Electrospun photosensitive nanofibers: potential for photocurrent therapy in skin regeneration†
Received
20th March 2012
, Accepted 29th June 2012
First published on 3rd July 2012
Abstract
Poly(3-hexylthiophene) (P3HT) is one of the most promising photovoltaic (PV) polymers in photocurrent therapy. A novel photosensitive scaffold for skin tissue engineering was fabricated by blending P3HT with polycaprolactone (PCL) and electrospun to obtain composite PCL/P3HT nanofibers with three different weight ratios of PCL
:
P3HT (w/w) of 150
:
2 [PCL/P3HT(2)], 150
:
10 [PCL/P3HT(10)] and 150
:
20 [PCL/P3HT(20)]. The photosensitive properties of the blend solutions and the composite nanofibers of PCL/P3HT were investigated. The incident photon-to-electron conversion efficiencies of the PCL/P3HT(2), PCL/P3HT(10), PCL/P3HT(20) were identified as 2.0 × 10−6, 1.6 × 10−5 and 2.9 × 10−5, respectively, which confirm the photosensitive ability of the P3HT-containing scaffolds. The biocompatibility of the scaffold was evaluated by culturing human dermal fibroblasts and the results showed that the proliferation of HDFs under light stimulation on PCL/P3HT(10) was 12.8%, 11.9%, and 11.6% (p ≤ 0.05) higher than the cell growth on PCL, PCL/P3HT(2) and PCL/P3HT(20), respectively. Human dermal fibroblasts cultured under light stimulation on PCL/P3HT(10) not only showed better cell proliferation but also retained cell morphology similar to the phenotype observed on tissue culture plates (control). Our experimental results suggest novel and potential application of an optimized amount of P3HT-containing scaffold, especially PCL/P3HT(10) nanofibrous scaffold in photocurrent therapy for skin regeneration.
1. Introduction
Human skin acts as an anatomical barrier from pathogens and damage between the internal and external environment in bodily defense and it plays a key role in protecting the body against excessive water loss.1,2 However, skin wounds such as burn injuries cause millions of patients to remain disabled and require hospitalization. Globally, over 6 million patients suffer from severe burns each year among which more than 300
000 persons die.3,4 Several bioengineered grafts including autografts and xenografts have been employed to cure skin wounds, and autografts have been regarded as the gold standard for treating full-thickness wounds. However, problems associated with limited donor sites restrict the application of autografts.5 Skin tissue engineering (TE) involves the use of scaffolds that support cell attachment and proliferation, together with the formation of a skin tissue-like structure to substitute the skin.6 Photocurrent therapy with participation of light and electrical stimulation could be an innovative and promising approach in skin regenerative medicine.7 The effects of electrical and photic signals on cell behaviors have been studied for a long time and the main signals used for electrical stimulation (ES) are DC electrical signals, pulsed cathodal electrical signals and electromagnetic fields. DC electrical signal could be a directional cue that plays a significant role in the spatial organization of the vascular structure.8 A pulsed cathodal ES used by Feedar et al. for chronic dermal ulcer healing showed that pulsed ES has a beneficial effect on healing chronic dermal ulcers.9 Three types of electromagnetic fields (inductive coupling, capacitive coupling, and combined electromagnetic fields) were applied by Brighton et al. to stimulate bone cells. These electromagnetic fields produced a significant increase in DNA content compared to the controls without electromagnetic field stimulation.10 The long-term ES of osteoblasts is also known to alter the pattern of gene expression resulting in enhanced extracellular matrix (ECM) synthesis.11 Concurrently, phototherapy is the therapy in the presence of light irradiation and clinical application of phototherapy or the therapeutic applications of light has been increased over recent years. Infrared (700–1200 nm) and near-infrared (600–700 nm) light delivered through light-emitting diodes or lasers have been reported to significantly accelerate the repair of chronic skin wounds.12 Phototherapy can reduce inflammatory reactions, increase the production of basic fibroblast growth factor, enhance cell proliferation and accelerate cutaneous wound healing.13 With electrotherapy and phototherapy having positive effects on regenerative medicine, it is also thought that photocurrent (the current generated under light stimulation) could be a promising method for application in regenerative medicine.
Aiming to prepare a suitable scaffold for photocurrent therapy in skin TE, we fabricated photosensitive nanofibrous scaffolds by electrospinning. Electrospinning is capable of producing continuous fibers with diameters down to nanometers to mimic the fiber size and orientation of in vivo extracellular matrix (ECM).14 Poly(3-hexylthiophene) (P3HT) is one of the most promising photovoltaic polymers and it shows great promise for a range of applications, including organic LEDs and photocurrent therapy.15 It is used in polymer-based photovoltaic cells mainly because P3HT possesses a high absorption coefficient close to the maximum photon flux in the solar spectrum and high charge-carrier mobility.16 However, electrospinning of pure P3HT, though attempted, is not possible mainly due to the absence of chain entanglement.15,17 Polycaprolactone (PCL) is a bio-resorbable and biocompatible polymer, and it has been studied as a wound dressing material since the 1970s. Extensive research has been conducted on its biocompatibility and efficacy, both in vitro and in vivo, resulting in FDA approval of a number of medical and drug-delivery devices composed of PCL.18 Polymer blending is one of the most effective methods for preparing desirable biocomposite scaffolds for particular applications,19 and during this study we blended PCL with P3HT to fabricate PCL/P3HT nanofibers via electrospinning. The chemical, mechanical and photovoltaic characteristics of the electrospun nanofibers, together with its cell biocompatibility evaluations were carried out. Our study results demonstrated that the PCL/P3HT(10) could be a promising scaffold for tissue engineering, especially in photocurrent-induced skin tissue regeneration.
