DOI:
10.1039/C3BM60162E
(Paper)
Biomater. Sci., 2014,
2, 212-219
Fabrication of viable cyborg cells with cyclodextrin functionality
Received
28th June 2013
, Accepted 27th August 2013
First published on 4th October 2013
Abstract
We describe two alternative methods for surface functionalisation of Saccharomyces cerevisiae cells with cyclodextrin molecules without affecting the cell viability. The first strategy involved using epichlorohydrin as a cross-linking agent which binds covalently the cyclodextrin to the glycoproteins on the cell wall. The second strategy of interfacing of the cells with CD involved polyelectrolyte mediated deposition of cyclodextrin sulphate on the cell surface. We used the formation of host–guest inclusion complexes of a dye with the grafted cyclodextrins to estimate the average number of CD molecules grafted per cell which can reach up to hundreds of millions of CD molecules. This indicates more than one monolayer of CDs on the cell surface within the surface layer surrounding the yeast cell membrane. Fluorescein diacetate was used to check the viability of the cells after functionalisation. Living cells functionalised with CDs may find many potential applications as they can be loaded with drugs, immunosuppressants and other molecules forming inclusion complexes with their cyclodextrin interface. Therefore, we foresee such cells being used as novel selective biosorbents in polluted waters, whole cell biosensors, drug delivery, cell therapy and cell implant applications.
Introduction
Interfacing living cells with nanoparticles and polymers is an emerging new field1–4 which brings together microbiology, surface chemistry, and physical chemistry of colloids. The modified cells can perform novel functions due to their different interface with the external media. Such “cyborg cells”1 have been created by using a variety of living cell species, ranging from microbial cells (bacteria,2 algae36 and fungi3,4), multi-cellular organisms5 (nematodes) to mammalian cells for a wide range of possible new applications. Many native cells bear negative surface charge due to dissociation of surface carboxylic groups originating from carbohydrates or proteins. This has been utilised in a range of cell functionalisation techniques based on the layer-by-layer (LbL) deposition of oppositely multi-charged species such as poly-electrolytes,6 magnetic particles,7,8 colloidal particles,9 metal nanoparticles6,10 and oligonucleotides. Cyborg cells produced using the LbL method have been used in whole-cell biosensors, toxicity micro-screening devices11 and cell-based therapies12 while non-viable cells have been used in areas such as biosorbents,13,14 biocatalysts and microelectronics.4 For example, magnetically-functionalised yeast cells were shown to effectively absorb heavy metal ions as well as industrial dyes.15 While cyborg cells interfaced with a variety of nanoparticles and polymers1–10 have already been fabricated, the surface functionalisation of living cells with cyclodextrins (CDs) has not yet been reported. Cyclodextrins are derived from starch by enzymatic reactions16–19 and are used as molecular containers in a range of pharmaceutical formulations,20,21 drug delivery applications,22–25 food,26,27 flavours28,29 and consumer personal care products.30 Cells functionalised with cyclodextrins may find many exciting new applications as living drug carriers where the cyclodextrins grafted on the cell surface can carry additional payload supplementing the cell's own functions. For example, cyclodextrin functionalised cyborg cells can be loaded with immunosuppressants and used as cell implants. In addition, such cells can be used as whole cell biosensors and biosorbents. However, grafting cyclodextrins to living cells can be a challenge as it requires aggressive reaction conditions which may adversely affect the cell viability.
Here we report for the first time a successful functionalisation of living yeast cells with three types of cyclodextrin (α-CD, β-CD and γ-CD). We developed two alternative strategies for interfacing cells with cyclodextrin. The first one involves using epichlorohydrin (EP) as a cross-linking agent as shown schematically in Scheme 1. This method does not significantly impact the cell viability and involves the following stages: (i) The cells are incubated in an aqueous solution of cyclodextrin. (ii) Epichlorohydrin is added to the cell dispersion which grafts the cyclodextrin molecules to the cell surface. (iii) The cyclodextrin-functionalised cells are filtered from the solution. The advantage of using EP as a cross-linker for CD is that the reaction can be carried out at room temperature at low EP concentration which preserves the cell viability. We have applied this method to functionalise yeast cells with cyclodextrins as a proof of principle.
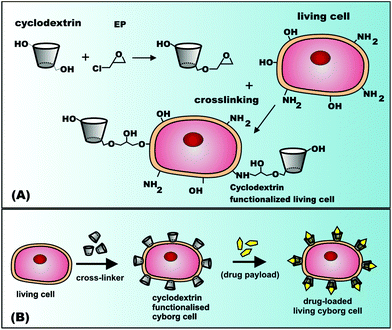 |
| Scheme 1 (A) Cross-linking reactions of living cells with cyclodextrins (CDs) and epichlorohydrin (EP). (B) Fabrication steps of living CD-functionalised cyborg cells loaded with drugs or immunosuppressants. | |
In addition, we employed a second strategy which involves the layer-by-layer deposition of cationic polyelectrolyte and β-CD sulphate on yeast cells and assessed the effect of the number of coats on the cell viability. We discuss both methods in detail below.
