DOI:
10.1039/C2NR32835F
(Paper)
Nanoscale, 2013,
5, 231-238
NIR light controlled photorelease of siRNA and its targeted intracellular delivery based on upconversion nanoparticles†
Received
20th September 2012
, Accepted 23rd October 2012
First published on 30th October 2012
Abstract
The most notable role of small interfering RNA (siRNA) is in RNA interference (RNAi) and post-transcriptional gene silencing, which leads to a surge of interest in RNAi for both biomedical research and therapeutic applications. However, “naked” siRNA cannot cross cellular membranes freely because of highly negative charges which limits its utility for gene therapy. In this work, a system of near-infrared (NIR) light-induced siRNA release from silica coated upconversion nanoparticles (Si-UCNPs) is presented. These Si-UCNPs were functionalized with cationic photocaged linkers through covalent bonding, which could effectively adsorb anionic siRNA through electrostatic attractions and were easily internalized by living cells. Upon NIR light irradiation, the photocaged linker on the Si-UCNPs surface could be cleaved by the upconverted UV light and thus initiated the intracellular release of the siRNA. The in vitro agarose gel electrophoresis and intracellular imaging results indicated that the Si-UCNPs-based gene carrier system allowed effective siRNA delivery and the applications of NIR light instead of direct high energy UV irradiation may greatly guarantee less cell damage.
1. Introduction
Small interfering RNA (siRNA) has emerged as a gene-based therapy due to their highly desirable roles in RNA interference and gene silencing effects in biomedical research. One of the key requirements for an effective siRNA therapy is that sufficient siRNA need to be introduced into cells or organs.1–4 However, because of the highly negatively charged nature of “naked” siRNA, it cannot spontaneously diffuse across cellular membranes, which becomes the most restrictive factor in siRNA-based therapy.5,6 Currently, many types of siRNA-carrier complexes have been developed for the purpose of effective siRNA delivery and targeted gene knockdown.7–10 Moreover, a number of nanomaterial conjugates such as gold nanoparticles, mesoporous silica nanoparticles and polymeric nanoparticles with positively charged functional groups to allow surface modification, have also been explored as delivery vehicles for transporting siRNA into living cells.11–17 Despite the promising progress with respect to their efficient siRNA delivery and specific gene silencing in living cells, remote control the release of siRNA or unpacking siRNA inside target cells in a highly spatial and temporal precision remains a critical challenge and systematic studies still need to be fully exploited.
So far, several intracellular controlled release strategies on the basis of light irradiation, low pH, enzymatic hydrolysis, redox reaction, etc., have been extensively established to direct the targeted delivery of bioactive moieties including therapeutic oligonucleotides into the living cells.18–24 Among various strategies, the photolysis or the use of a beam of light represents a unique approach to achieve time- and site-specific control of effective release of payload molecules from the carrier systems, which have been currently recognized as new directions for their promising biomedical practices in gene, drug or other related biomolecules delivery in vitro and in vivo.25–28 However, one potential problem which exists in most current photolysis delivery systems is that the activation of bioactive molecules must rely on the short wavelength UV irradiation. The unavoidable cellular damage and reduced tissue penetration would largely impede their further applications in vivo. Therefore, the search for appealing alternatives toward effective photorelease while maintaining minimum side effects in living cells is highly required. Recently, core–shell lanthanide-doped upconversion nanoparticles (UCNPs) have attracted much attention in the fields of biological imaging and targeted delivery mostly attributed to their novel optical properties.29–38 In general, UCNPs can absorb long wavelength NIR light and give out narrow and sharp emissions ranging from UV to visible or even NIR region. Such unique upconversion observed in UCNPs provides major advantages to suppress autofluorescence and to minimize photo damage to living cells, which enabled UCNPs to work as promising NIR-responsive delivery cargos for biological studies. Indeed, there are a few reports exploiting the converted UV or visible light from UCNPs to activate photocaged therapeutic agents or imaging probes in vitro and in vivo.39–42 Although, very recently, NIR-induced UCNPs delivery system has also been reported to successfully photorelease caged nucleic acids in cells and living animals.43 The chemical modification of target nucleic acids with photocaged groups has to be involved in order to functionalize the delivered nucleic acids and enhance their physical adsorption onto nanoparticles surface. Extensive studies to develop simple and reliable NIR photoactivatable nanoparticle platforms with higher payload of more diverse and intact DNA/siRNA moieties remain highly desirable in the field of gene therapy and have been minimally investigated so far.
Herein, we present a simple and effective NIR light reactive nanoparticle carrier system to exert target siRNA delivery in host living cells on the basis of silica coated UCNPs. In our system, lanthanide ytterbium (Yb3+) and thulium (Tm3+) co-doped sodium yttrium fluoride (NaYF4) nanocrystals were chosen as templates, which were coated with silica layer for the purpose of increasing biocompatibility and facilitating the subsequent chemical modification.44–46 The as-synthesized Si-UCNPs were then functionalized by cationic photocaged linkers through covalent bonding, which would greatly promote the physical adsorption of target DNA or siRNA on the particles surface and thus facilitated the cell membrane penetration for effective delivery in living cells. Scheme 1A illustrates the experimental design of the silica coated UCNPs carrier for the target siRNA delivery by using photoactive o-nitrobenzyl ester functionalized thiol-linker with a positively charged alkyl amine at the end of the structure. The constructed Si–UCNPs conjugate will associate with siRNA through electrostatic interactions between the phosphate ions in siRNA and the cationic ammonium on the surface of Si-UCNPs. Upon 980 nm laser irradiation, the upconverted UV light emitted from UCNPs will cleave the photosensitive o-nitrobenzyl linker and split the positively charged amino group from nanoparticles surface, and will therefore result in the efficient release of siRNA to silence target gene expression in living cells (Scheme 1B). The in vitro agarose gel electrophoresis and intracellular imaging experiments indicated that the constructed NIR light-induced siRNA carrier system could efficiently eliminate the membrane barrier and provided a simpler and more effective approach for targeted gene delivery with less photo damage in living cells.