2. Materials and methods
2.1. Materials
PCL (Mw 80
000), P3HT (Mw 45
000–65
000, Aldrich 99.995%), chloroform, methanol, dimethyl sulfoxide (DMSO) and phalloidin (Fluorescein Isothiocyanate Labeled) were all obtained from Sigma-Aldrich Pte Ltd, Singapore and used as received. Dulbecco's Modified Eagle's Medium (DMEM), fetal bovine serum (FBS), penicillin-streptomycin solution and 4,6-diamidino-2-phenylindole, dihydrochloride (DAPI) were purchased from Invitrogen Corporation, USA.
PCL and P3HT with three different weight ratios (150
:
2, 150
:
10 and 150
:
20) were dissolved in chloroform–methanol–DMSO (75
:
20
:
5 v/v) to obtain 9% (w/v) solutions. The polymer solutions were fed into a 3 ml standard syringe attached to a 27G blunted stainless steel needle using a syringe pump (KDS 100, KD Scientific, Holliston, MA) at a flow rate of 1.0 ml h−1. A high voltage of 15 kV (Gamma High Voltage Research, USA) was applied when the polymer solution was drawn into fibers and collected on an aluminum foil-wrapped collector kept at a distance of 12 cm from the needle tip. Nanofibers collected on 15 mm cover slips and aluminum foil were dried overnight under vacuum and used for the characterization and cell culture experiments.
Hereafter, in this manuscript P3HT-containing PCL nanofibers with three different weight ratios 150
:
2, 150
:
10 and 150
:
20 will be represented as PCL/P3HT(2), PCL/P3HT(10) and PCL/P3HT(20), respectively.
2.3. Characterization
UV-Vis spectra of the polymer solutions were measured using a Shimadzu UV-3600 UV-Vis-NIR spectrophotometer after dispersing the materials in chloroform and methanol (75
:
25 v/v). The morphology of the electrospun nanofibers was studied under Field Emission Scanning Electron Microscope (FEI-QUANTA 200F, Netherlands) at an accelerating voltage of 15 kV, after sputter coating with gold (JEOL JFC-1200 fine coater, Japan). Diameters of the electrospun fibers were analyzed from the SEM images using image analysis software (Image J, National Institutes of Health, USA). A CFP-1200-A capillary flow porometer (PMI, New York, NY) was used in this study to measure the pore size and distribution. Calwick with a defined surface tension of 15.9 dynes cm−1 (PMI, New York, NY) was used as the wetting agent for porometry measurements. Nanofibers were cut into 3 cm × 3 cm squares for porometry measurement. Nanofibers with similar thickness were obtained and each sample was tested individually. The J–V characteristics of the different PCL/P3HT composite nanofibers were recorded under inert atmosphere and at room temperature using a calibrated San-Ei XES-151 S (Japan) class A solar simulator under standard conditions (1 Sun, 1.5 G). Before the test, the FTO plate having the photosensitive nanofiber was pressed against an aluminium (Al)-sputtered FTO using binder clips and connected to the stimulator with wires. Photoluminescence (PL) spectra were recorded using the photonic multichannel spectral analyzer (Hamamatsu Photonics K.K., PMA 11) and the wavelength of the excitation beam was set between 380 and 420 nm.
2.4. Human dermal fibroblast culture
Human dermal fibroblasts (HDFs) used for this study was purchased from American Type Culture Collection, USA. HDFs were cultured in DMEM supplemented with 10% FBS and 1% antibiotic and antimycotic solutions (termed as normal growth media) in a 75 cm2 cell culture flask. Cells were incubated at 37 °C in a humidified atmosphere containing 5% CO2 for 6 days and the culture medium was changed once every 3 days. The 15 mm cover slips with electrospun nanofibers were placed in 24 well plate and pressed with a stainless steel ring to ensure complete contact of the scaffolds with the wells. The specimens were sterilized under UV light, washed thrice with phosphate-buffered saline (PBS) and subsequently incubated in DMEM overnight before cell seeding. HDFs were grown to confluency, detached by trypsin, counted by trypan blue assay using a hemocytometer and seeded on the scaffolds at a density of 10
000 cells per well.
2.5 Light stimulation
To study the effects of different amounts of P3HT incorporated in PCL nanofibrous scaffolds on fibroblast proliferation under light stimulation, illumination of human dermal fibroblasts on PCL, PCL/P3HT(2), PCL/P3HT(10) and PCL/P3HT(20) was performed using white light-emitting diodes (LEDs) (DIODER Lighting strip, IKEA, Singapore). The calibration of LEDs was performed with a USB 2000 spectrometer (Ocean optics, FL, USA). The wavelength of white LED is 421 to 670 nm, within the range of visible light (390–750 nm). Fig. 1 shows the schematic explanation of light stimulation. After seeding cells, the 24-well plates containing cell-scaffold composites were directly located under LEDs. Light stimulation study was carried out for 6 days and fibroblasts on PCL, PCL/P3HT(2), PCL/P3HT(10) and PCL/P3HT(20) were irradiated for 1 h once every 24 h. After culturing the cells for a period of 6 days, cell proliferation was evaluated after light stimulation by colorimetric MTS assay (CellTiter 96 AQueous One solution, Promega, Madison, WI, USA). After culturing the cells for a period of 2, 4 and 6 days, cells were rinsed with PBS to remove unattached cells and incubated with 20% MTS reagent in serum free medium for a period of 3 h at 37 °C. Absorbance of the obtained dye was measured at 490 nm using a spectrophotometric plate reader (FLUOstar OPTIMA, BMG lab Technologies). The absorbance of the formazan dye is measured at 490 nm and the amount of formazan crystals formed is directly proportional to the number of live cells. As a control, cells were also cultured on the scaffolds under non-stimulative conditions to understand the specific effect of light stimulation towards cell proliferation after seeding cells on the same scaffolds.
 |
| Fig. 1 Schematic explanation of the method used for light irradiation on cell-scaffold constructs. PCL (white) and P3HT containing nanofibrous scaffolds (black) were put in 24-well plates and directly located under the LEDs. | |
2.6 F-actin staining
After carrying out light stimulation of cells seeded on the scaffolds for a period of 6 days, F-actin staining was carried out to observe the cell phenotype. For this, the cells were initially fixed with 5% paraformaldehyde (Sigma, Singapore) at room temperature for 15 min, washed and incubated with 2% BSA to block any unspecific binding. The cells were further incubated with phalloidin for 2 h at room temperature. Further, the cell-scaffold constructs were washed with PBS and incubated with propidium iodide (1
:
5000) for 30 min at room temperature for nuclear staining. Final washing of the constructs was done with PBS before mounting on a glass slide and visualized under laser scanning confocal microscopy (LSCM).