Materials and methods
Materials
Alpha cyclodextrin (α-CD), beta cyclodextrin (β-CD), gamma cyclodextrin (γ-CD), β-cyclodextrin sulphate sodium salt, epichlorohydrin (EP), fluorescein diacetate (FdA31), polyallylamine hydrochloride (PAH, MW 58 kDa) all of 98% purity were purchased from Sigma (UK) and were used without further purification. Methyl orange (MO, grade Reag. Ph. Eur.) was sourced from Sigma (UK). Deionised water was used in all experiments, obtained by using a Milli-Q® water system. Baker's dry yeast was purchased from TESCO (UK).
Functionalisation of yeast cells with cyclodextrins using epichlorohydrin as a cross-linker
The yeast cells were pre-washed several times with Milli-Q water prior to their functionalisation. The cross-linking reaction was carried out by using a fixed amount of yeast cells (∼0.5 g) while the concentration of cyclodextrin (α-CD, β-CD or γ-CD) in the reaction mixture was varied from 0 to 0.4% m/v. For each particular sample, a fixed amount of CD was dissolved in 5 mL Milli-Q water followed by addition of the cells and homogenisation with a magnetic stirrer. The CDs were grafted onto the cell surface by adding 2 μL of EP to each sample including the control one (without CD). After incubation (with stirring) in the reaction mixture at room temperature for 24 h the cell samples were centrifuged (3000 rpm for 5 minutes) and washed first with Milli-Q water and several times with 2 mM phosphate buffer solution at pH 7. The functionalisation of yeast cells with cyclodextrins using epichlorohydrin as a cross-linker was carried out under buffered conditions using sodium hydrogen phosphate buffer solution at pH 7. Hence pH does not change in the course of the reaction. Epichlorohydrin is potentially toxic for the cells at moderate and high concentrations;34 therefore a very small amount was used to reduce its cytotoxic effects on the cells so that the functionalized cells retained their viability after functionalisation. This protocol was used for yeast incubated with α-CD, β-CD and γ-CD. To evaluate the success of this procedure in grafting cyclodextrin to the surface of the yeast cells, we used the ability of the cyclodextrins to form host–guest non-covalent inclusion complexes. A sample of 75 mg of each batch of CD-functionalised yeast cells was incubated in a stock solution of 60 μM methyl orange in 2 mM phosphate buffer at pH 7 for 60 minutes under agitation. Subsequently, the cells were centrifuged and the supernatant was collected through a 0.2 μm Whatman® Anotop® syringe filter. The MO concentration in the filtrates was measured spectroscopically by using standard calibration curves from a series of MO solutions at the same pH 7 phosphate buffer concentration.
UV-vis spectroscopy
UV-vis spectroscopy was used to quantitatively analyse the formation of host–guest complexes between MO and the cyclodextrins attached covalently to the yeast cells surface. A series of standard aqueous solutions of MO in 2 mM sodium phosphate buffer at pH 7 were prepared in 25 mL volumetric flasks and their absorbance spectra from 600 nm to 200 nm were measured by a double beam Perkin Elmer UV-vis spectrometer Bio Lambda 40 using UV Winlab software. From these spectra, calibration curves were produced by plotting the absorbance at λmax = 460 nm against the MO concentration of a given standard aqueous solution. 2 mM of an aqueous solution of sodium phosphate buffer (pH 7) was used as a base line of the absorbance in the UV-vis instrument. The absorbance spectra of 60 μM MO filtrates after incubation with a sample of α-CD, β-CD or γ-CD functionalised yeast cells were also obtained by scanning the absorbance vs. the wavelength to find out how the absorbance at λmax was affected by the formation of MO–CD inclusion complexes on the cell surface. The amount of MO that formed inclusion complexes with α-CD, β-CD or γ-CD molecules was calculated using the calibration curve of the absorbance (at 460 nm) of aqueous standard solutions of MO. From the MO concentration in the filtrate of solution exposed to the same mass of CD-functionalised cells and a control experiment with non-functionalised yeast cells, the number of cyclodextrins per yeast cell was estimated using the equation | 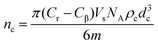 | (1) |
where nc is the number of β-CDs per cell, Cr is the MO residual concentration (in M) after filtering out the yeast cells (for the β-CDs non-treated) and Cβ is the equivalent concentration of MO after filtrating the β-CD-treated cells. The concentration difference in eqn (1) reflects the amount of MO retained in the MO–CD inclusion complexes on the cell surface. Here Vs is the volume of the MO stock solution (60 μM) incubating the sample of cells of mass m, NA is Avogadro's number, ρc is cell mass density and dc is the average cell diameter.
