DOI:
10.1039/C2RA21918B
(Paper)
RSC Adv., 2013,
3, 111-116
Formation of blue fluorescent ribbons of 4′,4′′′′-(1,4-phenylene)bis(2,2′:6′,2′′-terpyridine) and highly selective visual detection of iron(II) cations†
Received
24th August 2012
, Accepted 26th October 2012
First published on 30th October 2012
Abstract
Iron is known to be indispensable for life since its levels are related to biochemical, pharmacological and toxicological functions in the organisms. Herein, we demonstrate a very simple visual detection method of iron(II) cations (Fe2+) with high specificity based on its etching effects on the blue fluorescent ribbon aggregates of 4′,4′′′′-(1,4-phenylene) bis(2,2′:6′,2′′-terpyridine) (PBTPy). PBTPy, a terpyridine attached molecule, is observed to form blue fluorescent ribbon aggregates in a pH-modulated solvent of DMSO/H2O. Owing to the complexing of two terpyridine functional groups with Fe2+, the aggregates of PBTPy are gradually “etched” by Fe2+, giving new absorption bands at around 576 nm, 582 nm and 610 nm with a color change from purple to blue and then to cyan, depending on the content of Fe2+. This response is highly specific for Fe2+ over many other metal ions, including Fe3+, and thus successfully applied to the detection of Fe2+ released from the ferritin.
1. Introduction
Elemental iron is considered virtually indispensable for life, and is in both ferrous (Fe2+) and ferric (Fe3+) oxidation states for the purpose of different biological functions. Many biochemical processes at the cellular level, such as oxygen transportation and DNA synthesis, are involved in electron transfer between the two oxidation states of iron.1 On the other hand, the levels of iron are related to some biochemical, pharmacological, and toxicological functions in biological systems, and either iron deficiency or overload can lead to human disorders and diseases.1–6 Therefore, great efforts have been taken to develop detection methods for iron, including absorption and color,7–11 electrochemistry,12–15 magnetic resonance imagining and magnetic susceptometry,16 high-performance liquid chromatography,17 and fluorescence.18–26 We noticed that most of the methods focused on sensing the content of Fe3+ or the total of Fe2+ and Fe3+, and there were only a few examples for the selective detection of Fe2+.9,11–12,20 Although an inorganic/organic hybrid has been developed for the highly selective fluorescence “turn-on” sensing of Fe2+, the selectivity against Fe3+ was absent.20 In this work, we developed a very simple method for the highly selective detection of Fe2+ based on its etching effects on the ribbon aggregates, which could be visually observed with the naked eye.
It is known that organic molecules can form aggregates through hydrogen bonds, π–π type interactions, van der Waals forces, hydrophobic effects and so on,27–29 and aggregates of organic molecules have received great attention owing to their potential applications in light emitting diodes, electrochemical cells, and sensors.30,31 In sensing chemistry, sensing units usually contain recognition sites for a specific analyte. In our opinion, aggregates of organic molecules, which have effective recognition sites, such as terpyridine, might be capable of complexing with transition-metal ions through metal ion–nitrogen bonds, either generating metallo-supramolecular assemblies32–39 or being etched.40 Herein, we introduce a terpyridine attached molecule, 4′,4′′′′-(1,4-phenylene)bis(2,2′:6′,2′′-terpyridine) (PBTPy), which can form blue fluorescent aggregates in the form of ribbons in the mixture of water and dimethylsulfoxide (DMSO), and the ribbon aggregates could be gradually “etched” by Fe2+, giving new absorption bands and showing different colors from purple to blue and then to cyan depending on the content of Fe2+. The absorption and color responses are highly selective for Fe2+ over many other metal cations, including Fe3+. Thus, highly specific visual detection of Fe2+ is achieved.