2.7 Expression of collagen
Sirius red staining method was used to analyze the presence of collagen in the cell matrix. It is a strong anionic dye whose sulfonic acid groups interact with the basic groups of collagen producing red stains. The intensity of red stains were used to determine the secretion of the collagen-containing ECM by fibroblasts after light stimulation. The cells were first fixed with 2.5% formaldehyde, stained with Sirius red stain consisting of 0.1% Sirius red F3B in a saturated aqueous solution of picric acid for 1 h. The cells were washed with mild acidified water followed by 100% ethanol and viewed under a Leica BM IRB microscope. Collagen is stained red on a yellow background in the nanofibrous scaffolds.
2.8 Statistical analysis
All the data presented are expressed as mean ± standard deviation (SD) of the mean. Each experiment was repeated three times. Statistical differences were determined by Student's two-sample t-test. Differences were considered statistically significant at p ≤ 0.05.
3. Results and discussion
Fig. 2 shows the UV-vis absorption spectra of PCL, PCL/P3HT(2), PCL/P3HT(10) and PCL/P3HT(20) in chloroform and methanol. No absorption peak was observed for PCL solution. Evidently, an increase in P3HT concentration resulted in an increase in the intensity of bands in the UV-vis spectra for PCL/P3HT(2), PCL/P3HT(10) and PCL/P3HT(20) solutions. The surface plasmon absorption bands for P3HT-containing PCL solutions were centered around 465 nm, which is characteristic of the π–π* transition.17 Moreover, the absorption wavelength of P3HT (465 nm) lies within the range of the visible light (390 to 750 nm). The most common visible light that we encounter daily in our life is the day light (sunlight) and half of its spectrum lies in the visible short-wave region of the electromagnetic spectrum. Human skin is the outer covering of the body and it is under frequent exposure of sunlight. Photocurrent therapy with PCL/P3HT nanofibers for skin TE could be very feasible if carried out under the stimulation of light (sunlight).
 |
| Fig. 2 UV-Visible absorption spectra of pure PCL, PCL/P3HT(2), PCL/P3HT(10), and PCL/P3HT(20) solutions dispersed in chloroform and methanol (75/25 v/v). The concentration of each solution was 9% (w/v). | |
3.2. Morphology and characterization
SEM micrographs of the electrospun nanofibrous scaffolds revealed uniform, beadless, nano-scaled fibrous structures under the optimized spinning conditions utilized during this study (Fig. 3). Uniform nanofibers of PCL, PCL/P3HT(2), PCL/P3HT(10) and PCL/P3HT(20) with fiber diameters in the range of 608 ± 168, 503 ± 116, 424 ± 128 and 314 ± 101 nm, were respectively obtained. The diameters of PCL/P3HT nanofibers decreased with the increase of P3HT concentration in PCL solutions. P3HT is a photosensitive and conductive polymer and including P3HT in PCL solution will improve the conductivity of the solution.17 The increase of P3HT concentration in PCL solution resulted in increased conductivity of the solutions and, under the same electrospinning conditions, the high conductivity of the P3HT-containing polymer solution favored the formation of fibers with smaller diameters.20,21 Hence the PCL/P3HT(20) had smaller fiber diameters compared to PCL/P3HT(10) and PCL/P3HT(2). The porosity of the electrospun scaffolds was studied by capillary flow porometer (CFP-1200-A) and the pore size of PCL, PCL/P3HT(2), PCL/P3HT(10) and PCL/P3HT(20) was obtained as 4.3 μm, 3.0 μm, 2.1 μm and 1.9 μm, respectively. Applied with low pressure, capillary flow porometry, provides a simple and non-destructive technique that allows rapid and accurate measurement of pore size and distribution.22,23 Therefore, capillary flow porometry can provide reproducible pore size and distribution measurements with ignorable distortion errors.24 However, fiber diameter and fiber mass plays a dominant role in controlling the pore diameter of the networks.24,25 In our study, the thickness of PCL, PCL/P3HT(2), PCL/P3HT(10) and PCL/P3HT(20) was kept identical (approximately 35 μm). The mean pore sizes obtained for the nanofibers were found inversely proportional to the diameter of the fibers and our results were also in accordance with the results reported by Li et al. These researchers found that, at a given network mass per unit area and porosity, an increasing fiber diameter results in an increase in mean pore size.25 Uniform distribution of pore sizes was observed for PCL, PCL/P3HT(2), PCL/P3HT(10) and PCL/P3HT(20) (Fig. 3).
