collagen type I secretion. This suggests that bisphosphonates induce maturation of osteoblast cells from a proliferating stage to a mature matrix depositing cell.24
We propose the structure of PVPA-AA mimics the action of bisphosphonates due to the close proximity of the P–C bonds and the strong negative charge surrounding the molecule chelates calcium ions. Osteoclasts are then able to internalise the polymer which then leads to disruption of cellular activity and eventual osteoclast apoptosis.
In the present study, poly(ε-caprolactone) (PCL) electrospun fibres have been functionalised with PVPA-AA to form a PCL/PVPA-AA scaffold. PCL has been extensively electrospun for tissue engineering applications, however its use is limited to non-load bearing applications due to the fragile nature of electrospun fibres. Previously we have demonstrated that the 2D fibrous mat can be moulded into a 3D spherical structure which has suitably high compressive strength to be used in load bearing applications.25 This is primarily due to PVPA-AA stabilising the 3D PCL structure. Additionally, we have demonstrated that PCL/PVPA-AA scaffolds are capable of stimulating mineralised tissue formation in critical size defects in an ex vivo model.26 A series of in vitro experiments will be used to quantify the response of osteoblasts and osteoclasts to the scaffolds in terms of viability, protein expression and mineralisation.
2. Materials and methods
2.1 Materials
A 10 w/v% polymer solution was prepared by dissolving poly(ε-caprolactone) (PCL) pellets (Mn ≈ 80
000) (Sigma Aldrich, UK), in acetone at 50 °C under continuous stirring on a hot plate to assist dissolving.
2.2 Fabrication of PCL fibres
The fabrication of PCL/PVPA-AA scaffolds is described and characterised elsewhere in detail.25,27 Briefly, the PCL polymer solution was placed in a 10 ml plastic syringe (BD Medical, UK) fitted to a 21 gauge blunted needle (BD medical, UK). A syringe pump (World Precision Instruments Ltd, UK) was used to feed the polymer solution through the needle at a constant flow rate (0.05 ml min−1) using a needle to collector distance of 15 cm. A high voltage power supply (Glassman High Voltage Inc., UK) was attached to the needle and fibres were collected onto aluminium foil. Films of PCL/PVPA-AA were prepared by immersing the electrospun PCL mat in a reservoir of 15 wt% PVPA-AA in deionised water for 24 hours.2 The films were air dried for 48 hours and then heated treated at 55 °C for 24 hours, the structure and properties of the scaffolds has been previously reported.25–27
2.3 Osteoblast culture
Human osteoblast cells (HOBs) (The European Collection of Cell Cultures, UK) (Cell line no. 406-05a) were cultured in Dulbecco's modified Eagles medium (DMEM) (Gibco®, Paisley) supplemented with 10% FBS, antibiotics (100 U ml−1 penicillin, 100 mg ml−1 streptomycin) and 50 μM ascorbic acid. Culture medium was replenished every 2 days. Scaffolds (1.2 cm × 1.2 cm) were immobilised in 24 well plates using CellCrown inserts (Scaffdex, Finland) and sterilised using a series of increasing ethanol concentrations. Cells were counted and seeded onto scaffolds at a density of 40
000 cells per cm2. Plates were cultured under standard conditions, at 37 °C with 95% humidity and 5% CO2 for up to 28 days.
2.3.1 Evaluation of osteoblast viability.
2.3.1.1 Metabolic activity.
Metabolic activity was determined using the AlamarBlue® assay. Culture medium was removed and samples were washed with sterile phosphate buffered saline (PBS), 1 ml of fresh culture medium was added along with 100 μl of alamarBlue® solution (5 mg resazurin salt/40 ml PBS). The plates were incubated at 37 °C with 95% humidity and 5% CO2 for 2 hours and 30 minutes. The fluorescence was measured at an excitation of 530 nm and emission at 590 nm using the Fluostar Optima Fluorescence Microplate Reader (BMG Labtech, Germany). To determine the concentration of PVPA-AA that yielded the most positive osteoblast response, cells were cultured on various scaffolds containing different amounts of PVPA-AA and the metabolic activity was determined as described above.
2.3.1.2 Live/Dead® staining.
Live/Dead® staining solution (Invitrogen, UK) was prepared by adding ethidium homodimer-1 (2 μl ml−1) and Calcein-AM (0.5 μl ml−1) to sterile PBS. Culture medium was removed from the samples and the wells were washed with sterile PBS. The prepared staining solution was added to each well and incubated for 15 minutes at room temperature. The scaffolds were mounted onto glass microscope slides and viewed under the Nikon Eclipse 50i fluorescence microscope with a camera attachment.