2. Experimental
2.1 Chemicals and materials
4′,4′′′′-(1,4-Phenylene)bis(2,2′:6′,2′′-terpyridine) (PBTPy) was purchased from Sigma (St. Louis, MO), and its stock solution of 5.0 × 10−4 mol L−1 was prepared by dispersing the commercial solid PBTPy in dimethylsulfoxide (DMSO). Ferritin from equine spleen, Type I, saline solution (44 mg mL−1) was purchased from Sigma (St. Louis, MO). FeSO4·7H2O was purchased from Chongqing Inorganic Chemical Reagents Plant (Chongqing, China), and the stock solution of 5.0 × 10−3 mol L−1 kept the pH values at 2–3 by dissolving FeSO4·7H2O into water. Other metal cation reagents were all purchased from China. All chemicals were analytical reagents and were used without further purification. Milli-Q purified water (18.2 MΩ) was used throughout the experiment. All results were obtained in a solvent of 1
:
9 DMSO/H2O (V/V).
2.2 Instrumentation
The UV-vis spectra were recorded with a Hitachi U-3010 spectrophotometer (Tokyo, Japan). The fluorescence spectra were measured on an F-4600 spectrophotometer. The scanning electron microscope (SEM) images were performed with a Hitachi S-4800 scanning electron microscope (Tokyo, Japan), and the dynamic light scattering analysis was completed on a Zetasizer Nano-ZS System (Malvern Inc.). The fluorescence lifetime measurements were performed using a TCSPC Fluorolog (HORIBA JOBINYVON) with a LED centered at 370 nm as the irradiation source. An Olympus IX81LCS-DSU Disk Scanning confocal microscope (Olympus, Japan) was used for the cell imaging. The running software was Invivo 3.2/3D Analyzer 6.2. The image analysis and processing software was Image-Pro® Plus Version 6.3 for WindowsTM. AXIMA Performance was used to determine the mass of the metal complexes. The TAS-986 atomic absorption spectrophotometer (PGENERAL) was used to determine the amounts of iron released from the ferritin. A vortex mixer QL-901 (Haimen, China) was used to blend the solution.
2.3 Release of Fe2+ from Ferritin9
Ferritin from equine spleen, Type I (4.4 mg mL−1) in a solution of NaCl (15.0 mmol L−1) was incubated with 4.0 mmol L−1 ascorbic acid to release the iron and to reduce Fe(III) to Fe(II) at room temperature for 12 h. After that, the obtained mixture containing Fe(II) was deproteinized through a Microcon Ultracel YM-10, regenerated cellulose 10
000 MWCO (Millipore Corp., Bedford, MA). The filtrates were collected to determine the amounts of Fe(II).
2.4 Procedures of PBTPy coordinated with metal ions
150.0 μL of the aqueous solution of 0.1 mol L−1 HCl or pH 7.2 tris-HCl was mixed with 50.0 μL of the DMSO solution of PBTPy in a 1.5 mL plastic tube. Then an appropriate volume of metal ions was added. Finally, the mixture was diluted to 500 μL with doubly distilled water and blended thoroughly. Then the mixture was transferred to a quartz cell for measurements.
3. Results and discussion
3.1 Formation of blue fluorescent ribbons
PBTPy can be dispersed in the mixture of DMSO/H2O, displaying wide hump absorption bands in the wavelength region of less than 600 nm (the black curve in Fig. 1A) and giving two fluorescence emission bands centered at 358 nm and 565 nm (Fig. 1B), in both neutral and alkaline conditions (Fig. S1†, supplementary material). The wide hump absorption bands, as show in Fig. 1A, are usually observed in aggregates of organic molecules,31 and SEM images in Fig. 1C and 1D can identify that aggregates of PBTPy really have formed. In acidic conditions, however, the wide hump absorption bands gradually sharpened at 292 nm and disappeared in the region of 360–600 nm (Fig. 1A), simultaneously giving a new fluorescence emission band centered at around 430 nm when excited at 306 nm with the disappearance of the two emission bands at 358 nm and 565 nm (Fig. 1B). Further decreasing the pH values, the fluorescence and absorption spectra remain almost unchanged (Fig. S2†, supplementary material). Very interestingly, SEM and fluorescence images in Fig. 1E and 1F showed that the irregular particles formed in neutral or alkaline conditions have reassembled in the acidic medium, forming blue fluorescent ribbons.
