DOI:
10.1039/C2NR32248J
(Paper)
Nanoscale, 2013,
5, 225-230
Preparation of graphite-like carbon nitride nanoflake film with strong fluorescent and electrochemiluminescent activity†
Received
12th August 2012
, Accepted 18th October 2012
First published on 23rd October 2012
Abstract
The preparation, characterization, fluorescence (FL) and electrochemiluminescence (ECL) of graphite-like carbon nitride nanoflake particles (g-C3N4 NFPs) and nanoflake films (g-C3N4 NFFs) have been reported. Highly water-dispersible g-C3N4 NFPs with a height of ∼5 to 35 nm and a lateral dimension of ∼40 to 220 nm have been extracted from bulk g-C3N4 materials by chemical oxidation. New, stable and defined g-C3N4 NFFs can be easily obtained by drying NFPs on certain hydrophilic substrates such as glass or electrode surfaces. Both g-C3N4 NFPs and g-C3N4 NFFs have good FL activities, i.e. they can give strong blue light (435 nm) emission under UV light (365 nm) excitation. The as-prepared g-C3N4 NFFs on a glassy carbon electrode exhibit strong non-surface state ECL activity in the presence of reductive–oxidative coreactants, including dissolved oxygen (O2), hydrogen peroxide (H2O2) and peroxydisulfate (S2O82−) and give rise to blue light emission (435 nm), which is the same as the wavelength of FL. The non-surface state ECL mechanisms of the g-C3N4 NFF–coreactant systems have been studied and discussed in detail.
1. Introduction
Carbon nitride materials have experienced a renaissance of activity in recent years due to their unique properties such as high hardness, wear resistance, chemical inertness, wide-band optical transparency, stable electron field emission, and especially biocompatibility.1–3 They can exist in several allotropes that have diverse properties, however, the graphite phase carbon nitride (g-C3N4) with the tris-s-triazine tectonic unit is regarded as the most stable allotrope at ambient conditions, and has the smallest direct band gap (2.7 eV) due to the π-conjugated graphitic planes formed by the sp2 hybridization of carbon and nitrogen.4 g-C3N4 has shown several potential applications as a metal-free catalyst for Friedel–Craft reactions,5 as a reactant for metal nitride nanoparticle synthesis,6 and as a photocatalyst for hydrogen production.7
It has been found that the nano-sized g-C3N4 not only provides a high surface area but also improves the performance of the material.8 Accordingly, growing attention has been paid on the synthesis of the nano-sized g-C3N4. To date, the nano-sized g-C3N4 was usually obtained by the so-called hard-template technique using expensive mesoporous silica,4 porous anodic alumina oxide (AAO) membranes,9 and layered clays.10 The preparation of the nano-sized g-C3N4 without using a template has not been reported yet. In this work, we have prepared highly water-dispersible g-C3N4 nanoflake particles (NFPs) by an up-down method, i.e. by chemically oxidizing the bulk g-C3N4 with nitric acid. This method shows many advantages, including low cost, low-temperature operation, and simple experimental performance, and thus would be important in the large-scale production and extensive application of nano-sized g-C3N4. Additionally, it has been found that g-C3N4 NFPs can be easily made into g-C3N4 nanoflake films (NFFs) by drying the g-C3N4 NFP suspension on certain substrates. The as-prepared g-C3N4 exhibits good fluorescent and electrochemiluminescent properties, suggesting their promising applications in display devices and chemical sensors.
2. Experimental
2.1. Chemicals
Dicyanamide was purchased from Sigma. Potassium persulfate (K2S2O8) and hydrogen peroxide (H2O2) were obtained from Fuchen Chemical Reagent Co. (Tianjin, China). All other reagents were of analytical reagent grade and used without further purification. The 0.1 M pH 7.0 phosphate buffer solution (PBS) was used as the electrolyte. Doubly distilled water was used throughout the work.