 |
| Fig. 3 SEM images of electrospun (A) PCL/P3HT(2), (B) PCL/P3HT(10), (C) PCL/P3HT(20) and (D) PCL nanofibers and pore size distribution of (i) PCL/P3HT(2), (ii) PCL/P3HT(10), (iii) PCL/P3HT(20) and (iv) PCL nanofibers. | |
Photoluminescence (PL) is a process in which a substance absorbs photons and then re-radiates photons. PL spectra of electrospun nanofibers were collected to probe the electronic states of the P3HT present in the scaffolds. Fig. 4 shows the photoluminescence spectra of electrospun PCL, PCL/P3HT(2), PCL/P3HT(10) and PCL/P3HT(20) nanofibrous scaffolds. Spectra of every electrospun scaffold containing P3HT indicated the same maximum emission intensity at 699 nm. Chan et al. studied the structure of electrospun and cast P3HT membranes via PL spectroscopy and found the occurrence of blue shift in the PL spectra for electrospun P3HT membranes compared to cast film due to the presence of nano-voids in the electrospun fibers.26 In our study, no blue or red shifts were observed. A broader peak was observed in the emission spectrum of PCL/P3HT(20) compared to PCL/P3HT(10) than PCL/P3HT(2). The broadening of the peak can be correlated to the existence of a large distribution of P3HT within PCL nanofibers. The relative peak intensity of electrospun PCL/P3HT nanofibers was directly proportional to the amount of P3HT included in the PCL nanofibers. No emission intensity was however observed for electrospun PCL nanofibrous scaffolds. We therefore presumed that the P3HT was successfully conjugated with PCL within the nanofibers upon electrospinning.
 |
| Fig. 4 PL spectra for PCL, PCL/P3HT(2), PCL/P3HT(10) and PCL/P3HT(20) under excitation beam of 380–400 nm. | |
3.3 Photovoltaic study
P3HT is a conjugated material with a band gap of 1.9–2.0 eV.27 Upon illumination, certain electrons were generated due to the photosensitive properties of electrospun PCL/P3HT(2), PCL/P3HT(10) and PCL/P3HT(20), which was evaluated using a calibrated San-Ei XES-151 S (Japan) class A solar simulator. P3HT absorbs photons of light with appropriate wavelength (around 465 nm) and gets promoted to a higher energy level called the HOMO (highest occupied molecular orbital). The electrons getting to the HOMO level have high mobility in the delocalized orbitals of P3HT and they are free to move within a small space in P3HT chains based on the particle-in-a-box model, similar to the movement of a particle free to move in a small space surrounded by impenetrable barriers. When the photoinduced electrons move within the P3HT chains, an electrical current is formed. Initial measurements of the current density vs. voltage (J–V) of the PCL/P3HT(2), PCL/P3HT(10) and PCL/P3HT(20) were carried out under standard operating conditions (1 Sun, 1.5G). The ‘efficiency’ which determines the amount of radiated quantity of light that is converted into useable electrical energy obtained for the PCL/P3HT(2), PCL/P3HT(10) and PCL/P3HT(20) were 2.0 × 10−6, 1.6 × 10−5 and 2.9 × 10−5, respectively. The efficiency of photosensitive nanofibrous scaffolds was proportional to the amount of P3HT incorporated in the PCL nanofibers (Fig. 5A). The low efficiency of the photosensitive nanofibers can be explained due to the structure change of P3HT chains. The P3HT polymer chains exist in two resonant structures:28 aromatic (i) and quinoid (ii), as shown in Fig. 5B. When the polymer chains get more disordered, the aromatic structure becomes more favorable compared to the quinoid structure, because the adjacent rings in A are connected by a single bond making the chains more flexible, and this was inferred during our studies too when PCL was blended with P3HT polymer. This implies that even if the charge transfer occurs, the effect of disordering might overcome the effect of charge transfer29 and as a result fewer electrons might transit to the electron acceptor. Photocurrent was thus generated in the fiber, though of low intensity and this amount was sufficient to meet the essential requirement for application of electrospun photosensitive scaffolds in photocurrent therapy.
 |
| Fig. 5 (A) J–V graph of the PCL/P3HT(2), PCL/P3HT(10) and PCL/P3HT(20) photosensitive scaffolds measured under 1 Sun conditions. (B) The resonant structures, (i) aromatic and (ii) quinoid, of poly(3-alkylthiophenes) chains. | |
3.4 Light stimulation
The effect of light stimulation as well as the effect of different amounts of P3HT incorporated within the PCL scaffolds towards the proliferation of HDFs was evaluated by MTS assay (Fig. 6). In non-stimulated (NS) conditions, the proliferation of HDFs on PCL nanofibrous scaffold showed higher fibroblast proliferation compared to the proliferation of HDFs on PCL/P3HT(2), PCL/P3HT(10) and PCL/P3HT(20). Moreover, the proliferation of HDFs on PCL/P3HT(20) non-stimulated showed the lowest proliferation of cells due to higher amounts of P3HT present in PCL/P3HT(20). Hence, it was clear that the biocompatibility of P3HT-containing PCL naonfibrous scaffolds is not as good as pure PCL nanofibrous scaffold. However, the proliferation of HDFs under light stimulation (S) on PCL/P3HT(10) was significantly higher compared to the cell proliferation on other nanofibrous scaffolds. The ability of the photosensitive PCL/P3HT(10) in promoting the proliferation of HDFs was further confirmed after 6 days of MTS studies. It was demonstrated by Desmet et al. that light stimulation itself can promote cell proliferation.13 However, the cell proliferation on TCP under light stimulation after 6 days, was merely 5.4% (p ≤ 0.05) higher compared to cell proliferation on the non-stimulated TCP. On the other hand, the extent of cell proliferation was greatly improved by the combination of light stimulation with assistance of photosensitive P3HT-containing scaffolds. Using PCL/P3HT(10) as the substrate, we found higher proliferation (25.5%) of HDFs (p ≤ 0.05) under stimulated conditions compared to cell proliferation in non-stimulated (NS) conditions. The significant increase in cell proliferation on PCL/P3HT(10) scaffold was due to the photosensitive ability of PCL/P3HT(10) nanofibers under light stimulation. Meanwhile, the cell proliferation on stimulated PCL/P3HT(10) scaffolds was 16.1% higher compared to HDF proliferation on non-stimulated PCL. This result also supported the fact that the incorporation of optimized amount of P3HT in PCL/P3HT(10) favored cell proliferation through light stimulation. Furthermore, the proliferation of HDFs on PCL/P3HT(10) showed 12.8%, 11.9% and 11.6% (p ≤ 0.05) higher than the cell growth on PCL, PCL/P3HT(2) and PCL/P3HT(20) under light stimulated conditions, respectively (Fig. 6A). After 2, 4 and 6 days, the growth rates of cells on stimulated PCL/P3HT(2), PCL/P3HT(10), PCL/P3HT(20), PCL and TCP with respect to the non-stimulated scaffolds were also calculated (Fig. 6B). The proliferation of HDFs on day 2 and day 4 for light-stimulated PCL scaffolds was less compared to HDF proliferation on non-stimulated PCL. This could be because of the absence of P3HT (photosensitive material) within this scaffold. The inference was confirmed as we observed a similar effect on the scaffolds that contained minimum amounts of P3HT in our study, namely the PCL/P3HT(2) on day 2, which further increased its cell proliferation from day 4 onwards. By day 6, we observed that the growth of cells on all the stimulated scaffolds was higher compared to cells on corresponding non-stimulated scaffolds. Moreover, the cell proliferation on stimulated PCL/P3HT(10) scaffolds demonstrated both higher cell number and cell growth rate compared to the non-stimulated PCL. In conclusion, the amount of P3HT included in PCL/P3HT(10) is the optimized amount and is the most suitable scaffold for performing light-stimulated regenerative experiments and regenerative medicine.