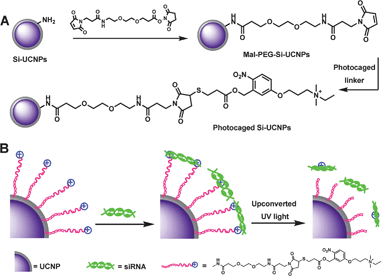 |
| Scheme 1 Schematic illustration of (A) the synthesis processes of cationic photocaged Si-UCNPs; (B) siRNA adsorption on the particles surface and then photo-release by upconverted UV light from UCNPs. | |
2. Experimental
2.1 Preparation of Si-UCNPs and NIR-responsive photoactived Si-UCNPs
Preparation of Si-UCNPs.
The monodispersed core–shell UCNPs composed of NaYF4 nanocrystals doped with Yb3+ and Tm3+ were synthesized according to the reported protocols.41,42,47 Then the silica coated UCNPs were prepared by following the literature procedure.48,49 Briefly, UCNPs (5 mg) were firstly dissolved in cyclohexane (20 mL). Triton X-100 (5 mL), 1-hexanol (5 mL) and Milli-Q water (850 μL) were added to the solution subsequently. Then TEOS (tetraethyl orthosilicate, 8 μL) and (3-aminopropyl)triethoxysilane (APTES, 2 μL) were added into the mixture for 6 hours and followed by further addition of NH4OH (200 μL). After stirring for 12 hours, the final product was collected by centrifugation and washed thoroughly with ethanol. Both of the prepared UCNPs and silica coated UCNPs were characterized by dynamic light scattering (DLS) and transmission electron microscopy (TEM), respectively. The luminescence emission spectrum (λex = 980 nm) of the UCNPs (0.05 mg mL−1, assuming the yield of the coating step was 100%) were recorded on a DM150i monochromator equipped with a R928 photon counting photomultiplier tube (PMT), in conjunction with a 980 nm diode laser.
Preparation of NIR-responsive photoactived Si-UCNPs.
The as-prepared Si-UCNPs (5 mg) were dissolved in dimethyl formamide (DMF, 100 μL) and then the solution of 100 μL of Mal-dPEG™2-NHS ester in DMF (20 mM, from Quanta Biodesign) and triethylamine (TEA, 2 μL) were added. The reaction mixture was stirred under dark conditions for 20 hours. Finally, the Mal-PEG functionalized nanoparticles were collected and washed by DMF three times.
To the suspension of Mal-PEG-modified nanoparticles (5 mg) in DMF (100 μL), o-nitrobenzyl photocaged linker (5 mM, 300 μL in DMF) and TEA (2 μL) were added. The reaction continued for 24 hours under dark conditions at room temperature. After collecting the photoactived Si-UCNPs by centrifugation, the product was washed by DMF/EtOH (v
:
v = 1
:
5) three times, followed by washing with the phosphate buffered saline (PBS, 0.1 M, pH = 7.2) three times to remove the organic solvent. The obtained product was resuspended in PBS and stored at −20 °C for further use. The loading efficiency for the photoactive linker was estimated by measuring the absorbance of photocaged group at 321 nm in DMSO. A calibration curve determined with different concentrations of photocaged linker (in DMSO) was used to calculate the value.
2.2 Loading of siRNA onto the surface of silica coated UCNPs
The loading of siRNA to the Si-UCNPs was conducted according to the method reported previously.11,21 The Alexa-siRNA was used to mix with cationic photocaged Si-UCNPs in PBS solution with different ratios (siRNA
:
photocaged linker = 1
:
5, 1
:
10 and 1
:
15). The final concentrations of siRNA were maintained at 1 μM. After 20 minutes incubation, the mixture was centrifuged and the pellet was gently washed with PBS two times to remove free Alexa-siRNA. The Alexa-siRNA adsorbed Si-UCNPs complex was resuspended in PBS and the uploading of siRNA onto nanoparticles surface was evaluated by agarose gel electrophoresis experiment (ESI†).
2.3
In vitro photoactivation of Si-UCNPs–Alexa-siRNA by 980 nm light irradiation
A solution (10 μL, 10 μM of siRNA in PBS) of Si-UCNPs–Alexa-siRNA (15
:
1) sample was transferred to a 1.5 mL Eppendorf tube for 980 nm light exposure. After 2 hours irradiation, the sample was diluted to 1 μM for UV-vis absorption measurement. The solution of Si-UCNPs–Alexa-siRNA (15
:
1) (100 μL, 1 μM of siRNA) was irradiated by UV light for 5 minutes which was used as control experiment.
2.4 Cell imaging and gene silencing experiments
Cell imaging for the photolysis of Si-UCNPs–Alexa-siRNA.