In this estimate, we assume that the cells are spherical and that each grafted CD molecule on the cell surface captures one MO molecule from the stock solution.
Viability test of the yeast cells
The viability of the cells after the functionalisation was assessed by using fluorescence microscopy. This was done by incubating a small amount of an aqueous dispersion of washed functionalised yeast cells with 1–2 drops of ethanol solution of fluorescein diacetate (FdA) solution for 10 minutes. The samples were covered with aluminium foil to reduce photo-bleaching and after 10 minutes the samples were washed multiple times with Milli-Q water and analysed with an Olympus BX-51 fluorescence microscope using a mercury vapour lamp as a light source and an Olympus Burner U-RFL-T-200 for mercury vapour excitation. The yeast cells were irradiated with blue light (435 nm) using FITC filter sets. The viability test compares the number of fluorescing cells and non-fluorescing cells in a given sample on the microscope glass slide. Thus counting of the fluorescing and the non-fluorescing cells was done by overlaying images from fluorescence microscopy and brightfield optical microscopy of the same field of view of the sample on the microscope glass slide and then counting the fluorescing cells out of that total number of cells.
Influence of epichlorohydrin on the number of cyclodextrins grafted on the yeast cells
This was investigated using β-CD and carried out by keeping the mass of yeast cells and the mass of cyclodextrins fixed but varying the amount of EP in the reaction mixtures. The influence of varying EP concentration on the uptake of methyl orange by the non-functionalised cells was demonstrated by running control experiments where the amount of yeast cells was the same but there was no cyclodextrin in the reaction mixture. EP may cause cell–cell binding which would manifest itself as a cell aggregation. However, we did not observe significant cell aggregation by studying the cells under an optical microscope which suggested that the reaction was mostly confined to CD-cell surface binding. We attribute this to the moderately low cell concentration in the reaction mixture and the stirring of the solution during the cross-linking reaction.
Functionalisation of yeast cells using layer-by-layer sequential deposition of PAH and β-cyclodextrin sulphate
Yeast cells were first subjected to a cycle of washing with Milli-Q water/centrifugation (4 times) to remove any impurities. The functionalisation was carried out by sequential deposition of PAH and β-CD sulphate. In this procedure, a sample of 0.4 g of yeast cells dispersed in 3 mL of Milli-Q water was first delivered by an SP100i syringe pump (flow rate 6 mL h−1) to a stirred (250 rpm) 3 mL of 1 mg mL−1 aqueous solution of PAH. The excess PAH after the deposition step was rinsed 4 times with Milli-Q water through a cycle of centrifugation (3000 rpm for 5 min) and decanting the supernatant. Then, the PAH pre-coated yeast cells were re-dispersed in 3 mL Milli-Q water and this then was delivered by an SP100i syringe pump (flow rate 6 mL h−1) to a stirred (250 rpm) 3 mL of 1 mg mL−1 aqueous solution of β-cyclodextrin sulphate. The excess β-cyclodextrin sulphate after the adsorption step was rinsed 4 times with Milli-Q-water through a cycle of centrifugation (3000 rpm for 5 min) and decanting the supernatant leaving a second layer of negatively charged β-CD sulphate on the yeast surface. The above procedure was repeated up to four times until 4 layers of PAH-β-CD sulphate were produced. Some variations in the concentrations of both PAH and β-CD sulphate were introduced (2 and 3 mg mL−1) while keeping all the other parameters constant. The aqueous phase was modified using 1 M NaCl.
Determination of the yeast cell average cell diameter
The yeast cell size distribution was measured by using a Malvern Mastersizer 2000 coupled to a Hydro 2000sm dispersion unit. The background was set by running the measurement in Milli-Q water without the sample. The samples were then added manually to a dispersion tank filled with Milli-Q-water and stirred at 330 rpm. The diluted samples were then pumped into the measurement cell of the Mastersizer. The cell size distribution was calculated from Fraunhofer's model using water as a dispersant with a refractive index of 1.33. The average of ten runs was taken as a representation of measured particle size distribution.
Zeta potential measurements
The functionalisation of yeast cells by consecutive deposition of PAH and β-CD sulphate was monitored by measuring the cells’ zeta potential. After each deposition, cells were washed by a cycle of centrifugation thrice and subsequently dispersed in Milli-Q-water to dilute them. The zeta potential of the dispersed cells was measured using a Malvern Zeta 3000HS with a flow-through sample cell. The average of 10 measurements was taken to represent the measured potential.