2.3.1.3 Osteoblast cell number.
Culture medium was removed and samples were washed with sterile PBS and 1 ml of dH2O was added to each well. Samples were freeze-thawed three times. The Hoechst 33258 stain solution was prepared as outlined by the manufacturer (Sigma Aldrich, UK). 50 μl of each sample and 50 μl of TNE buffer were then aliquoted to a flat-bottomed 96 well plate (Nunc, UK) along with 100 μl of Hoechst staining solution. The fluorescence was measured at an excitation of 355 nm and emission at 460 nm using the Fluostar Optima Fluorescence Microplate Reader (BMG Labtech, Germany).
2.3.1.4 Alkaline phosphatase quantification.
The samples used to determine cell number were used to calculate alkaline phosphatase activity. 20 μl of the sample solution was transferred to a flat-bottomed 96 well plate (Nunc, UK). The alkaline phosphatase substrate was prepared as described by the manufacturer; 200 μl of the substrate was added to each well. The absorbance was measured using the Labsystems Multiskan Ascent plate reader (Thermo Scientific, UK), every 30 seconds for 10 minutes.
2.3.1.5 Quantification of collagen secretion.
The matrix bound collagen expressed during the culture period was determined using the Sircol Collagen Assay (Biocolor, Ireland). The collagen concentration was determined by following the manufacturers instructions. The absorbance was measured using the Labsystems Multiskan Ascent plate reader (Thermo Scientific, UK) with the wavelength set to 540 nm.
2.3.1.6 Quantification of osteocalcin expression.
At specific time points, media was collected from the cultures and the osteocalcin was quantified using the human Osteocalcin ELISA kit (Invitrogen, UK). All components of the kit were used at room temperature. The osteocalcin concentration was determined by following the manufacturer's instructions.
2.3.1.7 Quantification of osteoprotegerin expression.
The osteoprotegerin (OPG) ELISA (Biomedica, Germany) was carried out to determine the effect of the scaffold on OPG expression. The osteoprotegerin concentration was determined by following the manufacturers instructions. The absorbance was measured at 450 nm with a reference filter of 630 nm using the Labsystems Multiskan Ascent plate reader (Thermo Scientific, UK).
2.3.1.8 Evaluation of the mineralisation.
To study mineralisation, osteogenic media consisting of Dulbecco's modified Eagles medium (DMEM) supplemented with 10% FBS, antibiotics (100 U ml−1 penicillin, 100 mg ml−1 streptomycin), 100 nM dexamethasone, 50 μM ascorbic acid and 10 mM β-glycerophosphate (Merck Biosciences, UK) was used. At day 7, 14, 21 and 28 culture medium was removed and cells were washed with sterile PBS and fixed in ice cold 70% ethanol for 1 hour. Cells were washed with distilled water and stained at room temperature for 10 minutes using a 40 mM alizarin red solution. The pH of the stain solution was adjusted to 4.2. For optical density measurements, 10% cetylpyridinium chloride was dissolved in 10 mM sodium phosphate. The 10% cetylpyridinium chloride solution was added (1 ml) to each well and incubated at room temperature for 30 minutes under continuous shaking. 200 μl of the solution was transferred to a clear, flat-bottomed 96 well plate (Nunc, UK) and the absorbance was measured at 562 nm using the Labsystems Multiskan Ascent plate reader (Thermo Scientific, UK).
2.4 Osteoclast culture
Human osteoclast precursor cells (Lonza®, UK) were dispersed in osteoclast precursor basal medium (α-MEM) supplemented with 10% FBS, 66 ng ml−1 RANK-L, 33 ng ml−1 M-CSF, antibiotics (100 U ml−1 penicillin, 100 μg ml−1 streptomycin) and 2 mM L-glutamine (All supplied by Lonza, UK). Cells were counted and seeded onto scaffolds and glass cover slips (Agar Scientific, UK) at a density of 60
000 cells per cm2, unless stated otherwise. Plates were cultured under standard conditions, at 37 °C with 95% humidity and 5% CO2 for up to 14 days and the osteoclast phenotype was verified using tartrate resistant acid phosphatase staining.