HeLa cells were purchased from ATCC (ATCC no.: CCL-2) and maintained in Dulbecco's Modified Eagle Medium (DMEM) containing 10% FBS. All cultures were incubated at 37 °C in atmosphere environment with 5% CO2.
HeLa cells were seeded in a 35 mm diameter μ-dish plastic-bottom (ibidi GmbH, Germany) and cultured for 24 hours in DMEM medium without FBS. After washing with DMEM medium three times, 0.1 μM of freshly prepared Si-UCNPs–Alexa-siRNA complex in 1 mL of DMEM medium was added to the cells and incubated at 37 °C. After 1 hour incubation, cells were washed twice with DMEM medium and illuminated with a 980 nm laser (with a 5 min break after each 30 min exposure, 2 hours irradiation). After removal of culture medium and followed by washing with Hank's Buffered Salt Solution (HBSS buffer) two times, the cellular imaging measurements were conducted by using fluorescence microscopy (Nikon, Eclipse TE2000-E). In the process of photo-controlled siRNA delivery, the standard Alexa-siRNA releasing experiment transferred by lipofectamine 2000 (Lipo2k, from Invitrogen) was used as a positive control. Similarly, HeLa cells incubated with Si-UCNPs–Alexa-siRNA complex but without light irradiation were also conducted, which was applied as a negative control.
Moreover, cellular imaging experiments with prolonged incubation times were also carried out for further study of the efficiency of NIR light-induced siRNA delivery. Basically, HeLa cells were incubated with Si-UCNPs–Alexa-siRNA complex for 1 hour and followed by 2 hours 980 nm light irradiation. After washing with HBSS buffer two times, the cell samples were used for fluorescence microscopy imaging studies. As a control, imaging measurements were also performed by using HeLa cells incubated with Si-UCNPs–Alexa-siRNA complex for 3 hours but without any light irradiation.
Gene expression and silencing experiments.
In order to evaluate if both gene expression and gene silencing would be effectively regulated by the developed NIR-responsive UCNPs platform, the photo-controlled siRNA release in the target cell lines with standard gene transfection was performed. In this typical experiment, plasmid encoding with EGFP gene was chosen for the imaging study. The transfection process was carried out according to the standard protocol provided from Invitrogen. Basically, HeLa cells were seeded in 35 mm diameter μ-dish plastic bottom for 20 hours to give about 70–80% cell confluency, culture medium was replaced with Opti-MEM medium (1 mL). 0.6 ng EGFP DNA plasmid was gently mixed with 100 L Opti-MEM for 5 minutes. Meanwhile, 4 μL Lipo2k was gently diluted in 100 μL Opti-MEM medium for another 5 minutes. The two solutions were mixed together for 20 minutes at room temperature, then the mixed solution was added into cells and followed by incubation for 5 hours. The medium was changed to DMEM with 10% FBS. Cell images were taken after 24 hours incubation.
After transferring EGFP plasmid into HeLa cells, Si-UCNPs–EGFP siRNA (Si-UCNPs–siRNA) complex (15
:
1, concentration of EGFP siRNA 40 pmol) in Opti-MEM was added subsequently. Then NIR irradiation was taken for 2 hours to release the siRNA. After replacing the medium with DMEM containing 10% FBS and followed by incubation for 24 hours, fluorescent imaging experiments were performed to evaluate the process of gene silencing.
As a contrast, EGFP siRNA transferred by Lipo2k was used as a positive control. Briefly, 40 pmol siRNA and 2 μL Lipo2k were diluted in 100 μL Opti-MEM respectively. After 5 minutes incubation, the two Opti-MEM solutions were mixed for another 20 minutes. Finally, the mixed Opti-MEM solutions were added into the EGFP plasmid transferred cell dish. Medium was changed after 5 hours incubation and cells were incubated for another 24 hours for further imaging measurements.
3. Results and discussion
3.1 Preparation of Si-UCNPs and photoactived Si-UCNP
In a typical experiment, the Yb3+/Tm3+ co-doped UCNPs were prepared by according to the protocol from the literature.41,42,47 The obtained nanocrystals were suspended in cyclohexane and further encapsulated in a silica shell with amine groups on the surface by using a water-in-oil microemulsion method.42,48,49 Both of the UCNPs and Si-UCNPs were characterized by TEM and DLS. As shown in Fig. 1A–D, the average size of UCNPs and Si-UCNPs were approximately about 65 nm and 74 nm respectively, which are suitable for effective cellular uptake.50,51 The silica layer coating is for the purpose of increasing the biocompatibility of UCNPs. Moreover, this silica shell can also facilitate easy surface functionalization for further biomedical studies. In contrast, the luminescence spectra shown in Fig. 1E and F indicated that all the post-modifications of the UCNPs would not affect their optical properties. In other words, the modified UCNPs exhibited the same optical properties as the “naked” UCNPs, including UV, visible and NIR light emission.
In order to achieve high thiol-photolinker loading efficiency, the Si-UCNPs were firstly modified with maleimide-terminated oligo(ethylene glycol) linker. It is well-known that addition of PEG linker will improve the dispersion stability of nanoparticles in aqueous solutions. The simple and specific thiol–maleimide conjugation between the cationic photocaged linker and PEG modified Si-UCNPs led to the formation of NIR-responsive nanoparticle delivery cargos. The loading efficiency of the photocaged linker on the surface of silica-UCNPs was determined by the UV-vis absorbance spectrum (Fig. 2) and the loading amount was estimated to be about 8000 molecules per nanoparticle.38 These photoactive linker functionalized Si-UCNPs were suitable for loading of siRNA because of the positive charge of the cationic linker.