2.2. Instrumentation
The preparation of bulk g-C3N4 was carried out in a tube furnace (GSL 1400X, Kejing Materials Technology Lt. Co., Hefei, China). Atomic force microscopy (AFM) images were obtained by tapping-mode on a Nanoscope IIIa Digital Instruments with NSC15 tips (silicon cantilever, MikroMasch). X-Ray power diffraction (XRD) patterns were measured with a Japan Rigaku D/max-3C using Cu Kα radiation. To investigate the optical properties of the g-C3N4 NFP aqueous solution, the photoluminescent spectrum was recorded on a fluorescence spectrophotometer (Cary Eclipse, Varian). Both the FL spectrum of the g-C3N4 bulk material and the ECL spectra of g-C3N4 NFFs were recorded with a steady/transient state fluorescence spectrophotometer (Edinburgh Instruments, FLS920). UV-vis absorption was characterized by a UV/vis/NIR spectrophotometer (Lambda 750). Elemental analysis for g-C3N4 bulk material and nanoflakes were performed on an organic elemental analyzer (Vario MICRO). The field emission scanning electron microscope (FE SEM) image of the g-C3N4 NFF coated on a glassy carbon (GC) electrode was captured by using a Nova NanoSEM 230 field-emission microscope (FEI, USA). Electrochemical (EC) and ECL measurements were carried out on an EC and ECL detection system (MPI-E, Remex Electronic Instrument Lt. Co., Xi'an, China) equipped with a three-electrode system, i.e. a GC disc working electrode (0.0707 cm2), a Pt wire counter electrode, and a Ag/AgCl (3 M KCl) reference electrode.
2.3. Synthesis of g-C3N4 NFPs
First, the g-C3N4 bulk material was prepared following the previously reported literature.7 Briefly, the g-C3N4 was prepared by heating dicyanamide for 4 h to 550 °C and kept at this temperature for another 4 h in air. Then the g-C3N4 NFPs were synthesized as follows: (1) 1 g of bulk g-C3N4 powder was ground well with a mortar and a pestle and was then put into 100 mL 5 M HNO3 and refluxed for 24 h. After naturally cooling to room temperature, no fluorescence was found in the transparent supernatant due to the poor dispersability of g-C3N4 NFPs in highly acidic solution. (2) The refluxed product was in turn centrifuged (2770g) and washed with doubly distilled water four times. The supernatant was collected until its pH reached 7 and concentrated on a rotary evaporator at 60 °C under reduced pressure, resulting in a milk-like suspension. The mass concentration of the g-C3N4 NFP suspension was calculated by weighing the powder dried from a certain volume of the suspension.
2.4. Preparation of g-C3N4 NFFs
Before preparing the g-C3N4 NFFs, substrates including glass slides and GC electrodes were thoroughly cleaned. The glass substrates were cleaned in turn with HCl, NaOH, H2O, and ethanol, while the GC electrodes (3.0 mm in diameter) were polished in turn with 0.3 and 0.05 μm aluminum slurry, rinsed thoroughly with redistilled water, and then sonicated in doubly distilled water for 2 min. A certain volume of g-C3N4 NFP suspension (1 mg mL−1) was dropped on the cleaned substrates and resulted in the formation of stable g-C3N4 NFFs on the glass slides and GC electrodes by drying the g-C3N4 NFP suspension at room temperature in the air.