 |
| Fig. 6 (A) HDF proliferation on electrospun PCL, PCL/P3HT(2), PCL/P3HT(10) and PCL/P3HT(20) nanofibrous scaffolds stimulated (S) compared to HDF proliferation on electrospun PCL/P3HT(2), PCL/P3HT(10), PCL/P3HT(20) and PCL nanofibrous scaffolds non-stimulated (NS) by MTS assay. * Significant against cell proliferation on TCP (NS) at p ≤ 0.05. ◆ Significant against cell proliferation on PCL/P3HT(2) (S) at p ≤ 0.05. # Significant against cell proliferation on PCL/P3HT(20) (S) at p ≤ 0.05. Significant against cell proliferation on PCL/P3HT(10) (NS) at p ≤ 0.05. ▼ Significant against cell proliferation on PCL/P3HT(10) and PCL (S) at p ≤ 0.05. ☆ Significant against cell proliferation on PCL/P3HT(10) and PCL (NS) at p ≤ 0.05. (B) Growth rate of cells on stimulated PCL/P3HT(2), PCL/P3HT(10), PCL/P3HT(20), PCL and TCP with respect to the non-stimulated scaffolds on day 2, day 4 and day 6. | |
The morphology of HDFs on electrospun PCL and PCL/P3HT(10) nanofibrous scaffolds was studied after 6 days light stimulation and the results are shown in Fig. 7. Fibroblasts attached on the scaffolds and stretched across the nanofibrous substrates upon proliferation. The number of cells that attached on PCL/P3HT(10) stimulated was higher than the number of cells attached on the same scaffold non-stimulated. Fibroblasts attached on PCL/P3HT(10) under light stimulation showed a characteristic spindle shape (Fig. 7A); however, fibroblasts attached on PCL and PCL/P3HT(10) nanofibrous scaffolds in non-stimulated conditions showed irregular morphology (Fig. 7D and 7E). From the SEM micrographs, we further established the non-toxicity of the PCL/P3HT(10) with successful adherence and proliferation of HDFs on PCL/P3HT(10) substrates under light stimulation, and confirmed their morphologies.
 |
| Fig. 7 SEM images of HDFs on electrospun (A) PCL/P3HT(10) (S), (B) PCL (S), (C) TCP (S), (D) PCL/P3HT(10) (NS), (E) PCL (NS) and (F) TCP (NS). | |
The mechanism of action could be such that the photosensitive polymer P3HT absorbs light (proved by UV-vis spectra), and photo-induced current was further generated in the P3HT-containing PCL fibers (shown as J–V curve), while the electromagnetic field was created. When the cells were stimulated by the electromagnetic field, Ca2+ ions translocated through the cell membrane voltage-gated calcium channels30 and this led to an increase in cytosolic Ca2+. The cytosolic Ca2+ would complex with the low molecular weight protein calmodulin, which then activated several key intracellular processes leading to cell division.31 Calmodulin was also thought to play a role in regulating the initiation of DNA synthesis. The increase in calmodulin-binding proteins in the nuclear fraction of cells stimulated cell proliferation.32 As a result, the increase in cytosolic Ca2+ led to an increase in cell proliferation (Fig. 8, green line). The effects of light stimulation (low power laser and light-emitting diode) on cell behavior were studied during the past few years and many mechanisms were proposed and one such mechanism was that the light irradiation (He–Ne laser, 632.8 nm) could induce the phosphorylation of tyrosine protein kinase receptor (TPKR) such as c-Met, the receptor of hepatocyte growth factor.33,34 After phosphorylation, TPKR will become an activated TPKR and it could promote the catalytic activity of phospholipase C (PLC)-gamma. The activated PLC c catalyzes the hydrolysis of some phospholipids which contain diacylglycerol (DAG) and inositol triphosphate (IP3). Further, the concentration of DAG and IP3 increase in the cytoplasm and the IP3 encourages the endoplasmic reticulum (ER) to release Ca2+, which works with DAG to activate protein kinase C (PKC).35,36 These are the routes through which PKCs are involved in improving the cell proliferation35,37 (Fig. 8). Minana et al. have previously shown that PKC inhibitors, namely H7 and calphostin C, prevent the proliferation of primary cell cultures.38 Similar results were also shown by Yoshimura et al. where they found that the proliferation of Schwann cells was significantly inhibited by staurosporine, a PKC inhibitor.39 Instead of inhibiting PKC, light stimulation activated PKC and improved the cell proliferation and this was also confirmed in our study, where we found higher HDF proliferation on PCL/P3HT(10) after light stimulation by MTS assay. It was proved that the biocompatibility of P3HT-containing PCL nanofibrous scaffolds were not as good as pure PCL nanofibrous scaffold. As a result, in was considered necessary to investigate in detail the amount of P3HT that might be optimum to be non-toxic to cells and support the proliferation of cells after light stimulation. Therefore, we followed a novel approach of incorporating various amounts of P3HT in PCL and studied the proliferation of fibroblasts cultured on P3HT-containing nanofibrous scaffolds. Our results demonstrated that the PCL/P3HT(10) favored cell proliferation after light stimulation and could be a suitable substrate for wound healing. Therefore, the cell–scaffold interaction studies by SEM, F-actin staining and collagen expression and such studies were not carried out on PCL/P3HT(2) and PCL/P3HT(20).