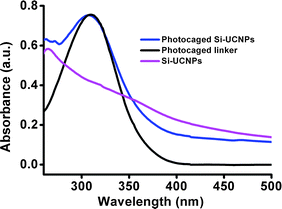 |
| Fig. 2 UV-vis spectra of Si-UCNPs, photocaged linker and photocaged linker conjugated Si-UCNPs. | |
3.2 Loading and unloading of siRNA triggered by NIR light in vitro
We next studied the loading and light-induced release of siRNA from the photocaged UCNPs platform. As a proof-of-concept, the Alexa Fluor@546 labeled siRNA (Alexa-siRNA) was loaded on the nanoparticles by mixing the particles with the siRNA (ratio
:
cationic linker/siRNA = 5
:
1, 10
:
1, 15
:
1) in PBS for 20 minutes at room temperature. As shown in Fig. 3A, the fluorescence intensity in gel electrophoresis indicated that about 60% siRNA was adsorbed on the particles surface in the case of 5
:
1 mixing ratio. When the ratio increased to 15
:
1, nearly all of the siRNA was found to be adsorbed onto the cationic photocaged UCNPs. Whereas the particles without cationic photocaged linker did not adsorb siRNA so tightly which could easily move in the gel running process (data not shown). Moreover, we have also monitored the loading procedure by measuring the fluorescence spectrum. The fluorescence intensity was decreased during the loading process (Fig. 3B), most likely due to the intermolecular interactions between dye molecules when they were densely packed on the nanoparticles surface.52,53 The loading capacity of Alexa-siRNA was characterized by measuring UV-vis absorption spectrum (Fig. S1†) and running agarose gel electrophoresis (Fig. 3A). The results showed that about 0.7 wt% Alexa-siRNA in total was adhered on the surface of the platform.
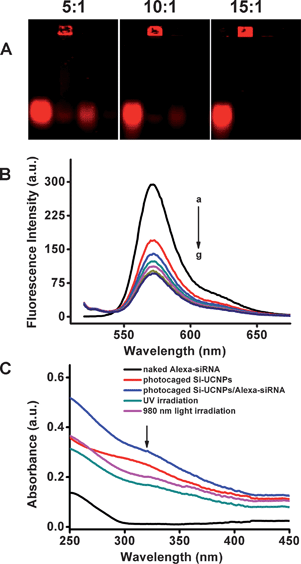 |
| Fig. 3
In vitro characterization of Si-UCNPs–Alexa-siRNA complex. (A) Agarose gel electrophoresis analysis of Alexa-siRNA (1 μM) loading with cationic Si-UCNPs with different ratios: 1 : 5, 1 : 10 and 1 : 15 (siRNA: photocaged linker). For each gel image, from left to right, lane 1: Alexa-siRNA solution; lane 2: Si-UCNPs–Alexa-siRNA complex; lane 3: supernatant after removal of the complex; lane 4: washing solution of the complex. (B) Time course of fluorescence spectra of Alexa-siRNA loading: (a–g: 0, 1 min, 2 min, 5 min, 10 min, 15 min, 20 min). (C) Absorbance spectra of Alexa-siRNA (black), photocaged Si-UCNPs (red), Alexa-siRNA loaded on photocaged Si-UCNPs (blue), the Si-UCNPs–siRNA complex irradiated with UV (dark cyan) 5 minutes and the Si-UCNPs–Alexa-siRNA complex irradiated with NIR light (magenta) 2 hours. | |
After the adsorption of the siRNA on the particle surface, NIR light-induced siRNA release from photocaged UCNPs was investigated. The solution of photoactived Si-UCNPs–Alexa-siRNA complex was exposed to light and the photo-cleaved process was monitored by UV-vis spectroscopy. As a proof-of-concept, UV light was firstly used to confirm the uncaging properties of the developed system. As shown in Fig. 3C, upon irradiation with UV light for 5 min, the peak (dark cyan line) of photocaged functional groups at 321 nm was significantly decreased, indicating the effective uncaging of photocaged linker caused by UV irradiation which subsequently resulted in the release of siRNA. With this information in hand, the Si-UCNPs–Alexa-siRNA complex was exposed with NIR light. Under this light irradiation, the cationic part of photocaged linker was cleaved and a similar phenomenon was observed (magenta line), in which the absorption at 321 nm also dropped (Fig. 3C). The decreased absorption at 321 nm indicated that the breakage of the ester bond and the positively charged alkyl amine part was released from the platform. Due to the change of charged properties on the surface of nanoparticles, the siRNA can easily escape from nanoparticles. These results demonstrated the fact that the photoactived Si-UCNPs–Alexa-siRNA complex can be uncaged and the adsorbed siRNA can be easily released from the platform by NIR light irradiation.