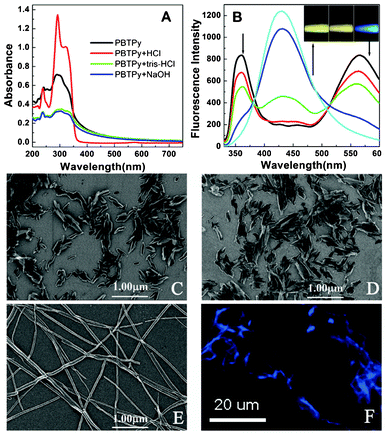 |
| Fig. 1 Absorption and fluorescence spectra as well as SEM and fluorescence images of PBTPy. (A) UV-visible absorption spectra of PBTPy dispersed in the mixture of DMSO/H2O and in the media of the buffer of pH 7.2 tris-HCl, the solutions of 0.03 mol L−1 NaOH and 0.03 mol L−1 HCl, respectively. (B) Fluorescence emission of PBTPy when excited at 306 nm. The arrows indicate that the added HCl was from 0 to 0.03 mol L−1 in turn. (C–E) SEM images of PBTPy aggregates in pH 7.2 tris-HCl (C) and in 0.03 mol L−1 NaOH (D) as well as in 0.03 mol L−1 HCl (E). (F) The fluorescence image of PBTPy aggregates in 0.03 mol L−1 HCl. Inset picture in (B) shows the color of the fluorescence emission corresponding to C–E using the 306 nm light as excitation source on the F-4600 fluorescence spectrophotometer. cPBTPy, 5.0 × 10−5 mol L−1. | |
Meanwhile, we performed a theoretical computation to gain an insight into the state of PBTPy depending on the acidity. The result shows that PBTPy monomer is not a big planar structure in the neutral condition (Fig. S3A†, supplementary material). But owing to the phenyl ring and six pyridine rings, the π–π stacking still exists. While in the acidic conditions, the nitrogen atom in terpyridine is protonated, and consequently, intramolecular hydrogen bonds between the proton and the lone pairs of the nitrogen atoms in pyridine are formed, leading to the increasing planarity of PBTPy, especially the two terpyridine groups (Fig. S3B†, supplementary material).32 In such a case, the intermolecular π–π stacking interactions become stronger, which is a possible explanation for the formation of long ribbon aggregates.
3.2 Binding of the PBTPy aggregates with Fe2+
Upon addition of metal ions, the fluorescence of the ribbon aggregates will be changed in different ways owing to the coordination ability of the functional group of terpyridine with metal ions. However, there is no specific fluorescent response of the PBTPy aggregates to a certain metal ion (Fig. S4†, supplementary material). For example, Zn2+, Cd2+ and Hg2+ can induce a redshift of the emission wavelength (Fig. S4A–C†). Fe2+, Co2+, Ni2+, and Cu2+ will all quench the blue fluorescence of the PBTPy aggregates (Fig. S4D–G†). Whereas Mg2+, Ca2+, Mn2+, Cr3+, and Pb2+ have negligible effects on the fluorescence of the ribbon aggregates (Fig. S4H–L†). So, the fluorescent characteristics are unfavorable to the sensing of metal ions because of the poor specificity.
A further interesting observation is that the formed blue fluorescent ribbons of the aggregates are colorless (Fig. 2A), but display a series of colors upon the addition of Fe2+. In the presence of Fe2+, the absorption band at 292 nm of the aggregates decreased with the simultaneous increase of the one at 322 nm, accompanied by the appearance of two absorption bands at 376 nm and 572 nm. A noticeable red shift to 576 nm was observed for the latter absorption band (Fig. S5A†, supplementary material), which is a metal–ligand charge transfer (MLCT) band and is responsible for the purple color (Fig. 2B).11,32 With further addition of Fe2+, a further bathochromic shift of the purple color-related absorption band to 582 nm was observed, resulting in a blue solution (Fig. 2C). If the concentration of Fe2+ is above 1 equiv. of PBTPy, the absorption of PBTPy at 322 nm began to shift to 326 nm with the appearance of two new absorption bands at 396 nm and 610 nm, relative to those at 376 nm and 582 nm, which was the most obvious change. Meanwhile, the color of the solution changed from blue to cyan (Fig. 2D and Fig. S5†, supplementary material).
