DOI:
10.1039/C2IB20166F
(Paper)
Integr. Biol., 2013,
5, 133-143
Surface modification of the TiO2 nanoparticle surface enables fluorescence monitoring of aggregation and enhanced photoreactivity†
Received
2nd May 2012
, Accepted 21st August 2012
First published on 29th August 2012
Insight, innovation, integration
This work identifies a method to monitor TiO2 nanoparticle aggregation both in vitro and intracellular without the use of transmission electron, X-ray fluorescence, or atomic force microscopies, but rather with fluorescence. This will enable real time tracking and differentiation of TiO2 nanoparticles and larger aggregates in cancer cells. To achieve these results, we incorporated the fluorescent dye, alizarin blue black B (ABBB) into TiO2 nanoconjugates, which ligates to the undercoordinated Ti metal center found at the corner defects of TiO2 nanoparticles. This paper integrates technology and biology in two manners: First, each of the two resulting ABBB–TiO2 nanoconjugate species emits a unique fluorescence signal upon excitation by visible light, enabling visualization and differentiation of nanoparticles and nanoparticle aggregates. Second, visible light activated ABBB–TiO2 nanoconjugates are capable of generating localized production of reactive oxygen species to damage cancer cells in a tunable manner. Each of these advancements will enhance the use of TiO2 nanoparticles in cancer theranostics at superficial sites or other locations where visible light can be applied.
|
Introduction
Nanomedicine is a rapidly developing sub-discipline of nanobiotechnology that has emerged over the last decade and is already advancing new medical technologies into the clinic.1 Nanoparticles possess unique characteristics at the nano scale2 and thus can be modified with various ligands to create nanoparticles that allow targeting and manipulating of desired cellular structures and biological agents.3 Much research within nanomedicine is focusing on using biologically and chemically modified nanoparticles to enhance diagnosis and treatment of various cancers. Titanium dioxide (TiO2) nanoparticles have been studied in the larger field of nanotechnology for several decades,4 but are only recently receiving more attention for their promise in cancer research.3f Bulk TiO2 possesses high stability, anti-corrosiveness, photoreactivity, a general lack of toxicity in humans,4b and is used in a wide range of products such as paints, sunscreens, cosmetics, and pharmaceuticals. As particle size decreases, TiO2 nanoparticles possess an increasing proportion of undercoordinated sites on the particle surface called ‘corner defects’ that allow the coordination of numerous chemical and biological materials.3f,g Bare TiO2 nanoparticles can also be excited using ultra-violet (UV) light exposure to release reactive oxygen species (ROS) into their surrounding environment.5 These properties yield biologically and chemically modified TiO2 nanoparticles useful in targeting and destroying cancer cells. UV light activation of TiO2 nanoparticles is undesirable in cancer applications as UV is a well-known mutagen and would thus adversely affect both the TiO2-targeted cancer cells as well as surrounding healthy cells. Therefore, recent investigations have focused on methods to allow visible light activation.3a
It is important to understand the dynamics between nanoparticles and cancer cells in cancer nanotechnology. The interactions that take place between the TiO2 nanoparticles and living cells are still being elucidated. The mechanism of nanoparticle cellular entry varies depending on the cell type that is treated, charge of the nanoparticles, degree of nanoparticle aggregation, and morphology of the cell.3d Previous research suggests that once bare TiO2 nanoparticles enter a cell, they remain inside endocytic vesicles6 and do not enter the nucleus, but rather take on a peri-nuclear localization surrounding the outer envelope.3a,7 The uptake of nanoparticles has been found to be time, temperature, and concentration dependent,6 and is likely affected by nanoparticle aggregation. Nanoparticle aggregation is a common problem in nanoparticles preparation and this likely decreases targeting and photoreactivity in the TiO2 nanoparticle regime. Consequently, there is a need to distinguish size of intracellular TiO2 nanoparticle aggregates by means that allow real time monitoring and are less invasive than transmission electron, X-ray fluorescence, and atomic force microscopy.
Herein we demonstrate that alizarin blue black B (ABBB) coordinated to the TiO2 nanoparticle enhances the resulting nanoparticles by (1) enabling the monitoring of intracellular TiO2 nanoparticle aggregation and (2) enhancing visible light activation of TiO2 nanoparticles above previously described methods to induce in vitro and intracellular damage to DNA and other targets. The creation of ABBB–TiO2 nanoparticles is demonstrated through sedimentation, spectral absorbance, and gel electrophoresis assays. The mechanism of ABBB modification of TiO2 is investigated through computational methods. Fluorescence emission spectroscopy studies indicate that ABBB coordination to the TiO2 nanoparticle surface enables discernment between nanoparticles and nanoparticle aggregates both in vitro and intracellular through fluorescence confocal microscopy. Visible light activated ABBB–TiO2 nanoparticles are capable of increased DNA cleavage as shown by visualizing the resulting plasmid DNA damage both with gel electrophoresis and atomic force microscopy. Finally, intracellular ABBB–TiO2 nanoparticles are capable of inflicting damage upon HeLa cells by inducing alterations in DNA structure and membrane associated proteins when excited with visible light.