3. Results and discussion
3.1. Morphology and FL properties of g-C3N4 NFPs and g-C3N4 NFFs
The concentrated g-C3N4 NFP suspension is a milk-like suspension (see photo (b) in Fig. 1A) and it is very stable (i.e. it can keep unchanged for more than 6 months under room temperature). In contrast, the untreated g-C3N4 bulk material cannot be dispersed in aqueous solution to form a homogenous suspension (see photo (a) in Fig. 1A). That the g-C3N4 NFPs exhibit highly water-dispersible might result from the formation of –COO− and –NH3+ on the surface of g-C3N4 NFPs by acid treatment. This viewpoint is supported by the elemental analysis results which show that oxygen and hydrogen contents are increased after chemical oxidation (data not shown). The diluted g-C3N4 NFPs suspension (photo (c) in Fig. 1A) shows bright blue luminescence under excitation with a 365 nm UV light (photo (d) in Fig. 1A), with a fluorescence quantum yield (FLQY) of 3.0% (see the ESI†). The FLQY of the as-prepared NFPs is similar to those of carbon quantum dots without good surface passivation.11–13 The XRD patterns of the g-C3N4 bulk material (curve (e) in Fig. 1B) and g-C3N4 NFPs (curve (f) in Fig. 1B) are compared in Fig. 1B. It is apparent, that there is no evident different in XRD patterns between the bulk and NFP materials, indicating that the chemical oxidation treatment with concentrated HNO3 does not destroy the structure of carbon nitrides, i.e. the graphite-like structure of g-C3N4 is retained after chemical oxidation. Although there is no evident change in the structure of g-C3N4, the FL peak of g-C3N4 is blue-shifted from 465 nm associated with bulk material (see curve (g) in Fig. 1C) to 435 nm associated with g-C3N4 NFPs (see curve (h) in Fig. 1C) after chemical treatment, suggesting a significant change in particle size.14 Additionally, g-C3N4 NFPs in aqueous solution has a large UV absorption peak at 296 nm and a shoulder absorption band around 365 nm (see curve (i) in Fig. 1C). Further particle size characterization for the obtained g-C3N4 NFPs were carried out by SEM and AFM (see Fig. 2). The SEM images obtained for g-C3N4 NFPs deposited on the surface of GC electrode (sections A and B in Fig. 2) indicate that g-C3N4 NFPs are nanoflakes with an average size less than 100 nm. The AFM data clearly show that g-C3N4 NFPs are nanoflakes with a height of ∼5 to 35 nm and a lateral dimension of ∼40 to 220 nm (see sections C and D in Fig. 2). Furthermore, the SEM images present a closely packed assembly of g-C3N4 NFPs, suggesting that thin g-C3N4 nanoflake films (i.e. g-C3N4 NFFs) can be formed easily on substrates such as the surface of the GC electrode.
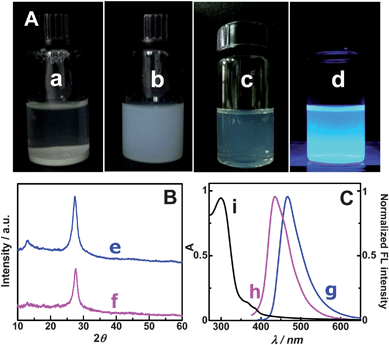 |