2.4.1 Evaluation of osteoclast viability.
2.4.1.1 Cell number.
Osteoclast cells were cultured on substrates for 14 days and medium was replenished every third day. A culture period of 14 days was used due to the low in vitro culture life of osteoclast cells. To compare the effect of the PVPA-AA on cell number with bisphosphonates, cells were cultured in medium conditioned with two concentrations of Alendronate (10 μmol and 100 μmol, referred to as AL-10 and AL-100 respectively, hereafter). At day 14, all cells were washed with PBS. Two millilitres of 0.02% EDTA was added to each well and plates were incubated for 20 minutes at room temperature. The EDTA was aspirated from each well and two millilitres of PBS was added to each well. A cell scraper was used to gently remove cells from the substrate. Cells from each well were counted by mounting 50 μl of cell suspension into an improved neubauer heamocytometer and viewed under the Leica DM-IL light microscope. The total cell number for each well was calculated.
2.4.1.2 Detection of apoptosis.
The APOPercentage apoptosis kit (Biocolor Ltd, Ireland) was used to quantify the number of apoptotic cells present on the substrates. The APOPercentage dye is actively transported into the cells that lose their membrane asymmetry, which occurs early during apoptosis. The dye was diluted (1
:
20) in culture medium, 50 μl of the staining solution was added to each well and the plates were incubated for 30 minutes under standard conditions. The dye was aspirated and samples were washed with PBS. The accumulation of the dye within the cells results in the cells developing a red/pink colour. 200 μl of APOPercentage dye releasing reagent was added to each well to release the dye. Plates were shaken for 30 minutes and the absorbance was read at 550 nm using the Labsystems Multiskan Ascent plate reader (Thermo Scientific, UK).
2.5 Statistical analysis
Statistical evaluation of data was performed using GraphPad TM software package. Tests were carried out in triplicate (n = 3) and all data is reported as mean ± standard deviation (SD) at a significance level of p ≤ 0.05. Two-way ANOVA with repeated measures was carried out to compare the groups of the osteoblast culture whereas a one-way ANOVA with the Bonferroni test was carried out to compare the groups of the osteoclast culture.
3. Results
3.1 Osteoblast proliferation and metabolic activity
The response of human osteoblast cells was monitored on difference concentrations of PVPA-AA in the PCL/PVPA-AA scaffolds (Fig. 1a). The Alamarblue assay was used to determine cell metabolic activity over 21 days on five difference PVPA-AA concentrations (0 wt%, 7.5 wt%, 15 wt%, 22.5 wt% and 30 wt% PVPA-AA). At day 1, there was no significant difference (p > 0.05) in metabolic between the five different concentrations. However at day 7, the metabolic activity of cells cultured on the 15 wt% PCL/PVPA-AA scaffold was significantly higher (p ≤ 0.001) than on 0 wt% and 30 wt% PCL/PVPA-AA scaffolds. From day 14, the metabolic activity of cells cultured on 15 wt% PCL/PVPA-AA scaffolds was significantly higher that the other scaffold types. The results demonstrates that the PVPA-AA polymer effects osteoblast activity on a dose dependant manner, with an optimum PVPA-AA concentration of 15 wt% therefore a 15 wt% PVPA-AA concentration was used for the remainder of the in vitro cell culture studies.
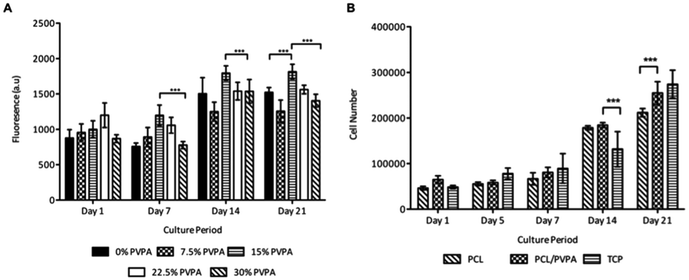 |
| Fig. 1 (a) Osteoblast metabolic activity on varying concentrations of PVPA-AA over 21 days. (b) Osteoblast proliferation over 21 days on PCL/PVPA-AA, PCL and TCP substrates. Mean ± sd, n = 9 triplicates (*p ≤ 0.05, **p ≤ 0.01, ***p ≤ 0.001). | |
Osteoblast cell number on 15 wt% PCL/PVPA-AA scaffolds was monitored over 21 days (Fig. 1b). There was no significant difference (p > 0.05) in cell number between scaffold types from day 1 to day 7. From day 14, osteoblast number was significantly higher (p ≤ 0.001) on PCL/PVPA-AA and PCL scaffolds in comparison with TCP substrates. However by day 21, osteoblast cell number was higher on PCL/PVPA-AA scaffolds compared to PCL scaffolds. However there was no significant difference (p > 0.05) between PCL/PVPA-AA and TCP substrates. The variations in cell number were also observed during Live/Dead staining of cells in the initial 48 hours after cells seeding (Fig. 2). A greater number of dead cells (red) were observed on PCL scaffolds, less so on PCL/PVPA-AA scaffolds and even less so on TCP substrates. The higher number of dead cells present on PCL scaffolds could be owed to the hydrophobic nature of the PCL substrate.