Materials and methods
TiO2 nanoparticles were synthesized through previously described methods8 and also purchased from Sigma (St. Louis, MO, USA). The nanoparticles were prepared using similar methods and the experimental results depicted were replicated with nanoparticles from each source. TiO2 nanoparticles were characterized by transmission electron microscopy (TEM, Tecnai F20), BET surface analysis, and a Nanosight LM10-HSBF Nanoparticle Characterization System. These nanoparticles were either used immediately or allowed to age and re-characterized before using. This resulted in two batches of nanoparticles, the first containing 21 nm TiO2 nanoparticles, and the second being an aged batch containing 160 nm TiO2 nanoparticle aggregates as the dominant species. Modification of TiO2 nanoparticles with the fluorescent dye, alizarin blue black B (ABBB, Sigma), was characterized through three different experimental methods (sedimentation, spectral light absorbance, and polyacrylamide gel electrophoresis) as well as modeling by computational methods. Sedimentation assays were performed by incubating ABBB and TiO2 nanoparticles, centrifuging samples at 0.2 g for 3 minutes, then photographing with a Cannon Powershot 7.1 Megapixel A620 Digital Camera. Shifts in spectral light absorbance between ABBB and ABBB-modified TiO2 nanoparticle samples were measured with a NanoDrop 2000 UV-Vis spectrophotometer (Thermo Scientific, Waltham, MA, USA) as described previously.7 Stability between ABBB–TiO2 nanoparticles was assessed by running samples through a 16% polyacrylamide gel for 2 hours as described previously.3c
Computational investigation of the interaction of ABBB with the TiO2 nanoparticle surface
The X-ray single crystal structure of a similar Ti complex was used as the starting point for the calculations.9 As the commercially obtained ABBB has not been synthesized in an isomerically pure form and has not been tested for its isomeric content, we accounted for all of the possible isomeric structures in our analysis. The gas phase structures were fully optimized in redundant internal coordinates,10 using density functional theory (DFT)11 and a wave function incorporating Becke's three-parameter hybrid functional,12 along with the Lee–Yang–Parr correlation functional.13 All of the calculations were performed using the Gaussian 09 (G09) package.14 The Ti and S atoms were represented with the effective core pseudopotentials of LanL2DZ and the associated basis sets.15 This basis set was verified to give comparable results obtained by using the large 6-31+G(d,p) basis set.16 The remaining atoms (C, H, N and O) were represented with 6-31+G(d,p) basis sets.17 The geometry optimizations were performed without any symmetry constraints, and the local minima were checked by frequency calculations. The polarity of the structures led to the solvent effects on their relative stabilities being evaluated by calculating the free energies of solvation using the integral equation formalism polarizable continuum model (IEF-PCM).18 The solvation cavity was generated using Universal Force Field (UFF) radii, and the radii were scaled by a factor of 1.1. The self consistent reaction field (SCRF) calculations were carried out for the gas-phase-optimized structures. The dielectric constant in the PCM calculations was set to ε = 78.3553 to simulate water as the solvent medium used in the experimental study. The energies discussed throughout the text are total free energies in solution. Gibbs free energies have been calculated at 298.15 K and 1 atm. The solvation free energy of a proton in water solvent was taken to be −260 kcal mol−1, which is widely accepted in the literature.19
Measuring in vitro and intracellular TiO2 nanoparticle aggregation with fluorescence
Fluorescence spectral emission of the 21 nm ABBB–TiO2 nanoparticles and 160 nm ABBB–TiO2 nanoparticles were measured in vitro using a NanoDrop 3300 Fluorospectrometer (Thermo Scientific). Intracellular fluorescence spectral emissions of the same two ABBB–TiO2 nanoparticle species were measured using an Olympus IX81-UCB Spinning Disc Confocal Microscope (Olympus, Center Valley, PA, USA) using a 100 W mercury burner (Ushio, Tokyo, Japan), brightline filters for TEXAS RED and Cy5 (Semrock, Rochester, NY, USA), and an ORCA-ER-1394 high resolution digital camera (Hamamatsu, Japan). Each image is presented as a 1 μm optical slice.