| Fig. 1 Comparison of g-C3N4 nanoflake particles (NFPs) with g-C3N4 bulk material. (A) The dispersion of g-C3N4 bulk material (a) and concentrated g-C3N4 NFPs (b) in water, and the photos of diluted g-C3N4 NFP solution under white light (c) and 365 nm UV light (d); (B) XRD patterns of g-C3N4 bulk material (e) and g-C3N4 NFPs (f); (C) FL emission spectra of g-C3N4 bulk material (g) and g-C3N4 NFPs (h), and UV-vis spectrum of g-C3N4 NFPs in aqueous solution (i). | |
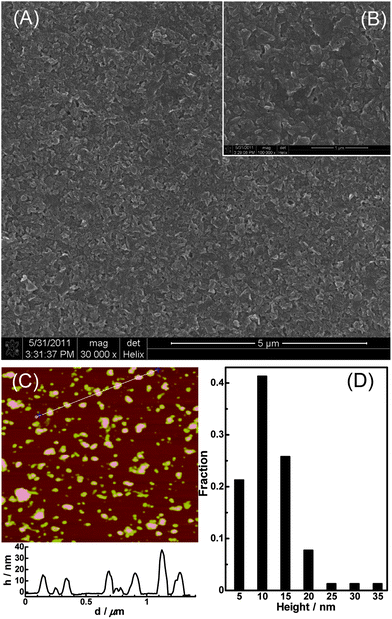 |
| Fig. 2 SEM images of g-C3N4 NFPs film on a glassy carbon substrate with scale bar of 5 μm (A) and 1 μm (B), and AFM image of g-C3N4 NFPs (C) and the statistical height distribution of g-C3N4 NFPs based on the measurement of 226 particles (D). | |
The excellent film-forming ability of the g-C3N4 NFPs was verified by the following experiments: the g-C3N4 NFP solution was dropped on the cleaned glass slides or glass disk templates and kept dry at room temperature in the air. It was found that a white, defined (without any cracks), and semitransparent film (i.e. g-C3N4 NFF) was coated on the glass surface (see Fig. 3A), exhibiting an excellent film-forming ability of the g-C3N4 NFPs. The as-prepared g-C3N4 NFFs can give bright blue FL emission when exposed to a UV light of 365 nm (see Fig. 3B). Interestingly, the g-C3N4 NFFs are very stable in aqueous solution as soon as the dry films are prepared from the g-C3N4 NFPs. The latter are highly water-dispersible (see Fig. 1). The shape and FL intensity of g-C3N4 NFFs coated on a glass template, and immersed in doubly distilled water can remain unchanged for more than 30 days (see sections C and D in Fig. 3). It is still not clear why the g-C3N4 NFFs do not dissolve back into g-C3N4 NFPs. Probably, the excellent stability of the g-C3N4 NFFs results from some interactions between –COOH and NH2 group at the surfaces of the g-C3N4 NFPs, for example, hydrogen bond interaction, or formation of –CONH– linkage by dehydration during drying of the g-C3N4 NFPs. The effects of environmental conditions such as metal ions, ionic strength and pH on the stability of g-C3N4 NFFs were investigated (Fig. S1–S3†). The g-C3N4 NFFs coated on glass substrates can remain stable under the above different environmental conditions, although its FL activity may be quenched by some metal ions such as copper(II) ion (Fig. S1†), high ionic strength (Fig. S2†) and high pH (Fig. S3†). Moreover, the quenched FL can be reversibly restored by rinsing the g-C3N4 NFFs with pure water. It is apparent that the high stability of the as-prepared g-C3N4 nanoflake films provide an easy and effective way for the development of g-C3N4 NFF-based sensors, display devices and photocatalysts.
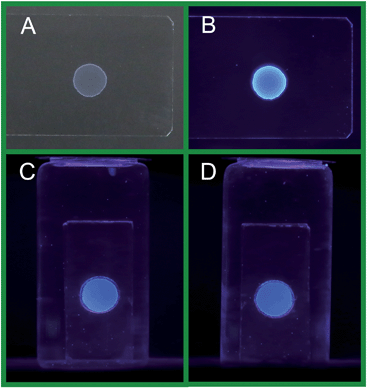 |
| Fig. 3 The photos of the g-C3N4 NFFs on the glass substrate exposed to air under a white light (A), and under a 365 nm UV light (B); the fluorescence photos (under 365 nm UV light excitation) obtained for the g-C3N4 NFF on a glass disk template as soon as it was immersed in the aqueous solution (C), and after it was immersed in the aqueous solution for 30 days (D). | |