 |
| Fig. 8 Signal pathway involved in light stimulation induced cell proliferation (black line) and photocurrent induced cell proliferation (green dotted line). | |
3.5 F-actin staining
To understand the interaction of fibroblasts with PCL/P3HT(10) and PCL nanofibrous scaffolds under light stimulation, phalloidin staining was carried out. Phalloidin is a bicyclic peptide that belongs to a family of toxins isolated from the deadly Amanita phalloides “death cap” mushroom and is commonly used in imaging applications to selectively label filamentous actin in fixed cells.40 Phalloidin staining was carried out after 6 days of light stimulation and the F-actin stains showed a cytoplasmic filamentous distribution under LSCM. It was found that the cell density of proliferated HDF under light stimulation (Fig. 9A–C) was higher compared to HDFs proliferated under non-stimulated conditions (Fig. 9D–F). We observed that the cell density and morphology was better on PCL/P3HT(10) under light stimulation compared to other electrospun nanofibrous scaffolds (Fig. 9). The spindle-shaped morphology of the HDFs on PCL/P3HT(10) under light stimulation (Fig. 9A) was comparable to its phenotype on tissue culture plates (Fig. 9C). HDFs attached on PCL/P3HT(10) under light stimulation showed sufficient cell-to-cell communication. However, HDFs attached on PCL/P3HT(10) non-stimulated (Fig. 9D) showed limited cell–cell communication with limited distribution of cells. As a result, the cell density on PCL/P3HT(10) non-stimulated was lower than the cells proliferated on the same scaffold under light stimulation.
 |
| Fig. 9 Laser scanning confocal microscopic (LSCM) micrographs of HDFs grown on (A) PCL/P3HT(10) (S), (B) PCL (S), (C) TCP (S), (D) PCL/P3HT(10) (NS), (E) PCL (NS) and (F) TCP (NS), expressing F-actin. | |
3.6 Collagen expression
Collagen staining with picro-sirius red confirmed the secretion of ECM by the cells in culture. Fig. 10 shows the secretion of collagen on fibroblasts grown on PCL and PCL/P3HT(10) after 15 days of light stimulation. Results showed that the secretion of collagen on PCL/P3HT(10) under light stimulation was better than other scaffolds. HDFs proliferated on PCL/P3HT(10) under light stimulation showed the highest amount of collagen staining. Huang et al. evaluated the influence of LED photoirradiation (LED-PI) on fibroblast proliferation and collagen production in vitro and found that the collagen production increased significantly with LED-PI.41 It was also found that electrical stimulation (ES) promoted collagen synthesis. Morris et al. developed the Ahn/Mustoe lapine wound model for systematic investigation of the effects of ES on ischemic wound therapy. Both type I collagen (COL-I) and type V collagen (COL-V) showed significantly higher expressions between day 7 and day 14 over a longer duration of electrical pulse treatment.42 In our study we found that the higher cell proliferation on PCL/P3HT(10) under light stimulation contributed to increased collagen stains on these scaffolds. However, no obvious collagen stains were observed on TCP. The secretion of collagen on PCL/P3HT(10) proves the biocompatibility of PCL/P3HT(10) stimulated under light stimulation and its ability to induce cells for collagen expressions, thus potentially suitable as substrates for wound healing or skin tissue regeneration.
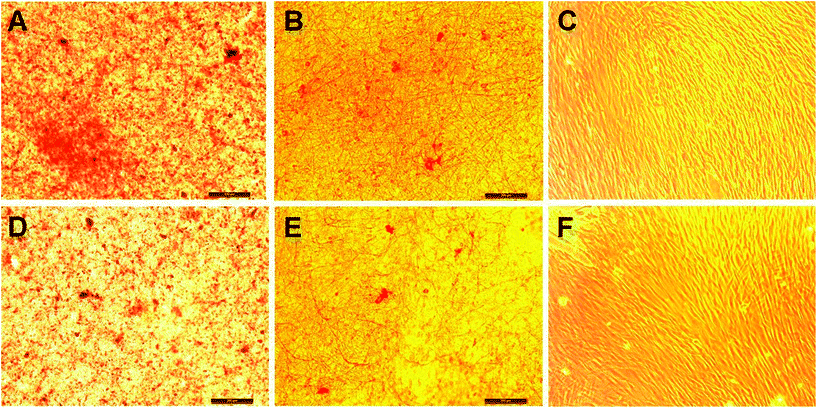 |
| Fig. 10 Collagen staining on fibroblasts on nanofibrous scaffolds: (A) PCL/P3HT(10) (S), (B) PCL (S), (C) TCP (S), (D) PCL/P3HT(10) (NS), (E) PCL (NS) and (F) TCP (NS). | |
Polymeric nanofibers are studied individually for skin TE and the photovoltaic property of solar cells has also been separately investigated by various researchers. However, to the best of our knowledge, there are no studies that focused on the application of nanofibrous scaffolds with photovoltaic ability for tissue regeneration. The photovoltaic ability of PCL/P3HT nanofiber scaffolds was demonstrated in this study by studying the current density versus voltage. The electrospun fiber can definitely absorb visible light and convert the optical energy to electrical energy causing the photocurrent effect. An optimized amount of P3HT was incorporated in PCL and our studies found higher HDF proliferation with collagen stains on PCL/P3HT(10) scaffolds after light stimulation. All these results proved that PCL/P3HT(10) could be a promising substrate for photocurrent therapy in skin TE.