Visualizing plasmid cleavage resulting from visible light photoactivation of ABBB–TiO2 nanoparticles
The effect of visible light activated ABBB–TiO2 nanoparticles on plasmid DNA was assessed by incubating pEGFP-C1 plasmid (90.88 ng μl−1) with dye modified TiO2 nanoparticles (3.33 μM) at 20 °C for 30 minutes. These samples and respective controls were then either illuminated with a Fiber-Lite MI-150 High Intensity Fiber Optic EKE 150 W 21 V Halogen Light Illuminator (Dolan-Jenner Industries, Boxborough, MA, USA) for 10 minutes or kept in dark conditions. All samples were then run on a 1.25% agarose gel in TAE buffer at 60 volts for 4 hours, stained with GelStar (Lonza, Mapleton, IL, USA), and imaged on a Kodak Gel Logic 2200 Imaging System (Kodak, Rochester, NY, USA) to view mobility differences between nicked, linearized, and supercoiled plasmid DNA. Portions of each of the same samples were applied to a freshly cleaved mica surface (Ted Pella, Redding, CA, USA) that was treated with sterile filtered MgCl2 (5 mM). Each sample was imaged using PeakForce Tapping Mode with Scan Asyst Air probes on an Icon Dimension Atomic Force Microscope (Bruker, Camarillo, CA, USA) to further confirm plasmid configurations. Images are presented as 2.0 μm × 2.0 μm after flattening and without further modification.
HeLa cells were grown on glass coverslips to approximately 20% confluence and then exposed to 21 nm ABBB–TiO2 nanoparticles or appropriate controls for 3 hours. When applicable, respective samples were either exposed to dark or light with a 750 W halogen bulb at a distance of 46 cm for an indicated period of time. All samples were fixed in 3.6% formaldehyde (Fisher Scientific, Waltham, MA, USA) and permeabilized in 0.2% Triton-100× (Fisher Scientific) for 10 minutes when later antibody labeling was performed. Some cells were then stained with a mouse monoclonal to emerin primary antibody (Abcam, Cambridge, MA, USA) followed by an Alexa488 goat anti mouse IgG (H+L) secondary antibody (Invitrogen, Grand Island, NY, USA). Some samples were then stained with Hoechst 33342 (Sigma) and coverslips were mounted in p-phenylenediamine-containing mounting media. Samples were imaged on an Olympus IX81-UCB Spinning Disc Confocal Microscope using a 100 W mercury burner, Brightline filters for DAPI, FITC, TEXAS RED, and/or Cy5 (Semrock), and an ORCA-ER-1394 high resolution digital camera. Each image is presented as a 1 μm optical slice.
Results
Characterizing dye–TiO2 nanoparticle interactions
To demonstrate the successful modification of TiO2 nanoparticles with the fluorescent dye, alizarin blue black B (ABBB), we utilized established experimental techniques such as sedimentation, spectrophotometry, and polyacrylamide gel electrophoresis.7 The possible coordination modes of ABBB were evaluated by computational methods as described in the next section. In the sedimentation assay, test tubes containing either (1) dH2O, (2) ABBB, (3) TiO2 nanoparticles, or (4) ABBB–TiO2 nanoparticles were prepared. Upon centrifugation of these samples at 0.2 g, TiO2 nanoparticles sedimented out of solution and formed a white pellet on the test tube bottom (Fig. 1a, tube 3), while free ABBB dye did not sediment under the same conditions (Fig. 1a, tube 2). In the tube containing ABBB dye and TiO2 nanoparticles, a deep purple pellet was observed at the bottom of the test tube, indicating successful interaction between ABBB and TiO2 nanoparticles (Fig. 1a, tube 4). When measuring spectral absorbance, ABBB-modified TiO2 nanoparticles demonstrated a red shift in spectral absorbance compared to their free dye counterparts (Fig. 1b), indicative of a dye–nanoparticle interaction.7 In the gel electrophoresis assay, we took advantage of the fact the TiO2 nanoparticles do not enter into a polyacrylamide gel during typical electrophoresis,3f while ABBB does enter into such a gel,3c to assess the stability of ABBB–TiO2 nanoparticle interactions. The same samples described above were used. Upon polyacrylamide gel electrophoresis of samples, ABBB (Fig. 1c, lane 2) migrated through the gel, while TiO2 nanoparticles (Fig. 1c, lane 3) and ABBB-modified TiO2 nanoparticles remained trapped in the wells of the gel and a distinct purple band was visible (Fig. 1c, lane 4). This electrophoresis data further supported the ability of ABBB dye to successfully interact with TiO2 nanoparticles and endure electrophoresis conditions. The chemical structure of ABBB is provided (Fig. 1d).