3.2. Electrochemiluminescent (ECL) properties of g-C3N4 NFFs
3.2.1. ECL of g-C3N4 NFFs in the presence of dissolved O2.
ECL,15,16 and electrochemical responses of g-C3N4 NFFs at GC electrode in air-saturated aqueous solution (pH 7.0 PBS) were investigated simultaneously, and the experimental results were shown in Fig. 4 (see curves (c) and (c′)). In the cathodic polarization process, two irreversible cathodic waves with peaks at −0.65 V and −1.33 V were observed for the g-C3N4 NFF-modified GC electrode (Fig. 4c). The cathodic wave with a peak at −0.65 V can be attributed to the electrochemical reduction of dissolved O2 for it disappears after the removal of oxygen from the solution (see Fig. 4b). The peak at −1.33 V can be assigned to the electrochemical reduction of g-C3N4 NFFs, as this cathodic wave cannot be observed in the absence of g-C3N4 NFFs (Fig. 4a). The simultaneously recorded ECL response shows that a strong ECL signal appears when the potential is more negative than the onset potential (−0.83 V) of the reduction potential of g-C3N4 NFFs, and reaches a maximum ECL intensity at −1.07 V (Fig. 4c′), suggesting that electron injection into the g-C3N4 NFF semiconductor is essential for the ECL of g-C3N4 NFFs. It should be noted here, neither the redox peak nor the ECL response can be observed for the g-C3N4 NFFs in the positive potential range from 0 to 1.2 V (before the electrolysis of water), indicating that the g-C3N4 NFFs are stable in the positive potential range, and suggesting that the initial potential of cathodic polarization can be set at a random potential between 0 and 1.2 V (e.g. 0 V or +1.0 V).
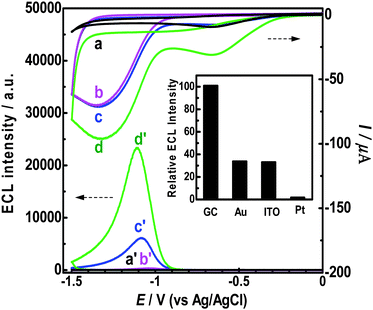 |
| Fig. 4 Simultaneous ECL and chemical responses of g-C3N4 NFFs on GC electrode in various aqueous solutions: (a) bare GC electrode in the air-saturated solution; (b) g-C3N4 NFF-modified GCE in the N2-saturated solution; (c) g-C3N4 NFF-modified GCE in the air-saturated solution; (d) g-C3N4 NFF-modified GCE in O2-saturated solution. All aqueous solutions contained 0.1 M and pH 7.0 PBS. The potential scan rates were all 100 mV s−1. Inset: comparison of ECL intensities at different electrode materials (GC, Au, ITO and Pt) with the same area (0.0707 cm2). | |
It has been revealed that dissolved O2 is necessary for the ECL, since no ECL signal can be observed after the dissolved O2 is removed from the air-saturated solution by N2 (see Fig. 4b′), and a much higher ECL intensity can be produced if the aqueous solution is saturated by pure O2 (see Fig. 4d′). In other words, dissolved O2 serves as a coreactant in the ECL of g-C3N4 NFFs. Additionally, besides on the GC electrode, the g-C3N4 NFFs also show good ECL activities on Au and indium tin oxide (ITO) electrodes (inset in Fig. 4). However, the g-C3N4 NFFs gave very weak ECL emission on the Pt electrode, due to an unknown ECL mechanism.
3.2.2. ECL of g-C3N4 NFFs in the presence H2O2 and S2O82−.
Besides the above-mentioned dissolved O2, other reductive–oxidative reagents, such as H2O2 and S2O82− can also act as coreactants of the g-C3N4 NFF ECL (see Fig. 5b and c). For comparing the ECL activities of dissolved O2, H2O2 and S2O82−, the concentrations of H2O2 and S2O82− were controlled to be 0.258 mM, which equals the concentration of dissolved O2 in an air-saturated solution. From Fig. 5, it is known that the coreactant ECL activity of H2O2 is slightly higher than that of dissolved O2, while the coreactant ECL activity of S2O82− is much (20–27 times) higher than those of dissolved O2 and H2O2. Although the ECL signals of the g-C3N4 NFF–O2 and the g-C3N4 NFF–H2O2 systems are obviously weaker than that of the g-C3N4 NFF–S2O82− system, they are sensitive enough and may have some promising applications in novel biosensors since O2 and H2O2 are well known reactants or products in various enzymatic reactions. Additionally, the as-prepared g-C3N4 NFFs can give much (ca. 65 times) higher ECL intensity than previously reported carbon quantum dots (CQDs),17 in the presence of the same concentration of S2O82− (see Fig. S4 in the ESI†), indicating that g-C3N4 NFFs are new nano-sized luminophores with very strong ECL activity.