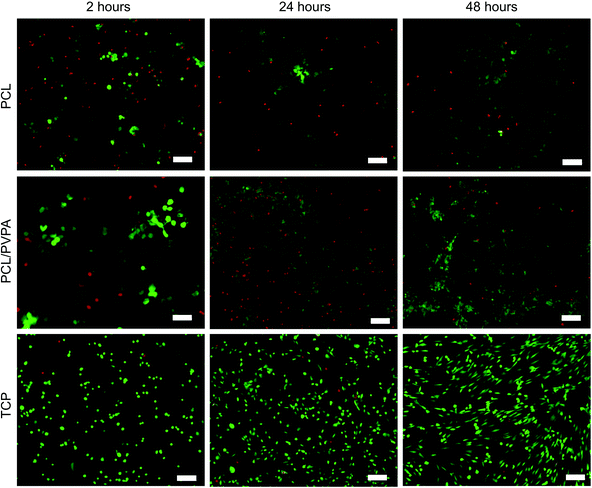 |
| Fig. 2 Live/Dead™ imaging of human osteoblast cells to illustrate cell viability on PCL/PVPA-AA, PCL and TCP substrates over 48 hours. Live cells stained green, dead cells stained red. n = 9, scale bar 20 μm. | |
3.2 Protein and enzyme expression
Collagen expression, alkaline phosphatase activity, osteocalcin expression and osteoprotegerin expression was monitored over 21 days on PCL/PVPA-AA scaffolds (Fig. 3). All protein expression increased over the culture period. From day 14 onwards, collagen expression was significantly higher (p ≤ 0.001) on PCL/PVPA-AA scaffolds compared to PCL and TCP substrates (Fig. 3a). Collagen expression plateaued from day 14; there was no significant difference (p > 0.05) between day 14 and day 21.
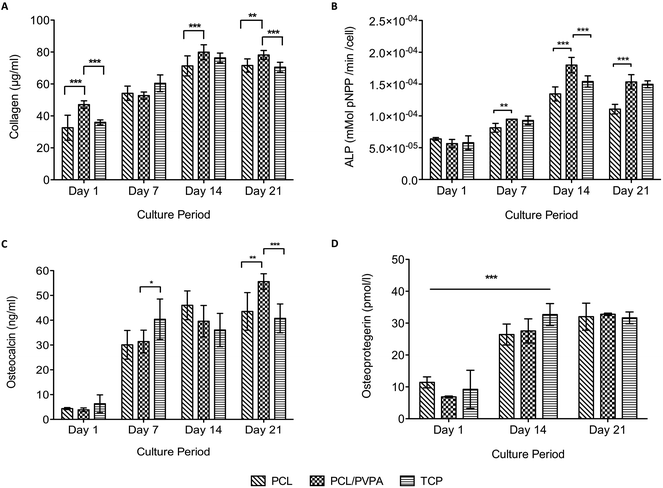 |
| Fig. 3 Quantification of protein and enzyme expression by human osteoblast cells on PCL/PVPA-AA, PCL and TCP substrates over 21 days. (a) Collagen expression, (b) alkaline phosphatase activity, (c) osteocalcin expression and (d) osteoprotegerin expression. Mean ± sd, n = 9 triplicates (*p ≤ 0.05, **p ≤ 0.01, ***p ≤ 0.001). | |
The initiation of mineralisation requires a critical concentration of both calcium and phosphorous. Phosphorous is generated through the action of the enzyme, alkaline phosphatase. The current study shows that alkaline phosphatase activity peaked at day 14 on all substrate types (Fig. 3b). At day 14, alkaline phosphatase activity was significantly higher (p ≤ 0.001) on PCL/PVPA-AA scaffolds compared to PCL and TCP substrates. At day 21, alkaline phosphatase activity decreased, however activity was still significantly higher (p ≤ 0.001) on PCL/PVPA-AA scaffolds compared to PCL scaffolds. The decrease in activity from day 14 to day 21 suggests that the previously deposited calcium and phosphorous is being mineralised.
Osteocalcin was expressed by human osteoblast cells in the current study. From day 1 to day 7, there was a significant increase (p ≤ 0.001) in osteocalcin expression. At day 7 and day 14, there was a significant difference in its expression between scaffold types. At day 21, osteocalcin expression was the greatest on PCL/PVPA-AA scaffolds.