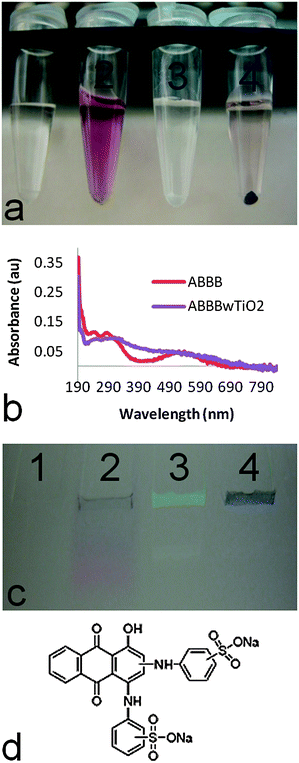 |
| Fig. 1 The interaction between ABBB and TiO2 nanoparticles is demonstrated through three different assays. (a) A sedimentation assay illustrated that upon centrifugation of samples at 0.2 g, ABBB (tube 2) remained in the supernatant, while TiO2 nanoparticles (tube 3) and ABBB–TiO2 nanoparticle modified (tube 4) formed a pellet at the bottom of the tube (tube 1 = dH2O). (b) ABBB-modified TiO2 nanoparticles (purple line) demonstrated a red shift in spectral absorbance compared to their ABBB counterparts (red line). (c) Upon polyacrylamide gel electrophoresis of samples, ABBB (lane 2) migrated through the gel, while TiO2 nanoparticles (lane 3) and ABBB-modified TiO2 nanoparticles (lane 4) remained trapped in the wells of the gel (tube 1 = dH2O). (d) The chemical structure of ABBB is shown (Sigma). | |
Computational investigation of the interaction of ABBB with the TiO2 nanoparticle surface
The commercially obtained ABBB has not been synthesized in isomerically pure form and has not been tested for its isomeric content. Therefore, it is possible to have several different isomeric structures. In our investigation of the interaction of ABBB with the TiO2 nanoparticle surface, we considered both the ortho- and meta-positions of the amine substituents of ABBB. There are multiple possible coordination sites, both monodentate and bidentate, through which ABBB can coordinate to the five coordinate corner defect sites of the TiO2 nanoparticle surface. It was suggested by Rajh et al. that similar compounds to ABBB prefer bidentate coordination modes to the TiO2 nanoparticle surface over monodentate coordination modes.3g Therefore we focused on the bidentate coordination modes of ABBB to the TiO2 nanoparticle surface (Scheme 1). In structures A, B, C and D, the bidentate coordination of ABBB to the TiO2 nanoparticle surface results in the formation of six membered rings with O–Ti–O and O–Ti–N angles of 120°. On the other hand, in structures E and F, the coordination of ABBB results in the formation of five membered rings with O–Ti–N and N–Ti–N angles of 108°. As the Ti metal center adopts an octahedral geometry in the bulk metal oxide with an O–Ti–O angle of 90°, five membered ring structures were proposed to result in less distortion from the ideal octahedral geometry and in a more favorable coordination mode than the coordination mode resulting in six membered ring structures. In structures G, H, I and J, the bidentate coordination of the sulfonate group of ABBB would result in the formation of unfavorable four membered rings with considerable ring strain.
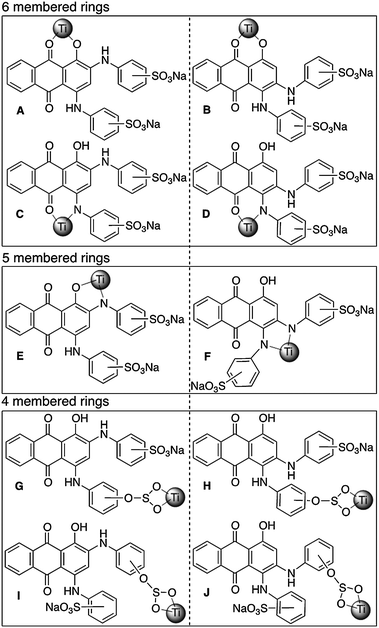 |
| Scheme 1 Possible bidentate coordination modes of structural isomers of ABBB to TiO2 nanoparticle surface. Structures A, C, E, G and I are the Ti–ABBB complexes resulting from the coordination of the meta-isomer of ABBB to the TiO2 nanoparticle surface and structures B, D, F, H and J result from the ortho-isomer of ABBB. Ti nanoparticle surface is represented by a sphere. | |
The interactions of structural isomers of ABBB with the TiO2 nanoparticle surface were investigated by using the Ti(OH)4 fragment to model the TiO2 nanoparticle surface (Scheme 2). The Ti–ABBB complexes resulting from the coordination of the meta-isomer of ABBB to the Ti(OH)4 fragment (Acalcd, Ccalcd, Ecalcd and Gcalcd) and those resulting from the ortho-isomer of ABBB (Bcalcd, Dcalcd and Fcalcd) were compared within each other (Fig. 2). The gas phase optimized structures all show an octahedral geometry around the Ti metal center, except for Gcalcd, in which ABBB coordinates through its sulfonate group. The ring strain associated with the four membered ring resulted in the cleavage of one of the S–O–Ti bonds, leading to a trigonal bipyramidal geometry around the Ti metal center. The relative solution free energies of Acalcd, Ccalcd, Ecalcd and Gcalcd suggest the significantly more favorable interaction of the ABBB through its sulfonate group over the other possible coordination sites. This might be due to the conservation of the distorted geometry of the Ti metal center on the defect sites and additional stabilization of this coordination mode with a six membered hydrogen bonding interaction with the surface Ti–O group. The relatively more favorable interaction of O coordination sites over N coordination sites agrees well with the hardness of the ligands: the harder O ligand binds stronger to the hard TiIV metal center.