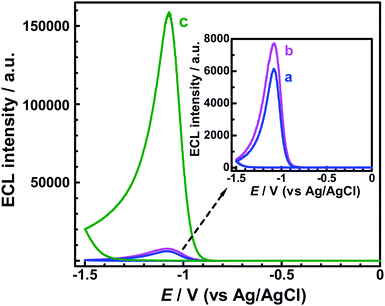 |
| Fig. 5 ECL responses obtained for g-C3N4 NFF-modified GC electrodes in various aqueous solutions: (a) air-saturated PBS (with concentration of ca. dissolved 0.258 mM O2); (b) 0.258 mM H2O2 (free of dissolved O2); and (c) 0.258 mM K2S2O8 (free of dissolved O2). All the aqueous solutions contained 0.1 M PBS (pH 7.0), and the potential scan rates were all 100 mV s−1. | |
3.2.3. Non-surface state ECL mechanism of g-C3N4 NFF–coreactant systems.
It has been reported that ECL of many semiconductor nanoparticles (NPs), such as Si NPs,18 CdSe/ZnSe NPs,19 Ge NPs,20 and CQDs,17,21,22 result from electron transfer in surface states. In the surface state ECL, the maximum ECL wavelengths are usually red-shifted compared with the maximum FL wavelengths, and the ECL intensities are very sensitive to surface passivation.22 It is of considerable research interest to know whether the ECL of g-C3N4 NFF consisting of g-C3N4 nanoflakes is associated with the surface states or not. In other words, is there any difference in wavelength between the FL and ECL of g-C3N4 nanomaterials?
The ECL spectrum of g-C3N4 NFFs was obtained by using S2O82− as the coreactant, since the g-C3N4 NFF–S2O82− ECL system could give stable and sufficiently strong ECL emission upon applying continuous pulse potentials. The pulse potentials were switched between −1.0 V (where the maximum ECL signal can be reached) and +1.0 V (where g-C3N4 NFFs is stable). A pulse period of 2 s was chosen, i.e. 1 s for application of +1.0 V and 1 s for application of −1.0 V, since a very stable ECL pulse signal could be obtained under the above pulse potential conditions. The obtained ECL spectrum is shown in Fig. 6B, which indicates that the maximum emission wavelength of the g-C3N4 NFF ECL system is ca. 435 nm. The ECL intensity was strong enough to allow direct observation of blue light emission on the surface of the GCE electrode surface (see inset of Fig. 6B). For comparison, the FL spectrum and FL photo of g-C3N4 NFPs is also shown in Fig. 6 (see section A). Apparently, the comparison of Fig. 6A and B suggests that the shape and the maximum wavelength of the ECL spectrum of the g-C3N4 NFF–coreactant system are almost the same as those of the FL spectrum of g-C3N4 nanoflakes. It is apparent, on the basis of above-mentioned luminescent spectra, that we can draw following two conclusions: (1) g-C3N4 NFFs serve as the luminophores in ECL reactions; (2) the ECL emission is associated with electron transfer in the core of the nanomaterials (i.e. g-C3N4 NFFs) rather than their surface states. In other word, the ECL of g-C3N4 the NFF–coreactant system is a non-surface state ECL.