Osteoprotegerin is a decoy protein secreted by osteoblasts and their precursor cells to prevent osteoclast formation. We report a significant increase in osteoprotegerin expression from day 1 to day 14 on all scaffold types. Osteoprotegerin expression did not change for the remainder of the study. There was a significant difference in osteoprotegerin expression between scaffold types throughout the study, suggesting that the PVPA-AA does not affect osteoprotegerin activity.
3.3 Mineralisation
Mineralisation is essential for healthy bone formation, and can be assessed in vitro using alizarin red staining. Staining of the scaffolds over 28 days revealed that calcium was deposited on all scaffold types, and from day 14 was significantly higher (p ≤ 0.001) on PCL/PVPA-AA scaffolds (Fig. 4a).
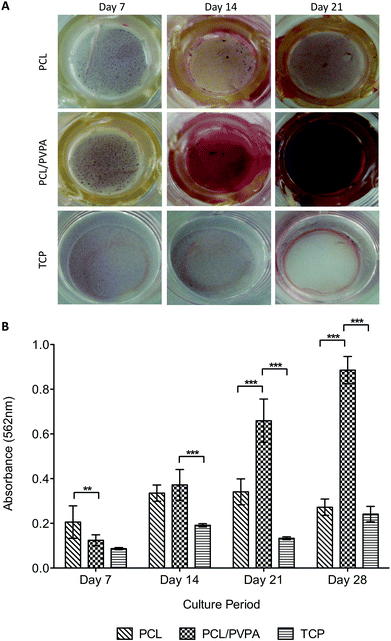 |
| Fig. 4 (a) Photographs illustrating the deposition of calcium on PCL/PVPA-AA, PCL and TCP substrates over 28 days. The red stain denotes the presence of calcium. (b) Quantification of calcium deposition by human osteoblasts on the test materials over 28 days. Mean ± sd, n = 9 triplicates (*p ≤ 0.05, **p ≤ 0.01, ***p ≤ 0.001). | |
At day 21, an intense red stain was on PCL/PVPA-AA scaffolds was visualised. The intensity of the stain indicates the presence of calcium and consequently calcium phosphate formation.
3.4 Osteoclast cell number
Osteoclast precursor cells were differentiated in to mature osteoclast cell with the addition of RANK-L and M-CSF.28,29 The osteoclast phenotype was verified using TRAP staining (Bassi et al., 2011a). Osteoclast viability was determined after 14 days on the different scaffold types (Fig. 5a). Cultures containing two concentrations (10 μmol and 100 μmol, referred to as AL-10 and AL-100, respectively) of Alendronate, a commercially available bisphosphonate, were used in the study. Results show that osteoclast viability was lowest on PCL/PVPA-AA, AL-10 and AL-100 substrates (p ≤ 0.001). Osteoclast viability of PCL/PVPA-AA substrates was comparable to that in AL-10 cultures, which is the concentration that mimics the in vivo physiological concentration. This would suggest that the PVPA-AA polymer can effect osteoclasts in a similar way to bisphosphonates. The reduction in osteoclast number was verified using an apoptosis assay (Fig. 5b). Osteoclast apoptosis was significantly higher on PCL/PVPA-AA scaffolds compared to PCL and TCP substrates.
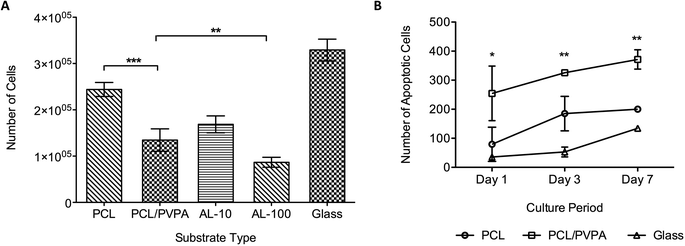 |
| Fig. 5 (a) Osteoclast cell number after 14 days in culture on PCL/PVPA, AL-10, AL-100, PCL and glass substrates. (b) The number of apoptotic cells present on PCL/PVPA, PCL and glass substrates over a 7-day culture period. 10 μmol and 100 μmol alendronate conditioned media, referred to as AL-10 and AL-100 respectively. Mean ± sd, n = 9 triplicates (*p ≤ 0.05, **p ≤ 0.01, ***p ≤ 0.001). | |
4. Discussion
We have reported the effect of a novel polymeric scaffold containing a phosphonic acid polymer on osteoblast and osteoclast cells. The electrospun PCL/PVPA-AA scaffold provides a biomimetic substrate for cell attachment and proliferation.25–27 Previously, we have demonstrated that the material has the physical and chemical properties to be used as a bone graft substitute in the repair of challenging bone defects.25 The PVPA-AA polymer is hypothesised to provide biological cues to stimulate bone formation and healing.