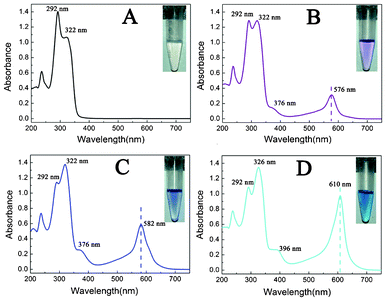 |
| Fig. 2 UV-vis absorption spectra and the observed color changes of PBTPy (A) upon titration with Fe2+ (B–D) in 0.03 mol L−1 HCl. The concentration ratios of Fe2+ to PBTPy from B–D are 0.5 : 1, 1 : 1, and 2 : 1 in turn. cPBTPy, 5.0 × 10−5 mol L−1. | |
3.3 Interaction mechanism between the PBTPy aggregates and Fe2+
On the basis of the above gradual changes of the absorption bands and colors, the mass spectra were obtained in order to understand the binding process between PBTPy and Fe2+. The mass peak at around m/z = 540.6 is assigned to the molecule of PBTPy. Whether the concentration ratio of Fe2+/PBTPy is 1
:
2, or 1
:
1, or 2
:
1, the mass spectra display the same mass peak at m/z = 596.6, which is ascribed to the formation of a 1
:
1 complex of PBTPy-Fe2+ (Fig. S6†, supplementary material). However, the mass of the 1
:
2 complex of PBTPy-Fe2+-PBTPy and the 2
:
1 complex of Fe2+-PBTPy-Fe2+ cannot be detected, most likely because the stability of the two Fe2+ complexes is not as good as that of the PBTPy-Fe2+ complex.
Dynamic light scattering (DLS) analysis, which is effective to measure shape changes, such as for gold nanorods,41 showed that the PBTPy aggregates in the acidic medium have an average hydrodynamic diameter of 101.3 ± 1.62 nm. Upon titration with Fe2+, however, the hydrodynamic diameter of the aggregates gets smaller (Fig. 3A), and the ribbon assemblies are gradually “etched” by Fe2+ as shown in Fig. 3B-D. When the concentration of Fe2+ gets increased to 2 equiv of PBTPy, nothing could be observed from the SEM images, and the emission of the PBTPy aggregates at 430 nm gets quenched (Fig. S4D†, supplementary material). The fluorescence decay curves are also performed (Fig. S7†, supplementary material) and the fitted fluorescence lifetimes (Table S1, supplementary material†) show that the percentages of the long lifetimes are increased and the percentage of the shortest component is gradually decreased with the increase of the concentration of Fe2+. In this work, the shortest lifetime can be ascribed to the PBTPy aggregates and the decrease in the percentage of the shortest component indicates that the amounts of the aggregates are decreased. The increase in the percentages of long components suggests the gradual complexation of PBTPy with Fe2+. So, we think that the complexation of PBTPy with Fe2+ destroys the noncovalent interactions of PBTPy molecules and leads to the aggregates falling apart.
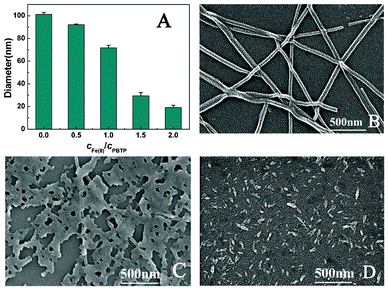 |
| Fig. 3 Changes in the hydrodynamic diameter and the shape of PBTPy aggregates. (A) The hydrodynamic diameters of PBTPy aggregates in the presence of Fe2+, and (B–D) SEM images of PBTPy aggregates in the (B) absence and (C, D) presence of Fe2+ in the medium of 0.03 mol L−1 HCl. The concentration ratios of Fe2+ to PBTPy are 0.5 : 1 and 1 : 1 of C and D in turn. cPBTPy, 5.0 × 10−5 mol L−1. | |
3.4 The response feature of the PBTPy aggregates for sensing of Fe2+
It is observed that in neutral and alkaline conditions, the complexation between PBTPy and Fe2+ is a slower process than that in the acidic conditions. Even if PBTPy and Fe2+ have been mixed together for three hours, the absorption spectrum and the color of the solution still change a little (Fig. S8†, supplementary material). However, in the acidic conditions, the absorption band in the wavelength region from 450–700 nm emerges after the addition of Fe2+, which is a dynamic process and can reach the maximum within 30 min (Fig. S9†, supplementary material). Generally, the protonated terpyridine was unfavourable to bind metal ions because the protons will compete with metal ions in the complexation. However, it seems that the aggregates of PBTPy in acidic conditions complex more easily with Fe2+, which is inconsistent with the general viewpoint. It is most likely that the aggregation modes of PBTPy molecules have an important effect on the complexation with Fe2+ whether the PBTPy molecules are protonated or not. Considering the fact that the response speed is an important factor of an analytical method, the aggregates of PBTPy in an acidic condition are more suitable for sensing of Fe2+.