4. Conclusions
Functional photosensitive P3HT-containing PCL nanofibrous scaffolds were fabricated by electrospinning. Fibroblast proliferation on PCL, PCL/P3HT(2), PCL/P3HT(10) and PCL/P3HT(20) nanofibrous scaffolds under light stimulation was studied and the results of our study suggest that fibroblasts cultured on PCL/P3HT(10) after light stimulation had better proliferation and morphology than fibroblasts cultured on electrospun PCL, PCL/P3HT(2) and PCL/P3HT(20) nanofibers. Electrospun PCL/P3HT(10) nanofibrous scaffold was capable of supporting cell proliferation and favoring cell ECM secretion. We concluded that the electrospun PCL/P3HT(10) nanofibers are suitable substrates for wound healing applications.
References
- K. C. Madison, Barrier function of the skin: “La Raison d’Être” of the epidermis, J. Invest. Dermatol., 2003, 121, 231–241 CrossRef CAS.
- E. Proksch, J. M. Brandner and J. M. Jensen, The skin: an indispensable barrier, Exp. Dermatol., 2008, 17, 1063–1072 CrossRef.
- S. Hettiaratchy and P. Dziewulski, ABC of burns. Introduction, Br. Med. J., 2004, 328, 1366–1368 CrossRef.
- J. M. Torpy, C. Lynm and R. M. Glass, Burn injuries, JAMA, J. Am. Med. Assoc., 2009, 302, 1828 CrossRef CAS.
- R. Guo, S. Xu, L. Ma, A. Huang and C. Gao, The healing of full-thickness burns treated by using plasmid DNA encoding VEGF-165 activated collagen-chitosan dermal equivalents, Biomaterials, 2011, 32, 1019–1031 CrossRef CAS.
- V. Beachley and X. Wen, Fabrication of nanofiber reinforced protein structures for tissue engineering, Mater. Sci. Eng., C, 2009, 29, 2448–2453 CrossRef CAS.
- H. Friedmann, R. Lubart, I. Laulicht and S. Rochkind, A possible explanation of laser-induced stimulation and damage of cell cultures, J. Photochem. Photobiol., B, 1991, 11, 87–91 CrossRef CAS.
- H. Bai, C. D. McCaig, J. V. Forrester and M. Zhao, DC electric fields induce distinct preangiogenic responses in microvascular and macrovascular cells, Arterioscler., Thromb., Vasc. Biol., 2004, 24, 1234–1239 CrossRef CAS.
- J. A. Feedar, L. C. Kloth and G. D. Gentzkow, Chronic dermal ulcer healing enhanced with monophasic pulsed electrical stimulation, Phys. Ther., 1991, 71, 639–649 Search PubMed.
- C. T. Brighton, W. Wang, R. Seldes, G. H. Zhang and S. R. Pollack, Signal transduction in electrically stimulated bone cells, J. Bone Joint Surg.-Am. Vol., 2001, 83A, 1514–1523 Search PubMed.
- H. P. Wiesmann, M. Hartig, U. Stratmann, U. Meyer and U. Joos, Electrical stimulation influences mineral formation of osteoblast-like cells in vitro, BBA-Mol. Cell. Res., 2001, 1538, 28–37 Search PubMed.
- M. J. Conlan, J. W. Rapley and C. M. Cobb, Biostimulation of wound healing by low-energy irradiation. A review, J. Clin. Periodontol., 1996, 23, 492–496 CrossRef CAS.
- K. D. Desmet, D. A. Paz, J. J. Corry, J. T. Eells, M. T. T. Wong-Riley, M. M. Henry, E. V. Buchmann, M. P. Connelly, J. V. Dovi, H. L. Liang, D. S. Henshel, R. L. Yeager, D. S. Millsap, J. Lim, L. J. Gould, R. Das, M. Jett, B. D. Hodgson, D. Margolis and H. T. Whelan, Clinical and experimental applications of NIR-LED photobiomodulation, Photomed. Laser Surg., 2006, 24, 121–128 CrossRef CAS.
- A. Greiner and J. H. Wendorff, Electrospinning: a fascinating method for the preparation of ultrathin fibers, Angew. Chem., Int. Ed., 2007, 46, 5670–5703 CrossRef CAS.
- J. P. Cannon, S. D. Bearden and S. A. Gold, Effect of wetting solvent on poly(3-hexylthiophene) (P3HT) nanotubles fabricated via template wetting, Synth. Met., 2010, 160, 2623–2627 CrossRef CAS.
- A. Santos, P. Formentn, J. Pallares, J. Fere-Borrull and L. F. Marsal, Fabrication and characterization of high-density arrays of P3HT nanopillars on ITO/glass substrates, Sol. Energy. Mater. Sol. Cells., 94, 1247–1253 Search PubMed.
- S. Sundarrajan, R. Murugan, A. S. Nair and S. Ramakrishna, Fabrication of P3HT/PCBM solar cloth by electrospinning technique, Mater. Lett., 2010, 64, 2369–2372 CrossRef CAS.
- E. J. Chong, T. T. Phan, I. J. Lim, Y. Z. Zhang, B. H. Bay, S. Ramakrishna and C. T. Lim, Evaluation of electrospun PCL/gelatin nanofibrous scaffold for wound healing and layered dermal reconstitution, Acta Biomater., 2007, 3, 321–330 CrossRef CAS.