Results and discussion
In this study, we have established two methods of functionalising living yeast cells with cyclodextrin molecules which allow active components to be encapsulated on the cell surface. The protocols involved in both cases are simple, preserve the cell viability and do not involve expensive equipment or chemicals.
Functionalisation of living yeast cells with CDs using epichlorohydrin as a cross-linker
Scheme 1 shows only two of the possible reactions of cross-linking of CDs to the surface of cells. However, the opening of the epoxy ring of EP can also produce other side reactions of conjugation of CD in complex polymers.32
Fig. 1 shows the absorbance of the MO solution filtrates after incubation with a fixed amount of cells functionalised at a constant amount of EP but varying concentrations of various CDs in the reaction mixture.
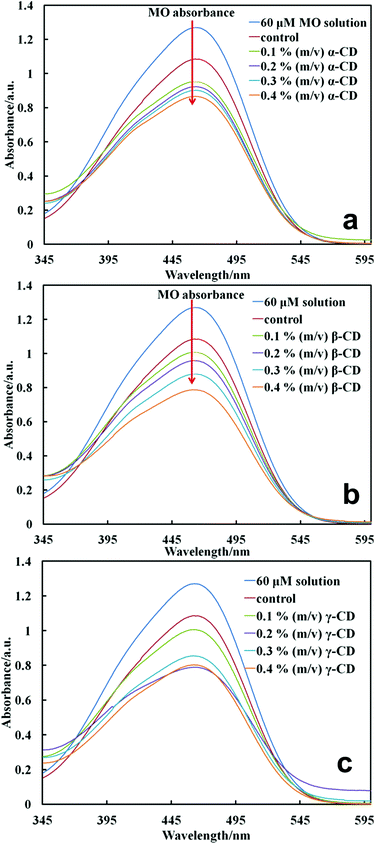 |
| Fig. 1 Absorbance of the filtrate of MO solution after incubation with cells functionalised with CDs at different concentrations in the reaction mixture at 5 mM EP. Results are presented with cells functionalised with (A) α-CD, (B) β-CD and (C) γ-CD. The graphs also give the absorbance of the filtrate from the control experiment with cells which were not treated with CD and the stock solution of MO. The red arrows show the decrease in MO absorbance as CD concentration is increased. | |
One sees that the functionalisation of the cell with CD consistently lowered the peak absorbance of MO with the increase of the CD concentration in the reaction mixture. Note that the control sample (cells treated with EP without CDs) also lowered the peak absorbance of MO but to a lower extent compared to cell samples cross-linked with CDs. This is related to the non-specific adsorption of MO on the cell surface layer. Since the binding constant of this type of dye in the inclusion CD complex is very large17 and the MO concentration in the stock solution is high we can assume that all CD molecules on the cell surface host an MO molecule upon incubation. The binding constant of MO–α-CD is 7300 mol−1 dm3 while MO–β-CD has a value of 2700 mol−1 dm3 for 1
:
1 complexes according to studies done by Hamai and Handa.33 From the material balance of the specifically adsorbed MO on the CDs grafted on the cell surface we estimated the number of CD molecules per cell using eqn (1). The average diameter of the yeast cells was 6 ± 1 μm from the Mastersizer measurement. Note that the absorbance of MO in the filtrates varies for a series of cell samples functionalised with CD (α-CD, β-CD and γ-CD) at different concentrations in the reaction mixture and a fixed concentration of EP. As expected, the depletion of the MO from the stock solution depends on the type of CD used for the cell functionalisation. This was not surprising because CDs have different cavity volumes which determined whether the incoming dye guest molecule formed a perfect fit, a loose fit or was too big to fit in the CD cavity. As seen in Fig. 1, comparing the cells functionalised with the same highest amount of CDs (∼0.4% g mL−1) and incubated in MO at the same concentration, β-CD functionalised cells showed the lowest maximum absorbance. We observed a consistent increase in the number of CDs per cell with the increase of the CD concentration upon grafting (Fig. 2a). This was consistent with our expectations since we were relying on the absorbance of the dyes, i.e. the dye which was depleted from the solution must be trapped in the CD cavity, hence as more and more dye molecules were trapped by CDs grafted on the cell few were left in the filtrate therefore giving a low absorbance maximum. However, we found that the extent to which the maximum absorbance was lowered depended on the type of CD used. In Fig. 2a, the number of CD molecules grafted per cell is not linearly dependent on CD concentration, especially at the γ-CD concentration of 0.2% (m/v). There are two possible factors which may contribute to this non-linearity of the attached γ-CD per cell and the γ-CD concentration: (i) the number of CD molecules grafted per cell was estimated using host–guest interactions between the CD hydrophobic cavity and the methyl orange. These interactions are formed through non-covalent bonds and depend on the perfect fit between the host and the guest molecules. Therefore there is a different dynamic equilibrium between the complex and free molecules, which could explain this observed non-linearity especially for γ-CD with the largest cavity which forms the least stable complex with methyl orange. (ii) The reactivity of the 6′-OH groups of the γ-CD can be different from those of α-CD and β-CD due to the higher conformational flexibility of the γ-CD molecule.