The PVPA-AA polymer has been found to affect cell metabolic activity in a dose dependant manner. A study by Tan et al., demonstrated a similar relationship when investigating protein adsorption on phosphonic acid containing substrates.30 Protein adsorption peaked in a dose dependent manner; this is most likely to be owed to the anionic functional groups present on the substrate. As the PVPA-AA concentration is increased, there is a greater repulsion between negatively charged serum proteins such as albumin and the PVPA-AA polymer, which consequently leads to a decrease in protein adsorption. Protein adsorption has been found to mediate cell proliferation; therefore lower protein adsorption would lead to a decrease in cell proliferation.
The incorporation of PVPA-AA into PCL fibres affects the in vitro cell response. There was an increase in osteoblast cell number over a 21-day culture period (Fig. 1b) on all scaffold types, however a significant increase was observed in the presence of PVPA-AA. The increase in cell number is confirmed in Fig. 2, where the number of live cells is greater on PCL/PVPA-AA scaffolds. Superior cell spreading is observed on PCL/PVPA-AA scaffolds, which could be owed to the hydrophilic properties of the scaffolds, which aids protein adsorption and consequent cell attachment. A high number of dead cells were observed on PCL substrates, which is owed to the hydrophobic attachment surface.25,27
There was no significant difference in collagen type I deposition from day 14 to day 21, suggesting that after day 14, no new matrix is deposited and instead the existing matrix is mineralised. This is in agreement with alizarin red staining where intense staining was observed from day 14 (Fig. 4). As described earlier, the initiation of mineralisation requires a critical concentration of both calcium and phosphorous. It is suggested that the phosphorous concentration is reached more efficiently as alkaline phosphatase is able to dephosphorylate the phosphorous containing molecules to produce free phosphate for subsequent calcium apatite formation. This theory would predict an increase in alkaline phosphatase activity. This increase was observed on PCL/PVPA-AA scaffolds from day 7 therefore reinforcing the view that the phosphorous concentration required to initiate mineralisation is reached more efficiently. As well as the phosphorous concentration increasing, it is likely that the –COOH groups present in the PVPA-AA polymer are able to chelate calcium ions and therefore produce calcium apatite. A previous ex vivo study investigating the healing of critical size calvarial defects has demonstrated apatite formation.26
The PVPA-AA polymer has been hypothesised to induce osteoclast apoptosis. The culture of osteoclast precursor cells demonstrated that after 14 days, osteoclast number significantly decreased (Fig. 5a) when compared to PCL (p ≤ 0.001) and glass (p ≤ 0.001) substrates. Moreover, osteoclast number was comparable to that obtained in cultures containing a commercially available bisphosphonate. The decrease in cell number on PCL/PVPA-AA, AL-10 and AL-100 substrates suggests that like alendronate, PVPA-AA is inducing apoptosis in osteoclast cells. The study has demonstrated that OPG expression is not effected by the PVPA-AA polymer as no difference was observed between the scaffold types. It was hypothesised that PVPA-AA could effect osteoclast formation by an increase in the expression of OPG. The increase in OPG was predicted to prevent the RANK–RANKL interaction and hence prevent osteoclast formation. However the study has suggested that this is not the mechanism of action as there is not a significant difference in its expression between the substrate types.
It is hypothesised that the presence of PVPA-AA in culture leads to the formation of nonhydrolysable analogues of ATP, AppCp. The accumulation of these analogues within the osteoclast cells leads to loss of its function and eventual cell death. From SEM analysis (data not shown), it has been shown that cells are present at day 1, suggesting that cells are not undergoing apoptosis immediately upon treatment with PVPA-AA. The presence of cells at day 14, also suggests that not all osteoclasts undergo apoptosis. Total osteoclast apoptosis in vivo would be undesirable as osteoclasts are required for healthy bone remodelling.
The prolonged use of bisphosphonates has been associated with osteonecrosis of the jaw, irritation of the gastrointestinal tract and kidney damage. As the PVPA-AA polymer has been found to have a similar effect on osteoclasts as bisphosphonates, it could offer a possible replacement of bisphosphonate therapy, which could reduce the risk of such long-term side effects as well improving bone stock locally.