3.5 Specific response of the PBTPy aggregates to Fe2+
In order to define the specific response of PBTPy to Fe2+, complexation experiments with some other common metal cations were performed. When an equimolar amount of a certain metal cation except Fe2+ was added to the solution of PBTPy, no distinct absorption band centered at 576 nm appeared, and there were only changes of the absorption bands at 292 nm and 322 nm (Fig. S10†, supplementary material). Even if the concentration ratio of the metal cations to PBTPy such as Zn2+, Cd2+, Hg2+, Cu2+, and Co2+ was up to 3, they still induced no responses of PBTPy near 576 nm (Fig. S11†, supplementary material). Whereas in the presence of Fe2+, the solution of PBTPy displayed strong absorption at 576 nm resulting in a purple solution, demonstrating the high selectivity of PBTPy for Fe2+ as shown in Fig. 4. Also, the competition experiment of PBTPy toward Fe2+ in the presence of the common metal cations was carried out. The results in Fig. 5 showed that these coexistent cations hardly affected the detection of Fe2+.
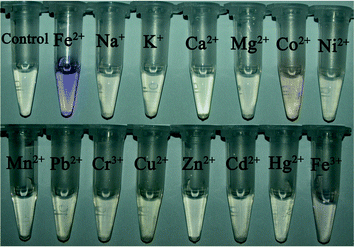 |
| Fig. 4 The color changes of PBTPy in the presence of different metal ions (5.0 × 10−5 mol L−1). cPBTPy, 5.0 × 10−5 mol L−1; cFe2+, 2.5 × 10−5 mol L−1. | |
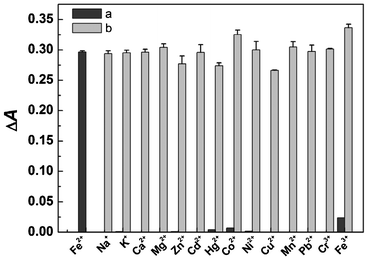 |
| Fig. 5 The binding of PBTPy aggregates with metal ions. (a) The absorbance changes of PBTPy aggregates at 576 nm upon the addition of Fe2+ and other metal ions (5.0 × 10−5 mol L−1) and (b) the competitive responses of PBTPy toward Fe2+ in the presence of the other metal ions. The other metal ions are all 5.0 × 10−5 mol L−1 except that Cu2+ and Hg2+ are 1.25 × 10−5 mol L−1. cPBTPy, 5.0 × 10−5 mol L−1, cFe2+, 2.5 × 10−5 mol L−1. | |
3.6 Selective visual detection of Fe2+
Upon titration with Fe2+, the increased absorbance (ΔA) at 576 nm of the PBTPy aggregates was linearly correlated with the concentration of Fe2+ in the range 2.5–37.5 μmol L−1 (r = 0.9999, from three measurements) (Fig. S5A†, supplementary material). Furthermore, this method has been successfully applied to the detection of Fe2+ released from the ferritin. Ferritin is a universal iron storage protein with a shell of organized apoferritin and a core of ferric oxyhydroxide and plays an important role in iron metabolism within cells. The iron deposition in ferritin usually begins with the uptake of Fe2+ into the protein shell, and the abnormal release of iron from ferritin is associated with some diseases.1 By releasing iron from the ferritin with ascorbic acid as a reducing agent and obtaining the solution of Fe2+ after a deproteinization process,9 we can directly determine the amount of Fe2+ with the value of 11.0 ± 0.2 μmol L−1, which was almost consistent with the value of 10.0 μmol L−1 determined from atomic absorption spectrometry.