3.3 Light-induced siRNA release in living cells
Encouraged by the promising results of effective siRNA release with NIR light irradiation in vitro, we further examined the potential transfection of Si-UCNPs–Alexa-siRNA complex in living cells. HeLa cell lines were utilized for a living cell experimental study. In this study, the Si-UCNPs–Alexa-siRNA conjugate (siRNA concentration, 25 nM) was incubated with cells for 1 hour followed by another 2 hours of NIR light irradiation. As we mentioned above, the fluorescence intensity was quenched by about 75% when Alexa-siRNA was loaded on the particles surface. After incubation with cells, weak red fluorescence signals were detected from the cells, which reflected the fact that the complex was efficiently uptaken by living cells. As shown in Fig. S2C,† the red fluorescence intensity significantly increased after NIR light irradiation. Due to the converted UV light emitted from UCNPs after 980 nm laser exposure, the o-nitrobenzyl groups were cleaved which induced the Alexa-siRNA release from the nanoparticles platform, whereas the cells treated with the Lipo2k/siRNA only showed very weak fluorescence signals under the same concentration of Alexa-siRNA, which further confirmed the effective siRNA delivery from the setup system (Fig. S2E†).
In order to further study the efficiency of siRNA delivery from photocaged UCNPs through NIR light irradiation, another two comparative cell experiments were also conducted accordingly. Typically, in the first experiment, the cells were incubated with silica-UCNPs–Alexa-siRNA complex for 3 hours but no light illumination, while the sample in the second experiment was incubated for 1 hour followed by 2 hours NIR light irradiation. The fluorescence microscopy images of two samples were shown in Fig. 4A and B, from which we can see that both of the cell samples showed red fluorescence signals. However, the cells which have been irradiated with 980 nm light exhibited much stronger fluorescence intensity than the one without light exposure (Fig. 4C).54,55 These images also further confirmed the efficiency of NIR light induced siRNA delivery in living cells.
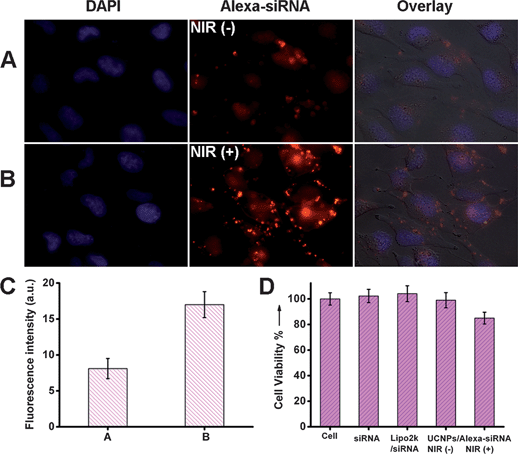 |
| Fig. 4 (A) Cell images of HeLa incubated with Si-UCNPs–Alexa-siRNA complex for 3 hours, and stained by DAPI. (B) Cell images of HeLa incubated with Si-UCNPs–Alexa-siRNA complex for 1 hour followed by 2 hours NIR light irradiation, and stained by DAPI. The concentration of Alexa-siRNA was 25 nM. (C) Analysis of fluorescence colocalization of Alexa-siRNA fluorescence in (A) and (B) with Image J software.54,55 (D) Cell viability of Si-UCNPs–Alexa-siRNA without or with NIR light irradiation. As controls, cells without any treatment, cells incubated with siRNA and cells incubated with Lipo2k/siRNA were also performed. | |
For further evaluation of the potential toxicity from NIR light irradiation, the cytotoxicity experiments were performed by standard MTT assay.22,56 Meanwhile, the Lipo2k/siRNA was also chosen as control and viability percentage of the cells without any treatment was set as 100%. From Fig. 4D, we can see that the UCNPs–Alexa-siRNA complex without NIR light irradiation showed no significant cytotoxicity when incubated with HeLa cells. Although the potential long term toxicity of UCNPs could not be excluded completely,57 the general cellular incubation results indicated that the developed siRNA delivery platform will not significantly affect the living cells viability and could be used for further biological studies safely. Similarly, the UCNPs–Alexa-siRNA complex also showed very weak cell cytotoxicity upon NIR light irradiation, which can be attributed to the light-induced thermal effect. However, this NIR light generated cytotoxicity was much less weak than that caused by UV light exposure (Fig. S3†).
3.4 Gene silencing test
The cellular imaging experiments have shown that siRNA could be successfully delivered into cells by the photocaged Si-UCNPs nanocarriers. When evaluating the effectiveness of the gene delivery pipeline, one of the most important concerns is if the delivered siRNA retains sufficient activity. The most notable role of siRNA is in their interference with the RNA pathway, where it interferes with the expression of some specific genes. To this purpose, another experiment has been conducted for the gene silencing measurements. In this typical experiment, plasmid encoding with enhanced green fluorescence protein (EGFP) gene was chosen for the imaging study. The Si-UCNPs–EGFP siRNA (Si-UCNPs–siRNA) complex was prepared by using the same method which was mentioned previously in section 2.4. Four parallel groups of HeLa cells samples were chosen to be transferred with the Lipo2k/EGFP plasmids in order to express the GFP. In parallel to this GFP expression, the second group sample was also treated with Lipo2k/siRNA as a control by using the standard gene silencing method (protocol supplied by Invitrogen, Fig. 5C and D). While the 3rd and 4th group of cell samples were treated with Si-UCNPs–siRNA complex, but only the last cells samples (4th group) were further exposed with NIR light after 2 hours incubation (Fig. 5G). The gene knockdown was checked after 24 hours incubation by taking the confocal microscopy images. As expected, the strong green fluorescence observed in Fig. 5A and no fluorescence signal observed in Fig. 5C clearly indicated that GFP could be effectively expressed by using standard cargo on the basis of Lipo2k, and meanwhile, siRNA transferred by Lipo2k could also significantly knockdown EGFP gene expression. With this information, then we discussed the phenomenon indicated in the rest of fluorescence images. As shown in Fig. 5E, very strong green fluorescence intensity was detected from cells which has been treated with Si-UCNPs–siRNA but there was no 980 nm laser irradiation. However, the cells treated with Si-UCNPs–siRNA and followed by 2 hours NIR light irradiation displayed very weak fluorescence signals (Fig. 5G). These results suggested that photocaged Si-UCNPs could effectively deliver siRNA into target cells, and NIR laser illumination could cleave photocaged functional groups and greatly activate the release of siRNA for the subsequent gene silencing in host cells. In addition, in order to confirm the details of the expected gene silencing results, all of the cell samples were harvested and related cell lysates were prepared for the further fluorescent analysis. To our delight, the trend of observed fluorescent intensity was consistent with the results shown in cell images (Fig. 5I). Similarly, during the course of gene silence, the cytotoxicity of applied photoactive Si-UCNPs–siRNA conjugates was also investigated by MTT assay. As shown in Fig. 5J, the cell viability observed in the samples with NIR light irradiation was quite similar with those treated with siRNA/Lipo2k. All of these results unequivocally suggested that the developed photocaged Si–UCNPs conjugate could work as a safe and reliable nanoplatform for the NIR-light controllable gene and siRNA delivery. Apart from the effective siRNA delivery to silence EGFP gene expression, such simple nanoparticle delivery platform would be easily extended to transport other therapeutic oligonucleotides without chemical modification of target nucleic acids structures.