5. Conclusions
We have described for the first time the effects of a 3D electrospun scaffold comprised of a phosphonic acid polymer on cell attachment and proliferation. We report osteoblast differentiation and maturation, and a significant increase in osteoblast mineralisation. Viable osteoclast cell number is reduced in the presence of the PVPA-AA polymer, a higher number of apoptotic cells were observed. The combined positive effects of the novel polymer scaffold on osteoblasts and osteoclasts may lead to successful bone repair and healing.
Acknowledgements
The author would like to thank The National Institute for Health Research and The Biotechnology and Biological Sciences Research Council for funding.
References
- M. Doblaré, J. M. García and M. J. Gómez, Modelling bone tissue fracture and healing: a review, Eng. Fract. Mech., 2004, 71, 1809–1840 CrossRef PubMed.
-
M. Zakikhani, Phosphonic acid polymers, US Patent, 5980776, 1999 Search PubMed.
- U. A. Liberman, S. R. Weiss, J. Broll, H. W. Minne, H. Quan and N. H. Bell,
et al. Effect of oral alendronate on bone mineral density and the incidence of fractures in postmenopausal osteoporosis, N. Engl. J. Med., 1995, 333, 1437–1443 CrossRef CAS PubMed.
- T. P. Van Staa, L. Abenhain and C. Cooper, Use of cyclical etidronate and prevention of non-vertebral fractures, Br. J. Rheumatol., 1998, 37, 87–94 CrossRef CAS.
- N. B. Watts, S. T. Harris, H. K. Genant, R. D. Wasnich, P. D. Miller and R. D. Jackson, Intermittent cyclical etidronate treatment of postmenopausal osteoporosis, N. Engl. J. Med., 1990, 323, 73–79 CrossRef CAS PubMed.
- M. Sato, W. Grasser, N. Endo, R. Akins, H. Simmons and D. D. Thompson,
et al., Bisphosphonate action. Alendronate localisation in rat bone and effects on osteoclast ultrastructure, J. Clin. Invest., 1991, 88, 2095–2105 CrossRef CAS PubMed.
- M. Ito, M. Chokki, Y. Ogino, Y. Satomi, Y. Azuma and T. Ohta, Comparison of the cytotoxic effects of bisphosphonates in vitro and in vivo, Calcif. Tissue Int., 1998, 63, 143–147 CrossRef CAS.
- A. M. Flanagan and T. J. Chambers, Dichloromethylenebisphosphonate (Cl2MBP) inhibits bone resorption through injury to osteoclasts that resorb Cl2MBP-coated bone, Bone Miner., 1989, 6, 33–43 CrossRef CAS.
- V. Breuil, F. Cosman, L. Stein, W. Horbert, J. Nieves and V. Shen,
et al., Human osteoclast formation and activity in vitro: effects of alendronate, J. Bone Miner. Res., 1998, 13, 1721–1729 CrossRef CAS PubMed.
- P. Masarachia, M. Weinreb, R. Balena and G. A. Rodan, Comparison of the distribution of 3H-alendronate and 3H-etidronate in rat and mouse bones, Bone, 1996, 19, 281–290 CrossRef CAS.
- F. H. Ebetino, M. D. Francis, M. J. Rogers and R. G. G. Russell, Mechanisms of action of etidronate and other bisphosphonates, Rev. Contemp. Pharmacother., 1998, 9, 233–243 CAS.
- M. J. Rogers, R. J. Brown, V. Hodkin, R. G. G. Russell and D. J. Watts, Bisphosphonates are incorporated into adenine nucleotides by human aminoacyl-tRNA synthetase enzymes, Biochem. Biophys. Res. Commun., 1996, 224, 863–869 CrossRef CAS PubMed.
- S. Pelorgeas, J. P. Martin and M. Satre, Cytotoxicity of dichloromethane diphosphonate and of 1-hydroxyethane-1,1-diphosphonate in the amoebae of the slime mould Dictyostelium discoideum, Biochem. Pharmacol., 1992, 44, 2157–2163 CrossRef CAS.
- J. C. Frith, J. Mönkkönen, G. M. Blackburn, R. G. G. Russell and M. J. Rogers, Clodronate and liposome-encapsulated clodronate are metabolized to a toxic ATP analog, adenosine 5′-b, g-dichloromethylene) triphosphate, by mammalian cells in vitro, J. Bone Miner. Res., 1996, 12, 1358–1367 CrossRef PubMed.