4. Conclusions
In conclusion, we studied the aggregation behavior of 4′,4′′′′-(1,4-phenylene)bis(2,2′:6′,2′′-terpyridine) (PBTPy) under different conditions. On the basis of the ribbon aggregates of PBTPy in acidic conditions, we established a simple detection method for Fe2+. This method shows remarkably good selectivity for Fe2+ among other common metal ions, including Fe3+, even in the presence of these metal cations, and the sensing process can be observed with the naked eye through stepwise color changes from purple to blue and then to cyan. Also, it can be employed for the detection of Fe2+ released from ferritin. We hope that this work can promote the application of the aggregates of organic molecules in sensing through attaching appropriate recognition sites.
Acknowledgements
The authors are grateful to the National Natural Science Foundation of China (NSFC, No. 21175109) and the special fund of Chongqing key laboratory (CSTC) for the financial support.
References
-
R. Crichton, Inorganic Biochemistry of Iron Metabolism: From Molecular Mechanisms to Clinical Consequences, John Wiley & Sons Ltd, Chichester, 2nd edn, 2001 Search PubMed.
- J. H. Martin and S. E. Fitzwater, Nature, 1988, 331, 341–343 CrossRef CAS.
- M. J. Behrenfeld, A. J. Bale, Z. S. Kolber, J. Aiken and P. G. Falkowski, Nature, 1996, 383, 508–511 CrossRef CAS.
- M. A. Smith, P. L. R. Harris, L. M. Sayre and G. Perry, Proc. Natl. Acad. Sci. U. S. A., 1997, 94, 9866–9868 CrossRef CAS.
- X. Liu and E. C. Theil, Acc. Chem. Res., 2005, 38, 167–175 CrossRef CAS.
- F. A. Tezcan, J. T. Kaiser, D. Mustafi, M. Y. Walton, J. B. Howard and D. C. Rees, Science, 2005, 309, 1377–1380 CrossRef CAS.
- J. F. Wm. Cagle and G. F. Smith, Anal. Chem., 1947, 19, 384–385 CrossRef.
- K. J. Wallace, M. Gray, Z. Zhong, V. M. Lynch and E. V. Anslyn, Dalton Trans., 2005, 2436–2441 RSC.
- Y.-F. Huang, Y.-W. Lin and H.-T. Chang, Langmuir, 2007, 23, 12777–12781 CrossRef CAS.
- T. Gupta and M. E. v. d. Boom, J. Am. Chem. Soc., 2007, 129, 12296–12303 CrossRef CAS.
- C. Bhaumik, S. Das, D. Maity and S. Baitalik, Dalton Trans., 2011, 40, 11795–11808 RSC.
- J. N. Richardson and A. L. Dyer, Anal. Chem., 2002, 74, 3330–3335 CrossRef CAS.
- K. Suganandam, P. Santhosh, M. Sankarasubramanian, A. Gopalan, T. Vasudevan and K.-P. Lee, Sens. Actuators, B, 2005, 105, 223–231 CrossRef.
- C. M. Wansapura, C. J. Seliskar and W. R. Heineman, Anal. Chem., 2007, 79, 5594–5600 CrossRef CAS.
- M. Stoytcheva, R. Zlatev, J.-P. Magnin, M. Ovalle and B. Valdez, Biosens. Bioelectron., 2009, 25, 482–487 CrossRef CAS.
- G. M. Brittenham and D. G. Badman, Blood, 2003, 101, 15–19 CrossRef CAS.
- H. Matsumiya, N. Iki and S. Miyano, Talanta, 2004, 62, 337–342 CrossRef CAS.
- B. P. Espósito, S. Epsztejn, W. Breuer and Z. I. Cabantchik, Anal. Biochem., 2002, 304, 1–18 CrossRef.
- J. L. Bricks, A. Kovalchuk, C. Trieflinger, M. Nofz, M. Büschel, A. I. Tolmachev, J. Daub and K. Rurack, J. Am. Chem. Soc., 2005, 127, 13522–13529 CrossRef CAS.
- L.-J. Fan and J. Wayne E. Jones, J. Am. Chem. Soc., 2006, 128, 6784–6785 CrossRef CAS.
- Y. Xiang and A. Tong, Org. Lett., 2006, 8, 1549–1552 CrossRef CAS.
- R. Kikkeri, H. Traboulsi, N. Humbert, E. Gumienna-Kontecka, R. Arad-Yellin, G. Melman, M. Elhabiri, A.-M. Albrecht-Gary and A. Shanzer, Inorg. Chem., 2007, 46, 2485–2497 CrossRef CAS.