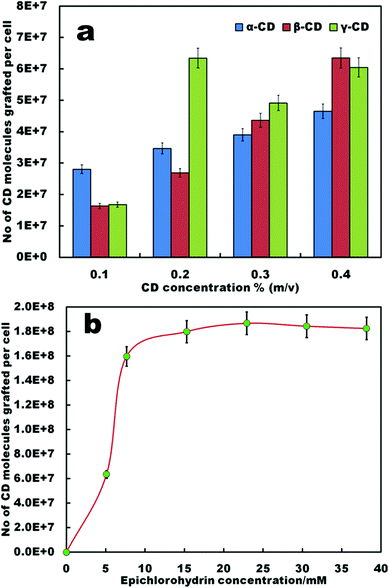 |
| Fig. 2 (a) Number of CD molecules grafted per cell as a function of the concentration of CD (α, β and γ) in the reaction mixture at fixed EP concentration (5 mM). (b) Effect of the EP concentration on the number of CDs grafted per cell at fixed CD concentration (0.4% m/v β-CD). | |
We studied the effect of the EP cross-linker concentration on the average number of CD molecules per cell by using a fixed amount (0.5 g) of yeast cells and β-CD at ∼0.4% m/v while varying the EP concentration in the reaction mixture. Fig. 2b shows that for β-CD functionalised cells, increasing the EP concentration leads to a sharp increase in the average number of CD molecules grafted per cell and a plateau was reached above 12 mM EP which was an indication of saturation of the binding sites for EP on the cell surface layer. However, it has been reported that the presence of EP in the solution may have a cytotoxic effect on the exposed cells even at low and moderate EP concentrations for a range of mammalian cells.34
We tested the viability of the CD-functionalised yeast cells by using fluorescein diacetate (FdA31). FdA is a non-ionic dye which diffuses through the cell membrane and can undergo hydrolysis by non-specific esterase to produce the fluorescein molecule which accumulates inside the cells which can be detected by fluorescence microscopy.18 Upon treatment with FdA the yeast cells turn fluorescent if their cell membranes are intact and the enzymes in the cell interior are active. Our FdA test results show that most of the yeast cells exhibit green fluorescence which indicates that the cells retained their viability after functionalisation with CD in the presence of EP (Fig. 3).
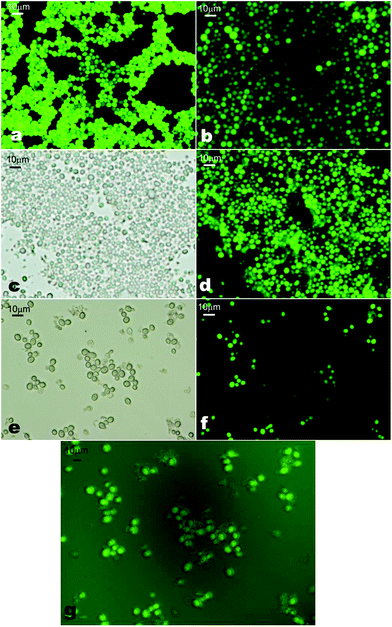 |
| Fig. 3 Cell viability test with fluorescein diacetate showing the cell fluorescence after functionalisation with β-CDs. (a) and (b) are the control samples (non-treated) in bright field and fluorescence microscopy with an FITC filter set. (c) and (d) are yeast cells functionalised with 0.1% m/v β-CD in bright field and fluorescence microscopy, respectively, while (e) and (f) show the yeast cells functionalised with 0.4% m/v β-CD. The concentration of the EP cross-linker used in both experiments was 0.6 mM. (g) Overlay of images E and F showing that above 80% of the cells retain their viability. | |
Functionalisation of yeast cells by LbL deposition of PAH and β-cyclodextrin sulphate
In addition to grafting CD molecules to the yeast cells using EP as a cross-linking agent, we exploited the availability of the negative surface charges present on the native yeast cells due to the ionization of polysaccharides making up the cell wall. We used the negative charges of the yeast cells to anchor cationic polyelectrolyte (PAH) and deposit anionic β-CD sulphated sodium salt by LbL deposition. Scheme 2 shows the schematic for the LbL functionalisation of yeast cells with β-CD sulphate. After deposition of each PAH/β-CD sulphate layer on the yeast cells, the dispersion was washed by a cycle of re-dispersing in Milli-Q water and centrifugation at 3000 rpm for 5 minutes (four times) to remove the excess polyelectrolyte. The presence of each deposited thin layer was assessed by measuring the zeta potential of the functionalised cells after washing and re-dispersing in Milli-Q water. We also varied the concentrations of the polyelectrolytes from 1 mg mL−1 to 3 mg mL−1. As can be seen from Fig. 4, the success of the sequential build-up of the coatings from PAH and β-cyclodextrin sulphate was reflected in oscillation of the zeta