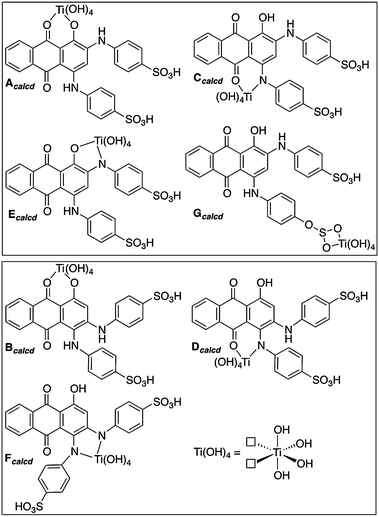 |
| Scheme 2 Ti–ABBB complexes used to model the possible bidentate coordination modes of structural isomers of ABBB to the Ti(OH)4 fragment. Structures Acalcd, Ccalcd, Ecalcd and Gcalcd are the Ti–ABBB complexes resulting from the coordination of the meta-isomer of ABBB to the TiO2 nanoparticle surface and structures Bcalcd, Dcalcd and Fcalcd result from the ortho-isomer of ABBB. The surface defect sites on the TiO2 nanoparticles are modeled using the Ti(OH)4 fragment, shown in the bottom right-hand corner. | |
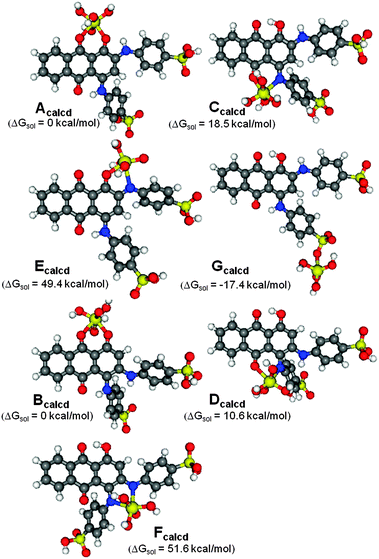 |
| Fig. 2 Gas-phase optimized ground state structures of Ti(OH)4–ABBB complexes used to model the possible coordination modes of structural isomers of ABBB to the TiO2 nanoparticle surface. Structures Acalcd, Ccalcd, Ecalcd and Gcalcd are the Ti–ABBB complexes with the meta-isomer and structures Bcalcd, Dcalcd and Fcalcd are with the ortho-isomer of ABBB. Only the para-position of sulfonate group has been investigated. The relative free energies in solution (kcal mol−1) with respect to Acalcd for Ccalcd, Ecalcd and Gcalcd; Bcalcd for Dcalcd and Fcalcd. | |
Measuring in vitro and intracellular TiO2 nanoparticle aggregation with fluorescence
We used a Nanodrop 3300 to view the visible light induced spectral emission of the different nanoparticles used within this study (Fig. 3). When 21 nm ABBB–TiO2 nanoparticles were excited with visible light, they emitted a strong fluorescence signal from 500–600 nm (Fig. 3a), which corresponds to the TEXAS RED brightline filter channel on the confocal microscope used later in this study. Visible light excitation of the 160 nm ABBB–TiO2 nanoparticles yielded two fluorescence emission peaks, the first being 500–560 nm (which is not detectable on the standard light/filter configurations on the confocal microscope used later) and the second being 625–725 nm (Fig. 3b) (which is compatible with both the Texas RED and Cy5 brightline confocal filters). The different in vitro fluorescence emission spectra produced by 21 nm and 160 nm ABBB–TiO2 nanoparticles provide a mechanism to discern smaller 21 nm TiO2 nanoparticles from their larger 160 nm aggregate counterparts within a cell via fluorescence confocal light microscopy. ABBB coordination of 21 nm TiO2 nanoparticles is expected to yield fluorescence emission in the TEXAS RED channel, whereas ABBB coordination of 160 nm TiO2 nanoparticle aggregates should be detectable with fluorescence signals in both the TEXAS RED and Cy5 channels simultaneously, and these signals should overlay.