- N. C. Lim, S. V. Pavlova and C. Brückner, Inorg. Chem., 2009, 48, 1173–1182 CrossRef CAS.
- A. J. Weerasinghe, C. Schmiesing, S. Varaganti, G. Ramakrishna and E. Sinn, J. Phys. Chem. B, 2010, 114, 9413–9419 CrossRef CAS.
- X. Wu, B. Xu, H. Tong and L. Wang, Macromolecules, 2010, 43, 8917–8923 CrossRef CAS.
- M. Kumar, R. Kumar and V. Bhalla, Org. Lett., 2011, 13, 366–369 CrossRef CAS.
- S. Lahiri, J. L. Thompson and J. S. Moore, J. Am. Chem. Soc., 2000, 122, 11315–11319 CrossRef CAS.
- C. Z. Huang, K. A. Li and S. Y. Tong, Anal. Chem., 1996, 68, 2259–2263 CrossRef CAS.
- C. Z. Huang, Y. F. Li and P. Feng, Anal. Chim. Acta, 2001, 443, 73–80 CrossRef CAS.
- G. Yu, S. Yin, Y. Liu, J. Chen, X. Xu, X. Sun, D. Ma, X. Zhan, Q. Peng, Z. Shuai, B. Tang, D. Zhu, W. Fang and Y. Luo, J. Am. Chem. Soc., 2005, 127, 6335–6346 CrossRef CAS.
- W. Tang, Y. Xiang and A. Tong, J. Org. Chem., 2009, 74, 2163–2166 CrossRef CAS.
-
R. Dobrawa, P. Ballester, C. R. Saha-Möller and F. Würthne, in Metal-Containing and Metallosupramolecular Polymers and Materials, ed. U. S. Schubert, G. R. Newkome and I. Manners, Washington, 2006, vol. 928, pp. 43–62 Search PubMed.
-
P. R. Andres, H. Hofmeier and U. S. Schuber, in Metal-Containing and Metallosupramolecular Polymers and Materials, ed. U. S. Schubert, G. R. Newkome and I. Manners, Washington, 2006, vol. 928, pp. 141–156 Search PubMed.
- U. S. Schubert, C. Eschbaumer, P. Andres, H. Hofmeier, C. H. Weidl, E. Herdtweck, E. Dulkeith, A. Morteani, N. E. Hecker and J. Feldmann, Synth. Met., 2001, 121, 1249–1252 CrossRef CAS.
- F. Barigelletti, L. Flamigni, G. Calogero, L. Hammarström, J.-P. Sauvage and J.-P. Collin, Chem. Commun., 1998, 2333–2334 RSC.
- R. Dobrawa and F. Würthner, Chem. Commun., 2002, 1878–1879 RSC.
- C. Goze, G. Ulrich, L. Charbonnière, M. Cesario, T. Prangé and R. Ziessel, Chem.–Eur. J., 2003, 9, 3748–3755 CrossRef CAS.
- N. Tuccitto, I. Delfanti, V. Torrisi, F. Scandola, C. Chiorboli, V. Stepanenko, F. Würthner and A. Licciardello, Phys. Chem. Chem. Phys., 2009, 11, 4033–4038 RSC.
-
U. S. Schubert, A. Winter and G. R. Newkome,Terpyridine-based Materials: For Catalytic, Optoelectronic and Life Science Applications, Wiley-VCH Verlag &Co. KGaA, Boschstr., Weinheim, Germany, 2011 Search PubMed.
- S. J. Zhen, F. L. Guo, L. Q. Chen, Y. F. Li, Q. Zhang and C. Z. Huang, Chem. Commun., 2011, 47, 2562–2564 RSC.
- B. Pan, D. Cui, P. Xu, Q. Li, T. Huang, R. He and F. Gao, Colloids Surf., A, 2007, 295, 217–222 CrossRef CAS.
Footnote |
† Electronic Supplementary Information (ESI) available: Additional figures (Fig. S1–S11) and Table S1. See DOI: 10.1039/c2ra21918b |
|
This journal is © The Royal Society of Chemistry 2013 |
Click here to see how this site uses Cookies. View our privacy policy here.