- M. Cheng, J. Deng, F. Yang, Y. Gong, N. Zhao and X. Zhang, Study on physical properties and nerve cell affinity of composite films from chitosan and gelatin solutions, Biomaterials, 2003, 24, 2871–2880 CrossRef CAS.
-
S. Ramakrishna, K. Fujihara, W. E. Teo, T. C. Lin and Z. Ma, in Electrospinning Process. Electrospinning and Nanofibres, World Scientific Publishing, Singapore, 2005, pp. 98–99 Search PubMed.
- G. Jin, M. P. Prabhakaran, B. P. Nadappuram, G. Singh, D. Kai and S. Ramakrishna, Electrospun poly(l-Lactic acid)-co-poly(ε-caprolactone) nanofibres containing silver nanoparticles for skin tissue engineering, J. Biomater. Sci. Polym. Ed., 2012 DOI:10.1163/156856211X617399.
- A. Jena and K. Gupta, Characterization of pore structure of filtration media, Fluid/Part. Sep. J., 2002, 14, 227–241 Search PubMed.
- A. Jena and K. Gupta, Pore volume of nanofiber nonwovens, Int. Nonwovens J., 2005, 14, 25–30 Search PubMed.
- D. Li, M. W. Frey and Y. L. Joo, Characterization of nanofibrous membranes with capillary flow porometry, J. Membr. Sci., 2006, 286, 104–114 CrossRef.
- S. J. Eichhorn and W. W. Sampson, Statistical geometry of pores and statistics of porous nanofibrous assemblies, J. R. Soc. Interface, 2005, 2, 309–318 CrossRef.
- K. H. K. Chan, T. Yamao, M. Kotaki and S. Hotta, Unique structural features and electrical properties of electrospun conjugated polymer poly(3-hexylthiophene) (P3HT) fibers, Synth. Met., 2010, 160, 2587–2595 CrossRef CAS.
-
R. D. McCullough and P. C. Ewbank, in Handbook of Conducting Polymers, ed. T. A. Skotheim, Marcel Dekker, New York, 1998, pp. 225–258 Search PubMed.
- J. L. Bredas, Relationship between band gap and bond length alternation in organic conjugated polymers, J. Chem. Phys., 1985, 82, 3808–3811 CrossRef CAS.
- V. Shrotriya, J. Ouyang, R. J. Tseng, G. Li and Y. Yang, Absorption spectra modification in poly(3-Hexylthiophene):methanofullerene blend thin films, Chem. Phys. Lett., 2005, 411, 138–143 CrossRef CAS.
- C. Brighton, W. Wang, R. Srldes, G. Zhang and S. Pollack, Single transduction in electrically stimulated bone cells, J. Bone. Joint. Surg. Am., 2001, 83-A, 1514–1523.
- S. Tomlinson, S. MacNeil, S. Walker, C. Ollis, J. Merrit and B. Brown, Calmodulin and cell function, Clin. Sci., 1984, 66, 497–507 Search PubMed.
- O. Bachs, L. Lanini, J. Serratosa, M. J. Coll, R. Bastos, R. Aligue, E. Rius and E. Carafoli, Calmodulin-binding proteins in the nuclei of quiescent and proliferatively activated rat liver cells, J. Biol. Chem., 1990, 265, 18595–18600 CAS.
- G. Shefer, U. Oron, A. Irintchev, A. Wernig and O. Halevy, Skeletal muscle cell activation by low-energy laser irradiation: A role for the MAPK/ERK pathway, J. Cell. Physiol., 2001, 187, 73–80 CrossRef CAS.
- X. Gao and D. Xing, Molecular mechanisms of cell proliferation induced by low power laser irradiation, J. Biomed. Sci., 2009, 16, 4 CrossRef.
- D. C. Braun, S. H. Garfield and P. M. Blumberg, Analysis by fluorescence resonance energy transfer of the interaction between ligands and protein kinase Cδ; in the intact cell, J. Biol. Chem., 2004, 280, 8164–8171 CrossRef.
- S. G. Rhee and Y. S. Bae, Regulation of phosphoinositide-specific phospholipase C isozymes, J. Biol. Chem., 1997, 272, 15045–15048 CrossRef CAS.
- M. Musashi, S. Ota and N. Shiroshita, The role of protein kinase C isoforms in cell proliferation and apoptosis, Int. J. Hematol., 2000, 72, 12 Search PubMed.
- M. Minana, H. Cabedo, V. Felipo and S. Grisolia, Inhibition of the proliferation of primary cell cultures and of L-132 cells by protein kinase C inhibitors, Cancer. J., 1993, 6, 136–141 Search PubMed.
- T. Yoshimura, S. Goda, T. Kobayashi and I. Goto, Involvement of protein kinase C in the proliferation of cultured Schwann cells, Brain Res., 1993, 617, 55–60 CrossRef CAS.
- J. Cooper, Effects of cytochalasin and phalloidin on actin, J. Cell Biol., 1987, 105, 1473–1478 CrossRef CAS.
- P. J. Huang, Y. C. Huang, M. F. Su, T. Y. Yang, J. R. Huang and C. P. Jiang,
In vitro bservations on the influence of copper peptide aids for the LED photoirradiation of fibroblast collagen synthesis, Photomed. Laser Surg., 2007, 25, 183–190 CrossRef CAS.
- K. Morris, M. McGee, J. Jasper and K. Bogie, Evaluation of electrical stimulation for ischemic wound therapy: a feasibility study using the lapine wound model, Arch. Dermatol. Res., 2008, 301, 323–327.
Footnote |
† This article is published as part of a themed issue on current topics in photodermatology. |
|
This journal is © The Royal Society of Chemistry and Owner Societies 2013 |
Click here to see how this site uses Cookies. View our privacy policy here.