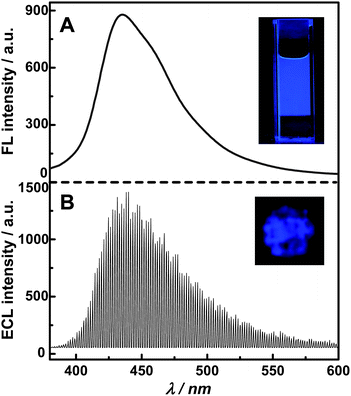 |
| Fig. 6 Comparison of FL (A) and ECL (B) spectra of g-C3N4 nanostructures. The experimental conditions for FL spectrum: the concentration of g-C3N4 NFP aqueous solution was 15 μg mL−1; excitation wavelength was 360 nm. The experimental conditions for ECL spectrum: the continuous pulse potential was switched between +1 V (for 1 s) and −1 V (for 1 s); the testing solution contained 0.15 M K2S2O8 and 0.6 M PBS (pH 7.0). Inset in part A: the FL photo of the g-C3N4 NFP aqueous solution illuminated by a UV beam of 365 nm. Inset in part B: the ECL image of the g-C3N4 NFF modified GC electrode. | |
According to the above investigation, the ECL mechanisms of the g-C3N4 NFF–coreactant systems can be proposed in Fig. 7. The ECL reactions may involve four processes, i.e. electron injection, hole donor production, hole injection, and electron–hole annihilation producing ECL emission. The energy of the electron on GCE is raised with the cathodic polarization of potential, and is high enough to be injected into the conducting bands (CB) of g-C3N4 NFF:
| g-C3N4 + e− → g-C3N4˙− | (R1) |
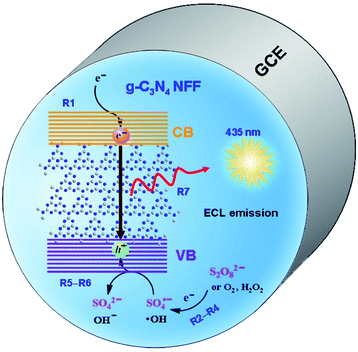 |
| Fig. 7 Proposed ECL reaction mechanism for g-C3N4 NFF–coreactant systems. | |
Meanwhile, hole donors (i.e. free radicals) are produced from the reductive–oxidative coreactants, such as dissolved O2, H2O2 and S2O82−:
| O2 + 2H2O + 2e− → 2H2O2 | (R2) |
| H2O2 + e− → OH− + ˙OH | (R3) |
| S2O82− + e− → SO42− + SO4˙− | (R4) |
The holes are subsequently injected into the valence bands (VB) of g-C3N4 NFF by the interaction of the free radicals (˙OH or SO4˙−) with g-C3N4 NFF:
| ˙OH + g-C3N4˙− → g-C3N4* + OH− | (R5) |
| SO4˙− + g-C3N4˙− → g-C3N4* + SO42− | (R6) |
Finally, the electrons injected in the conducting band annihilates with the holes in the valence band to form the excited state of the g-C3N4 nanoflakes that emits blue light when they go down to their ground states:
| g-C3N4* → g-C3N4 + hν | (R7) |
4. Conclusions
Highly water-dispersible g-C3N4 nanoflake particles (i.e. g-C3N4 NFPs) with a height of ∼5 to 35 nm and a lateral dimension of ∼40 to 220 nm has been extracted from the g-C3N4 bulk material by chemical oxidation. The g-C3N4 NFPs exhibit excellent film-forming ability, on the basis of which new, defined and stable g-C3N4 nanoflake films (i.e. g-C3N4 NFPs) can be easily prepared on hydrophilic substrates such as glass slides and GCE surfaces. Both g-C3N4 NFPs and g-C3N4 NFFs have good FL activities, giving strong blue light (435 nm) emission under UV light (365 nm) excitation. The g-C3N4 NFFs on a glassy carbon electrode exhibit strong ECL activity in the presence of reductive–oxidative coreactants including dissolved O2, H2O2 and S2O82− and give rise to blue light emission (435 nm). The ECL can be attributed to a non-surface sate ECL since the shape of the ECL spectrum and the maximum ECL emission wavelength are almost the same as those of FL spectrum. The ECL mechanisms of the g-C3N4 NFF–coreactant systems are proposed to involve the following processes: electron injection into the conducting bands of g-C3N4 NFFs; electrogeneration of hole donors (˙OH or SO4˙−) on the electrode; hole injection into the valence bands of g-C3N4 NFFs; and the annihilation of electron–hole pairs to produce ECL emission. The excellent properties of g-C3N4 NFFs, such as easy preparation, excellent stability in solution, good FL activities, and high ECL sensitivity towards dissolved O2, H2O2, and S2O82− enable the new g-C3N4 NFFs nanomaterials to have promising applications in biosensors, the study of free radicals, and new display devices.