potential of the coated cells. When PAH was the outer coat, the zeta potential increased (positive) compared to the non-coated yeast cells (negative). The PAH does not get internalised by the yeast cells due to the relatively thick (about 200 nm) cell wall consisting of glycoproteins and carbohydrates which surrounds the lipid membrane. The PAH coated cells were stable since the cells were washed by centrifugation and when observed under an optical microscope, the cells still maintained their integrity and the zeta potential measurement showed that the PAH was on their surface as the potential was positive (ζ ≈ +40 mV) while the non-coated cells are negatively charged (ζ ≈ −20 mV). Note that when the β-CD sulphate was the outer layer, the measured zeta potential was more negative than the non-coated yeast cells. The reversal of zeta potential in the LbL deposition has been reported in many articles to confirm the deposition of oppositely charged polyelectrolytes (e.g. see ref. 2). For example, Hillberg and Tabrizian have shown the charge alternation with successive deposition of chitosan (polycation) and alginate (polyanion) to encapsulate E. coli.12 This result therefore was a confirmation of the functionalisation of yeast cells with modified β-CD. We observed that as the number of layers was increased, the zeta potential became slightly more negative and became more positive on increasing the layering. This shows that the cell's surface becomes more charged with the increase in the number of deposited layers and this trend was observed for all concentrations up to 4 layers. As indicated above epichlorohydrin is cytotoxic;34 therefore the functionalisation of cells using epichlorohydrin as a cross-linker may be an issue for very sensitive cell cultures and in vivo applications. This is why we also used the layer-by-layer method as an alternative method for this situation. In addition the PAH in this procedure may also be replaced with more biocompatible polycations such as chitosan or branched polyethyleneimine (PEI) for CD-functionalisation of more sensitive cell cultures and for safer in vivo applications of the functionalised cells.
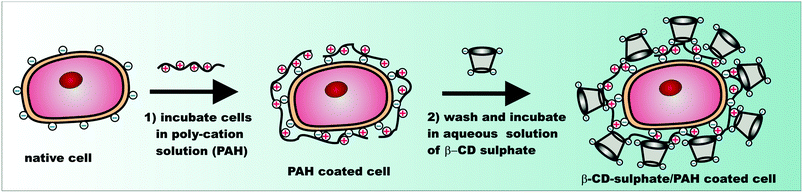 |
| Scheme 2 Schematic illustrating the sequential deposition of cationic polyelectrolyte (PAH) and β-CD sulphate to functionalise the cell surface with CD. | |
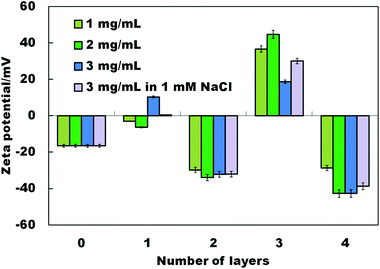 |
| Fig. 4 Zeta potential change with the sequential deposition of PAH (polycation) and β-CD sulphate on the surface of viable yeast cells. | |
The best results of LbL deposition were achieved at 2 mg mL−1 polyelectrolyte and 2 mg mL−1 β-CD sulphate concentrations. The thickness, strength and morphology of polyelectrolyte layers can be further tailored by altering the pH, the ionic strength and the poly-ion materials.35 We investigated the effect of the ionic strength by depositing the polyelectrolytes from a solution of 1 mM NaCl. As can be seen in Fig. 4, the sequential deposition of PAH and β-CD sulphate in the presence of 1 mM NaCl reduced very slightly the magnitude of the zeta potential which is attributed to the electrostatic screening.
The calculation of the number of CDs deposited on the cell surface by layer-by-layer assembly is challenging due to the presence of PAH between the CD layers as it also adsorbs methyl orange molecules electrostatically in addition to the ones that bind to the CDs. However, we have shown using zeta potential measurements that the β-CD sulphate molecules were deposited on the surface of the cells as shown by the cell surface charge reversal (see Fig. 4).