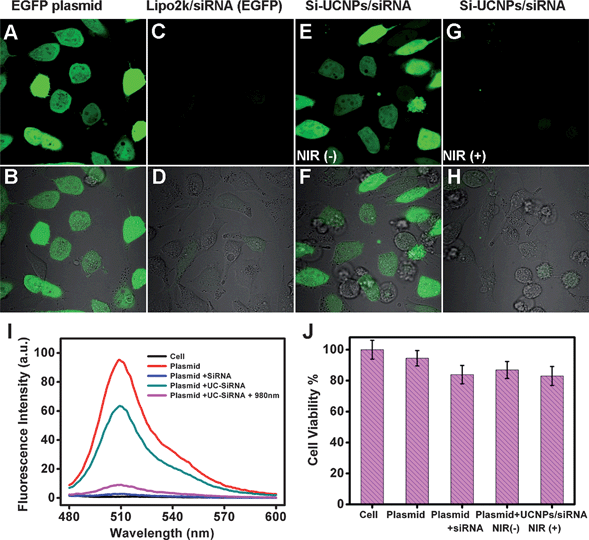 |
| Fig. 5 (A) Fluorescence and (B) overlapped bright field cell image of HeLa cells treated with EGFP plasmid; cells were treated with Lipo2k/siRNA after transferred with EGFP plasmids: (C) fluorescence and (D) overlapped bright field cell image; cells were treated with UCNPs–siRNA no light treatment after transferred with EGFP plasmids: (E) fluorescence and (F) overlapped bright field cell image; cells were treated with UCNPs–siRNA with NIR light irradiation after transferred with EGFP plasmids: (G) fluorescence and (H) overlapped bright field cell image. For (I), fluorescence spectra of the cells with different treatments; and (J), cell viability treated with EGFP plasmids, EGFP plasmids + siRNA/Lipo2k, EGFP plasmids + UCNPs–siRNA, EGFP plasmids + UCNPs–siRNA + NIR light. | |
4. Conclusions
In summary, we have developed effective strategies for targeted siRNA delivery by using photocaged silica coated UCNPs as the nanocarriers. The uploading of negatively charged siRNA to photocaged silica coated UCNPs can be easily achieved through the complementary electrostatic interactions caused by the cationic linkers on particles surface. After cellular uptake and followed by 980 nm light exposure, the functionalized Si-UCNPs could emit UV light which effectively triggered siRNA photorelease from UCNPs platform. Compared to the existing carrier systems for UV-controlled siRNA delivery, the use of unique NIR-to-UV property of UCNPs would result in negligible phototoxicity and guarantee high tissue penetration by NIR irradiation. Most importantly, the siRNA delivered here is physically adsorbed on the UCNPs surface. The NIR irradiation released siRNA inside cells retains good biological-activity in the RNA interference pathway, which has been confirmed by gene silencing tests. The multiple advantages of cationic functionalized UCNPs in gene delivery, cell imaging and potential therapeutic applications, combined with recent breakthroughs,58–60 could make it serve as an outstanding template in future biomedical research and therapeutic applications.
Acknowledgements
This work was supported by Start-Up Grant (SUG), MOE, Tier 1 (RG 64/10) in Nanyang Technological University, Singapore.
Notes and references
- Y. Dorsett and T. Tuschl, Nat. Rev. Drug Discovery, 2004, 3, 318–329 CrossRef CAS.
- Y. Singh, P. Murat and E. Defrancq, Chem. Soc. Rev., 2010, 39, 2054–2070 RSC.
- R. L. Juliano, X. Ming and O. Nakagawa, Bioconjugate Chem., 2011, 23, 147–157 CrossRef.
- A. de Fougerolles, H.-P. Vornlocher, J. Maraganore and J. Lieberman, Nat. Rev. Drug Discovery, 2007, 6, 443–453 CrossRef CAS.
- D. M. Dykxhoorn and J. Lieberman, Cell, 2006, 126, 231–235 CrossRef CAS.