Acknowledgements
This study was financially supported by National Natural Science Foundation of China (21075018), the Program for New Century Excellent Talents in Chinese University (NCET-10-0019), the National Basic Research Program of China (2010CB732400), and the Program for Changjiang Scholars and Innovative Research Team in University (no. IRT1116).
Notes and references
- E. Kroke and M. Schwarz, Coord. Chem. Rev., 2004, 248, 493 CrossRef CAS.
- D. C. Cameron, Surf. Coat. Technol., 2003, 169–170, 245 CrossRef CAS.
- F. Z. Cui and D. J. Li, Surf. Coat. Technol., 2000, 131, 481 CrossRef CAS.
- A. Thomas, A. Fischer, F. Goettmann, M. Antonietti, J. O. Müller, R. Schlögl and J. M. Carlsson, J. Mater. Chem., 2008, 18, 4893 RSC.
- F. Goettmann, A. Fischer, M. Antonietti and A. Thomas, Angew. Chem., Int. Ed., 2006, 45, 4467 CrossRef CAS.
- A. Fischer, M. Antonietti and A. Thomas, Adv. Mater., 2007, 19, 264 CrossRef CAS.
- X. C. Wang, K. Maeda, A. Thomas, K. Takanabe, G. Xin, J. M. Carlsson, K. Domenand and M. Antonietti, Nat. Mater., 2008, 8, 76 CrossRef.
- Y. J. Zhang, A. Thomas, M. Antonietti and X. C. Wang, J. Am. Chem. Soc., 2009, 131, 50 CrossRef CAS.
- X. H. Li, J. S. Zhang, X. F. Chen, A. Fischer, A. Thomas, M. Antonietti and X. C. Wang, Chem. Mater., 2011, 23, 4344 CrossRef CAS.
- G. F. Jiang, C. H. Zhou, X. Xia, F. Q. Yang, D. S. Tong, W. H. Yu and S. M. Liu, Mater. Lett., 2010, 64, 2718 CrossRef CAS.
- X. Xu, R. Ray, Y. Gu, H. J. Ploehn, L. Gearheart, K. Raker and W. Scrivens, J. Am. Chem. Soc., 2004, 126, 12736 CrossRef CAS.
- J. Zhou, C. Booker, R. Li, X. Zhou, T. K. Sham, X. Sun and Z. Ding, J. Am. Chem. Soc., 2007, 129, 744 CrossRef CAS.
- H. Liu, T. Ye and C. Mao, Angew. Chem., Int. Ed., 2007, 46, 6473 CrossRef CAS.
- M. Groenewolt and M. Antonietti, Adv. Mater., 2005, 17, 1789 CrossRef CAS.
- M. M. Richter, Chem. Rev., 2004, 104, 3003 CrossRef CAS.
- W. Miao, Chem. Rev., 2008, 108, 2506 CrossRef CAS.
- Y. Dong, N. Zhou, X. Lin, J. Lin, Y. Chi and G. Chen, Chem. Mater., 2010, 22, 5895 CrossRef CAS.
- Z. Ding, B. M. Quinn, S. K. Haram, L. E. Pell, B. A. Korgel and A. J. Bard, Science, 2002, 296, 1293 CrossRef CAS.
- N. Myung, Y. Bae and A. J. Bard, Nano Lett., 2003, 3, 1053 CrossRef CAS.
- N. Myung, X. Lu, K. P. Johnston and A. J. Bard, Nano Lett., 2004, 4, 183 CrossRef CAS.
- L. Y. Zheng, Y. W. Chi, Y. Q. Dong, J. P. Lin and B. B. Wang, J. Am. Chem. Soc., 2009, 131, 4564 CrossRef CAS.
- Y. Dong, R. Wang, H. Li, J. Shao, Y. Chi, X. Lin and G. Chen, Carbon, 2012, 50, 2810 CrossRef CAS.
Footnote |
† Electronic supplementary information (ESI) available. See DOI: 10.1039/c2nr32248j |
|
This journal is © The Royal Society of Chemistry 2013 |