- M. J. Rogers, R. G. G. Russell, G. M. Blackburn, M. P. Williamson and D. J. Watts, Metabolism of halogenated bisphosphonates by the cellular slime mould Dictyostelium discoideum, Biochem. Biophys. Res. Commun., 1992, 189, 414–423 CrossRef CAS.
- M. J. Rogers, X. Ji, R. G. G. Russell, G. M. Blackburn, M. P. Williamson and A. V. Bayless, Incorporation of bisphosphonates into adenine nucleotides by amoebae of the cellular slime mould Dictyostelium discoideum, Biochem. J., 1994, 303, 303–311 CAS.
- H. Monkkonen, M. J. Rogers, N. Makkonen, S. Niva, S. Auriola and J. Monkkonen, The cellular uptake and metabolism of clodronate in RAW 264 macrophages, Pharm. Res., 2001, 18, 1550–1555 CrossRef CAS.
- J. C. Frith, J. Monkkonen, S. Auriola, H. Monkkonen and M. J. Rogers, The molecular mechanism of action of the anti-resorptive and anti- inflammatory drug clodronate: evidence for the formation in vivo of a metabolite that inhibits bone resorption and causes osteoclast and macrophage apoptosis, Arthritis Rheum., 2001, 244, 2201–2210 CrossRef.
- P. P. Lehenkari, M. Kellinsalmi, J. P. Napankangas, K. V. Ylitalo, J. Monkkonen and M. J. Rogers, Further insight into mechanism of action of clodronate: inhibition of mitochondrial ADP/ATP translocase by a nonhydrolyzable, adenine-containing metabolite, Mol. Pharmacol., 2002, 61, 1255–1262 CrossRef CAS.
- L. Mortensen, P. Charles, P. J. Bekker, J. Digennaro and C. C. Johnston Jr., Risedronate increases bone mass in an early postmenopausal population: two years of treatment plus one year of follow-up, J. Clin. Endocrinol. Metab., 1998, 83, 396–402 CrossRef CAS.
- G. G. Reinholz, B. Getz, L. Pederson, E. S. Sanders, M. Subramaniam and J. N. Ingle,
et al., Bisphosphonates Directly Regulate Cell Proliferation, Differentiation, and Gene Expression in Human Osteoblasts, Cancer Res., 2000, 60, 6001–6007 CAS.
- G. Im, S. A. Qureshi, J. Kenney, H. E. Rubash and A. S. Shanbhag, Osteoblast proliferation and maturation by bisphosphonates, Biomaterials, 2004, 25, 4105–4115 CrossRef CAS PubMed.
- M. Tanahashi and T. Matsuda, Surface functional group dependence on apatite formation on self-assembled monolayers in a simulated body fluid, J. Biomed. Mater. Res., 1997, 34, 305–315 CrossRef CAS.
- P. Ducy, C. Desbois, B. Boyce, G. Pinero, B. Story and C. Dunstan,
et al., Increased bone formation in osteocalcin-deficient mice, Nature, 1996, 382, 448–452 CrossRef CAS PubMed.
- A. K. Bassi, J. E. Gough, M. Zakikhani and S. Downes, The Chemical and Physical Properties of PCL Scaffolds functionalised with Poly(vinyl phosphonic acid-co-acrylic acid), SAGE Open, 2011 DOI:10.4061/2011/615328.
- A. K. Bassi, J. E. Gough, M. Zakikhani and S. Downes, A novel phosphonate for the repair of critical size bone defects, J. Tissue Eng. Regener. Med., 2012, 6, 833–840 CrossRef CAS PubMed.
-
A. K. Bassi, J. E. Gough, M. Zakikhani and S. Downes, Bone Tissue Regeneration, in Electrospinning for Tissue Regeneration, ed. L. A. Bosworth and S. Downes, Woodhead Publishing, Cambridge, 2011, pp. 93–110 Search PubMed.
- L. Xing, E. M. Schwarz and B. F. Boyce, Osteoclast precursors, RANKL/RANK, and immunology, Immunol. Rev., 2005, 208, 19–29 CrossRef CAS PubMed.
- G. Hattersley, J. Owens, A. M. Flanagan and T. J. Chambers, Macrophage colony stimulating factor (M-CSF) is essential for osteoclast formation in vitro, Biochem. Biophys. Res. Commun., 1991, 177, 526–531 CrossRef CAS.
- J. Tan, R. A. Gemeinhart, M. Ma and W. M. Saltzman, Improved cell adhesion and proliferation on synthetic phosphonic acid-containing hydrogels, Biomaterials, 2005, 26, 3663–3671 CrossRef CAS PubMed.
|
This journal is © The Royal Society of Chemistry 2014 |