- T. Lobovkina, G. B. Jacobson, E. Gonzalez-Gonzalez, R. P. Hickerson, D. Leake, R. L. Kaspar, C. H. Contag and R. N. Zare, ACS Nano, 2011, 5, 9977–9983 CrossRef CAS.
- S. J. Tan, P. Kiatwuthinon, Y. H. Roh, J. S. Kahn and D. Luo, Small, 2011, 7, 841–856 CrossRef CAS.
- M. Sioud and A. Mobergslien, Bioconjugate Chem., 2012, 23, 1040–1049 CrossRef CAS.
- A. Agrawal, D.-H. Min, N. Singh, H. Zhu, A. Birjiniuk, G. von Maltzahn, T. J. Harris, D. Xing, S. D. Woolfenden, P. A. Sharp, A. Charest and S. Bhatia, ACS Nano, 2009, 3, 2495–2504 CrossRef CAS.
- A. Paganin-Gioannii, E. Bellard, B. Couderc, J. Teissié and M. Golzio, J. RNAi Gene Silencing, 2008, 4, 281–288 Search PubMed.
- G. Liu, K. Y. Choi, A. Bhirde, M. Swierczewska, J. Yin, S. W. Lee, J. H. Park, J. I. Hong, J. Xie, G. Niu, D. O. Kiesewetter, S. Lee and X. Chen, Angew. Chem., Int. Ed., 2012, 51, 445–449 CrossRef CAS.
- S. S. Dunn, S. Tian, S. Blake, J. Wang, A. L. Galloway, A. Murphy, P. D. Pohlhaus, J. P. Rolland, M. E. Napier and J. M. DeSimone, J. Am. Chem. Soc., 2012, 134, 7423–7430 CrossRef CAS.
- J. Beloor, C. S. Choi, H. Y. Nam, M. Park, S. H. Kim, A. Jackson, K. Y. Lee, S. W. Kim, P. Kumar and S.-K. Lee, Biomaterials, 2012, 33, 1640–1650 CrossRef CAS.
- W. H. Kong, K. H. Bae, C. A. Hong, Y. Lee, S. K. Hahn and T. G. Park, Bioconjugate Chem., 2011, 22, 1962–1969 CrossRef CAS.
- H.-K. Na, M.-H. Kim, K. Park, S.-R. Ryoo, K. E. Lee, H. Jeon, R. Ryoo, C. Hyeon and D.-H. Min, Small, 2012, 8, 1752–1761 CrossRef CAS.
- X. Li, Q. R. Xie, J. Zhang, W. Xia and H. Gu, Biomaterials, 2011, 32, 9546–9556 CrossRef CAS.
- A. K. R. Lytton-Jean, R. Langer and D. G. Anderson, Small, 2011, 7, 1932–1937 CrossRef CAS.
- D. P. Ferris, Y. L. Zhao, N. M. Khashab, H. A. Khatib, J. F. Stoddart and J. I. Zink, J. Am. Chem. Soc., 2009, 131, 1686–1688 CrossRef CAS.
- B. J. Heilman, J. St. John, S. R. J. Oliver and P. K. Mascharak, J. Am. Chem. Soc., 2012, 134, 11573–11582 CrossRef CAS.
- L. Sun, Y. Yang, C.-M. Dong and Y. Wei, Small, 2011, 7, 401–406 CrossRef CAS.
- G. Han, C. C. You, B. J. Kim, R. S. Turingan, N. S. Forbes, C. T. Martin and V. M. Rotello, Angew. Chem., Int. Ed., 2006, 45, 3165–3169 CrossRef CAS.
- Q. Shao, T. Jiang, G. Ren, Z. Cheng and B. Xing, Chem. Commun., 2009, 4028–4030 RSC.
- P. D. Thornton and A. Heise, J. Am. Chem. Soc., 2010, 132, 2024–2028 CrossRef CAS.
- G. Mayer and A. Heckel, Angew. Chem., Int. Ed., 2006, 45, 4900–4921 CrossRef CAS.
- Q. Shao and B. Xing, Chem. Soc. Rev., 2010, 39, 2835–2846 RSC.
- S. Sortino, J. Mater. Chem., 2012, 22, 301–318 RSC.
- Y. T. Chang, P. Y. Liao, H. S. Sheu, Y. J. Tseng, F. Y. Cheng and C. S. Yeh, Adv. Mater., 2012, 24, 3309–3314 CrossRef CAS.
- X. J. Yang, X. Liu, Z. Liu, F. Pu, J. S. Ren and X. G. Qu, Adv. Mater., 2012, 24, 2890–2895 CrossRef CAS.
- L.-L. Li, R. Zhang, L. Yin, K. Zheng, W. Qin, P. R. Selvin and Y. Lu, Angew. Chem., 2012, 124, 6225–6229 CrossRef.
- J. Zhou, Z. Liu and F. Li, Chem. Soc. Rev., 2012, 41, 1323–1349 RSC.
- F. Wang and X. Liu, Chem. Soc. Rev., 2009, 38, 976–989 RSC.
- J.-C. Boyer, L. A. Cuccia and J. A. Capobianco, Nano Lett., 2007, 7, 847–852 CrossRef CAS.
- K. A. Abel, J.-C. Boyer and F. C. J. M. van Veggel, J. Am. Chem. Soc., 2009, 131, 14644–14645 CrossRef CAS.