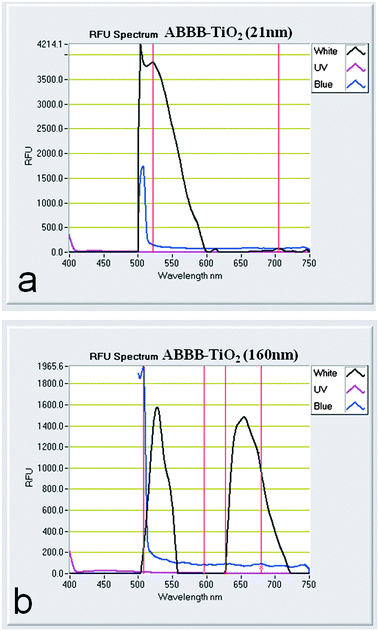 |
| Fig. 3 Fluorescence emissions of (a) 21 nm ABBB–TiO2 nanoparticles and (b) 160 nm ABBB–TiO2 nanoparticles are depicted. Samples were excited with visible (black line), blue (blue line), and UV (pink line) LED light sources. | |
The intracellular spectral emission data of the different nanoparticles used within this study (Fig. 4) correlated with the in vitro predictions. ABBB coordinating to the 21 nm TiO2 nanoparticles enabled visualization of the 21 nm TiO2 nanoparticles (red) in the TEXAS RED channel and the localization was speckled in a perinuclear fashion. Conversely, 160 nm ABBB–TiO2 nanoparticles were detectable (white boxes) in both the TEXAS RED (red) and Cy5 (purple) channels, and the fluorescence signals in these two channels overlapped as seen in the fluorescence overlay (magenta). In contrast to their 21 nm counterparts, the 160 nm ABBB–TiO2 nanoparticles exhibited fewer, more punctuate signals overall, suggesting that nanoparticle aggregation also influences localization.
 |
| Fig. 4 Fluorescence confocal microscopy images (1 μm slices) are presented as DIC, TEXAS RED, Cy5, fluorescence overlay and overlay. HeLa cells were exposed to each treatment for 3 hours, fixed in 4% formaldehyde, and then imaged. No fluorescence signals are detect when HeLa cells were exposed to water or ABBB, however when HeLa cells were exposed to 21 nm ABBB–TiO2 nanoparticles, they are detected within the RFP channel and exhibit a speckled, perinuclear localization. HeLa cells exposed to 160 nm ABBB–TiO2 nanoparticle aggregates are detectable both in the RFP and Cy5 channels. These results support the in vitro fluorescence emission findings obtained with the Nanodrop 3300. | |
Visualizing plasmid cleavage resulting from visible light photoactivation of ABBB–TiO2 nanoparticles
The DNA phosphate backbone has an affinity for TiO2.5b We have previously shown that visible light activated alizarin red s (ARS)–TiO2 nanoparticles are capable of releasing ROS and inducing DNA damage.3a Here we sought to expand upon these previous findings and compare the ability of ABBB–TiO2 nanoparticles (against that of their ARS counterparts) to induce plasmid DNA damage. We also sought to investigate the effect of nanoparticle aggregation on this dependent variable. A standard agarose gel electrophoresis technique that was developed by others was used to investigate these phenomena. Within this assay, nicked (single-stranded DNA break), linear (double-stranded DNA break), and supercoiled (undamaged) plasmid DNA can be distinguished on an agarose gel by viewing differences in mobility (with the nicked plasmid DNA migrating at the slowest rate and supercoiled plasmid DNA migrating at the fastest rate).3c Samples were prepared that contained plasmid DNA and either (1) dH2O, (2) ABBB, (3) TiO2 nanoparticles, or (4) ABBB–TiO2 nanoparticles (Fig. 5a). Half of each of the four samples was exposed dark and the other half of each sample was exposed to visible light from an EKE 150 W 21 V halogen bulb for 10 minutes. All samples were then loaded on a 1.25% agarose gel and electrophoresis was executed for 5 hours at 50 volts (Fig. 5b–e). This experiment was also repeated for ARS–TiO2 nanoparticles.
 |
| Fig. 5 (a) A schematic of the different samples used in this assay is presented. (b) Increased nicking and linearization of plasmid DNA was evident when 21 nm alizarin blue black B (ABBB)-modified TiO2 nanoparticles were exposed to visible light for 10 minutes (lane 4, yellow) compared to no light (lane 4, white), and this increase in nicking was greater than when ABBB was exposed to visible light (lane 2, yellow). (c) Reduced nicking of plasmid DNA was evident when 21 nm alizarin red s (ARS)-modified TiO2 nanoparticles were exposed to visible light for 10 minutes compared to their ABBB counterparts. The increase in plasmid nicking witnessed in the presence of visible light activated ARS-modified nanoparticles was greater than when ARS was exposed to visible light (lane 2, yellow). (d, e) When the experiment is repeated with 160 nm ABBB–TiO2 and ARS–TiO2 nanoparticles, the previously observed differences in plasmid cleavage between visible light activated dye (lane 2, yellow) and dye–TiO2 nanoparticles (lane 4, yellow) is no longer evident. All lanes contained plasmid DNA + either: lane 1 = no addition, lane 2 = dye, lane 3 = TiO2 nanoparticles, lane 4 = dye–TiO2 nanoparticles, yellow = visible light exposure, white = no light exposure. | |
All samples contained various configurations of plasmid DNA and the major conformation was supercoiled in form with the following exceptions. When plasmid DNA was exposed to visible light activated 21 nm ABBB–TiO2 nanoparticles (Fig. 5b, yellow lane 4), an increase in nicked (single-stranded break) and linearized (double-stranded break) plasmid DNA was observed, accompanied by a respective decrease in supercoiled (undamaged) plasmid DNA. Such an increase in nicked plasmid DNA was not seen in samples containing dark exposed ABBB–TiO2 nanoparticles or any other samples, with the exception of a slight, expected increase in nicked plasmid DNA in the visible light exposed samples containing ABBB (Fig. 5b, yellow lane 2). Visible light activated ABBB–TiO2 nanoparticles induced higher levels of DNA damage compared to their ARS counterparts (Fig. 5bversusFig. 5c). This was expected as the absorbance maximum of the former aligned more closely with the spectral emission of the utilized visible light source,3a thus highlighting the tunable nature of the dye–TiO2 nanoparticle platform.