Cell viability of CD-functionalised cells by the LbL method
We monitored the viability of the cells after each deposition using FdA. Samples taken after each layer deposition were incubated in a solution by adding a drop of FdA solution (5 mg mL−1) dissolved in acetone. We observed that with the increase of the number of PAH/β-CD sulphate layers, the fluorescence intensity of the cells decreased. After the fourth layer, the viability as tested with FdA visibly worsened and hence we only applied this LbL procedure up to four layers. One possible explanation for this result could be that the build-up of CD molecules on the cell surface depletes the FdA which can lead to lower penetration in the cell interior. We also observed some partial aggregation in CD-functionalised cells compared to the native ones. This was dependent on the way of introducing the cells into the polyelectrolyte solution and could be subject to further optimisation to avoid formation of large aggregates. Fig. 5 shows the micrographs of the bare yeast cells and after the deposition of the fourth layer of PAH/β-CD sulphate.
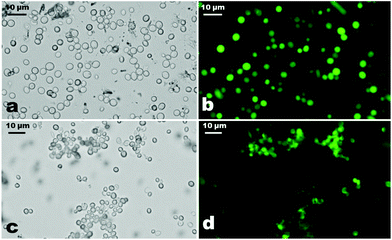 |
| Fig. 5 Micrographs showing the viability of yeast cells tested with FdA. Transmitted light microscopy image for bare yeast cells (a) and (b) fluorescence micrographs for bare yeast cells while (c) and (d) show transmitted and fluorescence micrographs after the deposition of four consecutive layers of PAH and β-CD sulphate. | |
In practical applications, it could be a good idea to check the stability of the CD/PAH/cells in the presence of a serum. We will make such tests in future publications of results with CD-functionalised cells for particular applications. However, even the present results indicate that the CD/PAH-coated cells are stable upon further coating with oppositely charged polyelectrolytes as presented in Fig. 4. Thus, further treatment with PAH and β-CD sulphate does not incur cell aggregation provided that the polyelectrolyte is in excess with respect to the cells. However, adding small amounts of polyelectrolyte of opposite charge to the cell suspension causes cell aggregation rather than charge reversal.
Conclusions
In summary, we produced a new type of cyborg cells functionalised with three different types of CDs using epichlorohydrin as a cross-linking agent without affecting the cell viability. We used the formation of host–guest inclusion complexes of methyl orange dye with the grafted CD to estimate the average number of CD molecules cross-linked per cell which can reach up to tens of millions. In addition, we employed the layer-by-layer deposition to functionalise living yeast cells with cationic polyelectrolyte and β-CD sulphate. Living cells functionalised with CDs may find many potential applications as they can be loaded with drugs, immunosuppressants and other molecules forming inclusion complexes with their cyclodextrin interface. Therefore, we foresee such cells being used as novel selective biosorbents in polluted waters, whole cell biosensors, drug delivery, cell therapy and cell implant applications. The cyclodextrin-functionalized cells are not limited to being able to encapsulate drug molecules on their surfaces. Such cells can also be used as biosorbents14 in polluted waters where they use cyclodextrins on their surfaces to extract pollutant molecules of the right size from water depending on the cyclodextrin used. All the other potential applications of these cells are based on utilizing the CDs on the cell surface as encapsulating sites.
Acknowledgements
BGM would like to thank Botswana College of Agriculture for financial support. V. N. P. appreciates support from the COST action CM1101.
Notes and references
- R. F. Fakhrullin, A. I. Zamaleeva, R. T. Minullina, S. A. Konnova and V. N. Paunov, Chem. Soc. Rev., 2012, 41, 4189–4206 RSC.
- R. F. Fakhrullin, L. V. Shlykova, A. I. Zamaleeva, D. K. Nurgaliev, Y. N. Osin, J. García-Alonso and V. N. Paunov, Macromol. Biosci., 2010, 10, 1257–1264 CrossRef CAS PubMed.
- A. I. Zamaleeva, A. V. Porfireva, G. A. Evtugyn and R. F. Fakhrullin, Langmuir, 2010, 26, 2671–2679 CrossRef CAS PubMed.
- R. Kempaiah, S. Salgado, W. L. Chung and V. Maheshwari, Chem. Commun., 2011, 47, 11480 RSC.
- R. T. Minullina, Y. N. Osin, D. G. Ishmuchametova and R. F. Fakhrullin, Langmuir, 2011, 27, 7708–7713 CrossRef CAS PubMed.
- R. F. Fakhrullin, A. I. Zamaleeva, M. V. Morozov, D. I. Tazetdinova, F. K. Alimova, A. K. Hilmutdinov, R. I. Zhdanov, M. Kahraman and M. Culha, Langmuir, 2009, 25, 4628–4634 CrossRef CAS PubMed.
- J. García-Alonso, R. F. Fakhrullin and V. N. Paunov, Biosens. Bioelectron., 2010, 25, 1816–1819 CrossRef PubMed.