- J. Shen, L.-D. Sun and C.-H. Yan, Dalton Trans., 2008, 5687–5697 RSC.
- F. Wang, D. Banerjee, Y. Liu, X. Chen and X. Liu, Analyst, 2010, 135, 1839–1854 RSC.
- P. Zhang, W. Steelant, M. Kumar and M. Scholfield, J. Am. Chem. Soc., 2007, 129, 4526–4527 CrossRef CAS.
- G. Chen, T. Y. Ohulchanskyy, R. Kumar, H. Agren and P. N. Prasad, ACS Nano, 2010, 4, 3163–3168 CrossRef CAS.
- C. J. Carling, F. Nourmohammadian, J. C. Boyer and N. R. Branda, Angew. Chem., Int. Ed., 2010, 49, 3782–3785 CrossRef CAS.
- H. S. Qian, H. C. Guo, P. C.-L. Ho, R. Mahendran and Y. Zhang, Small, 2009, 5, 2285–2290 CrossRef CAS.
- B. Yan, J. C. Boyer, N. R. Branda and Y. Zhao, J. Am. Chem. Soc., 2011, 133, 19714–19717 CrossRef CAS.
- J.-C. Boyer, C.-J. Carling, B. D. Gates and N. R. Branda, J. Am. Chem. Soc., 2010, 132, 15766–15772 CrossRef CAS.
- Y. M. Yang, Q. Shao, R. R. Deng, C. Wang, X. Teng, K. Cheng, Z. Cheng, L. Huang, Z. Liu, X. G. Liu and B. G. Xing, Angew. Chem., Int. Ed., 2012, 51, 3125–3129 CrossRef CAS.
- M. K. G. Jayakumar, N. M. Idris and Y. Zhang, Proc. Natl. Acad. Sci. U. S. A., 2012, 109, 8483–8488 CrossRef CAS.
- Y. M. Yang, J. X. Aw, K. Chen, F. Liu, P. Padmanabhan, Y. L. Hou, Z. Cheng and B. G. Xing, Chem.–Asian J., 2011, 6, 1381–1389 CrossRef CAS.
- Y. Piao, A. Burns, J. Kim, U. Wiesner and T. Hyeon, Adv. Funct. Mater., 2008, 18, 3745–3758 CrossRef CAS.
- A. Guerrero-Martinez, J. Perez-Juste and L. M. Liz-Marzan, Adv. Mater., 2010, 22, 1182–1195 CrossRef CAS.
- F. Wang, Y. Han, C. S. Lim, Y. Lu, J. Wang, J. Xu, H. Chen, C. Zhang, M. Hong and X. Liu, Nature, 2010, 463, 1061–1065 CrossRef CAS.
- S. Santra, R. P. Bagwe, D. Dutta, J. T. Stanley, G. A. Walter, W. Tan, B. M. Moudgil and R. A. Mericle, Adv. Mater., 2005, 17, 2165–2169 CrossRef CAS.
- C.-W. Lai, Y.-H. Wang, C.-H. Lai, M.-J. Yang, C.-Y. Chen, P.-T. Chou, C.-S. Chan, Y. Chi, Y.-C. Chen and J.-K. Hsiao, Small, 2008, 4, 218–224 CrossRef CAS.
- F. Lu, S.-H. Wu, Y. Hung and C.-Y. Mou, Small, 2009, 5, 1408–1413 CrossRef CAS.
- S. Zhang, J. Li, G. Lykotrafitis, G. Bao and S. Suresh, Adv. Mater., 2009, 21, 419–424 CrossRef CAS.
- C. Wang, H. Tao, L. Cheng and Z. Liu, Biomaterials, 2011, 32, 6145–6154 CAS.
- M. K. Yu, Y. Y. Jeong, J. Park, S. Park, J. W. Kim, J. J. Min, K. Kim and S. Jon, Angew. Chem., Int. Ed., 2008, 47, 5362–5365 CrossRef CAS.
- S. V. Boddapati, G. G. M. D'Souza, S. Erdogan, V. P. Torchilin and V. Weissig, Nano Lett., 2008, 8, 2559–2563 CrossRef CAS.
- X. Zheng, U. W. Sallum, S. Verma, H. Athar, C. L. Evans and T. Hasan, Angew. Chem., Int. Ed., 2009, 48, 2148–2151 CrossRef CAS.
- S. D. Brown, P. Nativo, J.-A. Smith, D. Stirling, P. R. Edwards, B. Venugopal, D. J. Flint, J. A. Plumb, D. Graham and N. J. Wheate, J. Am. Chem. Soc., 2010, 132, 4678–4684 CrossRef CAS.
- J.-C. Zhou, Z.-L. Yang, W. Dong, R.-J. Tang, L.-D. Sun and C.-H. Yan, Biomaterials, 2011, 32, 9059–9067 CrossRef CAS.
- F. Wang, R. Deng, J. Wang, Q. Wang, Y. Han, H. Zhu, X. Chen and W. Liu, Nat. Mater., 2011, 10, 968–973 CrossRef CAS.
- W. Zou, C. Visser, J. A. Maduro, M. S. Pshenichnikov and J. C. Hummelen, Nat. Photonics, 2012, 6, 560–564 CrossRef CAS.
- X. Xie and X. Liu, Nat. Mater., 2012, 11, 842–843 CrossRef CAS.
|
This journal is © The Royal Society of Chemistry 2013 |
Click here to see how this site uses Cookies. View our privacy policy here.