One of the main tenets of nanotechnology is that the unique properties of nanomaterials are derived from the high ratio of surface atoms that dominate interactions in nanomaterials (compared to the same material in bulk).2 It is consistent that the enhanced plasmid cleavage seen upon exposure to visible light activated ABBB–TiO2 and ARS–TiO2 nanoparticles (relative to each dye alone) will diminish as nanoparticle size increases. When the above two experiments were repeated each with 160 nm ABBB–TiO2 and ARS–TiO2 nanoparticle aggregates, some plasmid cleavage was witnessed in all the light exposed dye and dye–TiO2 nanoparticle treatments, however, this effect was greatly diminished (compare Fig. 5dversusFig. 5b and compare Fig. 5eversusFig. 5c). These results emphasize the negative effect of nanoparticle aggregation on subsequent photoactivation and degradation of biological targets.
Atomic force microscopy was also used to further substantiate the alterations suggested in the above electrophoresis experiments. Selected subsamples of the above solutions were also placed on freshly cleaved mica surfaces for imaging in Peakforce Tapping mode. As seen through gel electrophoresis, the supercoiled plasmid conformation was dominant in most samples (Fig. 6). The exception was that when plasmid DNA was exposed to visible light activated ABBB–TiO2 nanoparticles, nicked (*) and linearized plasmid (⁁) conformations were also discovered (Fig. 6h). It is important to note that the positively charged mica surface facilitated strong interaction with the plasmid DNA, leading to tight plasmid supercoiling and particularly dense appearance observed in the supercoiled plasmid configurations.
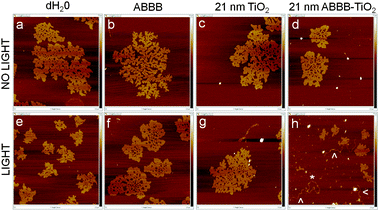 |
| Fig. 6 Representative sample images of plasmid DNA on freshly cleaved mica are presented using Peakforce Tapping Mode on a Dimension-Icon Atomic Force Microscope. Each image has the dimensions: 2.0 μm length × 2.0 μm width; 7.5 nm maximum height (white). All samples contain plasmid DNA plus (a, e) water, (b, f) ABBB, (c, g) 21 nm TiO2 nanoparticles, (d, h) 21 nm ABBB–TiO2 nanoparticles. Samples (a–d) were kept under dark conditions, whereas samples (e–h) were exposed to a 150 W halogen bulb for 10 minutes). | |
Effect of visible light activated ABBB–TiO2 nanoparticles on intracellular DNA structure and emerin localization
Bare TiO2 nanoparticles have been used in a variety of studies to induce damage in cancer cells upon excitation by UV light,5 and recently it has been shown that dye–TiO2 nanoparticles can induce cellular damage upon visible light activation.3a Visible light activation broadens the use of TiO2 nanoparticles in cancer research. Next, we sought to determine whether the 21 nm ABBB–TiO2 nanoparticles retained this capability to induce cellular damage upon exposure to visible light. To discern the variation of responses that occur under negative and positive control conditions HeLa cells were first exposed to either dark conditions or UV light for 3 minutes, fixed, stained for DNA and emerin, and viewed at either 8, 12 or 24 hours post exposure (Fig. 7). Ultraviolet (UV) light causes damage to cells by initiating thymine dimmers and is known to induce general apoptotic responses.20 HeLa cells exposed to dark conditions exhibited normal DNA staining (blue) and emerin (green) localization. Some HeLa cells exposed to UV light demonstrated nuclear condensation and abnormal emerin integrity and localization (*). These effects were evident at all time points, with the maximum impact being viewed at 24 hours post UV light exposure. It is important to note the heterogeneous cellular responses to UV light and the fact that many cells appear unaffected according to this assay.