- M. R. Dzamukova, A. I. Zamaleeva, D. G. Ishmuchametova, Y. N. Osin, A. P. Kiyasov, D. K. Nurgaliev, O. N. Ilinskaya and R. F. Fakhrullin, Langmuir, 2011, 27, 14386–14393 CrossRef CAS PubMed.
- R. F. Fakhrullin and R. T. Minullina, Langmuir, 2009, 25, 6617–6621 CrossRef CAS PubMed.
- S. H. Yang, E. H. Ko and I. S. Choi, Langmuir, 2012, 28, 2151–2155 CrossRef CAS PubMed.
- J. García-Alonso, R. F. Fakhrullin, V. N. Paunov, Z. Shen, J. D. Hardege, N. Pamme, S. J. Haswell and G. M. Greenway, Anal. Bioanal. Chem., 2010, 400, 1009–1013 CrossRef PubMed.
- A. L. Hillberg, Biomacromolecules, 2006, 7, 2742–2750 CrossRef CAS PubMed.
- D. Park, Y.-S. Yun and J. M. Park, Biotechnol. Bioprocess Eng., 2010, 15, 86–102 CrossRef CAS PubMed.
- J. Wang and C. Chen, Biotechnol. Adv., 2009, 27, 195–226 CrossRef CAS PubMed.
- I. Safarik, K. Pospiskova, K. Horska and M. Safarikova, Soft Matter, 2012, 8, 5407–5413 RSC.
- A. Biwer, G. Antranikian and E. Heinzle, Appl. Microbiol. Biotechnol., 2002, 59, 609–617 CrossRef CAS PubMed.
- J. Szejtli, Chem. Rev., 1998, 98, 1743–1754 CrossRef CAS PubMed.
- D. Landy, I. Mallard, A. Ponchel, E. Monflier and S. Fourmentin, Environ. Chem. Lett., 2012, 10, 225–237 CrossRef CAS PubMed.
- T. V. Frank van de Manakker, C. F. van Nostrum and W. E. Hennink, Biomacromolecules, 2009, 10, 3157–3175 CrossRef PubMed.
- M. Singh, R. Sharma and U. C. Banerjee, Biotechnol. Adv., 2002, 20, 341–359 CrossRef CAS.
- M. E. Brewster and T. Loftsson, Adv. Drug Delivery Rev., 2007, 59, 645–666 CrossRef CAS PubMed.
- R. Challa, A. Ahuja, J. Ali and R. Khar, AAPS PharmSciTech, 2005, 6, E329–E357 CrossRef PubMed.
- A. Vyas, S. Saraf and S. Saraf, J. Inclusion Phenom. Macrocyclic Chem., 2008, 62, 23–42 CrossRef CAS.
- R. Arun, Sci. Pharm., 2008, 76, 567–598 CrossRef PubMed.
- D. Duchêne, G. Ponchel and D. Wouessidjewe, Adv. Drug Delivery Rev., 1999, 36, 29–40 CrossRef.
- L. Szente and J. Szejtli, Trends Food Sci. Technol., 2004, 15, 137–142 CrossRef CAS PubMed.
- G. Astray, C. Gonzalez-Barreiro, J. C. Mejuto, R. Rial-Otero and J. Simal-Gandara, Food Hydrocolloids, 2009, 23, 1631–1640 CrossRef CAS PubMed.
- E. M. M. Del Valle, Process Biochem., 2004, 39, 1033–1046 CrossRef CAS.
- H. M. C. Marques, Flavour Fragrance J., 2010, 25, 313–326 CrossRef.
- J. Szejtli, J. Mater. Chem., 1997, 7, 575–587 RSC.
- Please note that FdA in this paper stands for fluorescein diacetate and should not be confused with the abbreviation for the US Food and Drug Administration which is also used in the literature.
- E. Renard, A. Deratani, G. Volet and B. Sebille, Eur. Polym. J., 1997, 33, 49–57 CrossRef CAS.
- S. Hamai and M. Handa, Anal. Lett., 1999, 32, 1037–1047 CrossRef CAS.
- W. H. Lawrence, M. Malik, J. E. Turner and J. Autian, J. Pharm. Sci., 1972, 61, 1712–1717 CrossRef CAS.
- A. J. Priya, S. P. Vijayalakshmi and A. M. Raichur, J. Agric. Food Chem., 2011, 59, 11838–11845 CrossRef CAS PubMed.
- D. Zhang, R. F. Fakhrullin, M. Özmen, H. Wang, J. Wang, V. N. Paunov, G. Li and W. E. Huang, J. Microbial Biotech., 2011, 4, 89–97 CrossRef CAS PubMed.
|
This journal is © The Royal Society of Chemistry 2014 |