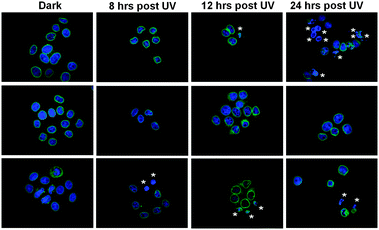 |
| Fig. 7 HeLa cells were exposed to UV light for 3 minutes and then fixed at different time points. This helps elucidate the post exposure time when any cellular alterations are most visible and serves as a positive control to which later experiments are compared. Maximum DNA condensation (*) is evident at 24 hours post UV exposure, though cellular response is heterogeneous. (Blue = Hoechst 33342/DNA; Green = Emerin). | |
HeLa cells were also exposed to water, ABBB, 21 nm TiO2 nanoparticles, or 21 nm ABBB–TiO2 nanoparticles (red) for 3 hours to investigate the same cellular responses. Samples were then exposed to either dark conditions or 750 W halogen visible light for 30, 60, or 180 minutes. After a 24 hour period of incubation, HeLa cells were fixed in 4% formaldehyde, stained to visualize DNA (blue) and emerin (green), and imaged using a fluorescence confocal microscope. Fluorescence overlays are presented as 1 μm optical slices (Fig. 8). No major alterations in DNA structure or emerin integrity were viewed in HeLa cells exposed to dark or 30 minutes of visible light for any of the treatments (Fig. 8). However, when HeLa cells were exposed to ABBB–TiO2 nanoparticles and visible light for 60 minutes, condensation of DNA and alterations in emerin integrity and localization were evident in some of the cells (*). These results were further exaggerated when ABBB–TiO2 nanoparticle-treated HeLa cells were exposed to visible light for 180 minutes. Under these conditions, DNA condensation, emerin disruption, and cellular aggregation were all viewed (*). As with the UV exposed, positive control cells, it is important to note that cellular responses were heterogeneous, and some cells demonstrated no detectable changes even when ABBB–TiO2 nanoparticle-treated cells were also exposed to visible light. It is also apparent that some photobleaching of samples occurred as the red fluorescence signal of the ABBB–TiO2 nanoparticles diminishes as light illumination time increases.
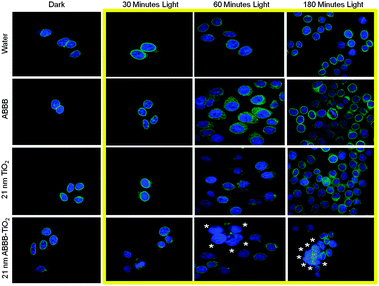 |
| Fig. 8 HeLa cells were exposed to water, ABBB, 21 nm TiO2 nanoparticles, or 21 nm ABBB–TiO2 nanoparticles and either no light or 750 W halogen visible light for 30, 60, or 180 minutes (yellow box). Cells were fixed in 4% formaldehyde 24 hours post exposure and 1 μm slices fluorescence confocal images are presented. Under this duration of light activation, no detectable cellular responses are visualized under any of the treatments exposed to dark or 30 minutes of visible light. Increased nuclear condensation, alterations in emerin integrity, and cellular aggregation (*) are visualized some cells exposed to ABBB–TiO2 nanoparticles and increased durations of visible light (60 and 180 minutes). The cellular response is heterogeneous. (Blue = Hoechst 33342/DNA; Green = Emerin; Red = ABBB–TiO2 nanoparticles). | |
Discussion
Cancer is the second most common cause of death in the US, exceeded only by heart disease, accounting for nearly 1 of every 4 deaths. According to the National Cancer Institute, about 1
638
910 new cancer cases are expected to be diagnosed in 2012 and about 577
190 Americans are expected to die of cancer within this same year.21 Cancer is currently treated with many approaches including surgery, chemotherapy, immunotherapy, and radiation; however, these treatments are often invasive or possess suboptimal targeting. Nanomedicine is investigating many new methods to enhance cancer diagnostics and therapeutics. TiO2 is one such promising nanoparticle platform due to its corner defects and photoreactivity, which readily allow modification by chemical and biological ligands and localized production of reactive oxygen species (ROS) respectively. The findings described in this current study expand upon the existing literature by developing the field's understanding of the mechanisms of dye–nanoparticle interactions, the use of nanoparticles in cancer imaging and therapeutics, and demonstrating the diverse set of cellular responses to nanoparticles.
The extent of the interaction between ABBB and TiO2 nanoparticles depends on the number of the corner defect sites on the TiO2 nanoparticles. It has been shown that as particle size decreases, TiO2 nanoparticles possess an increasing proportion of ‘corner defects’ which readily enable coordination of enediol bidentate and monodentate ligands to the nanoparticle surface.3g In light of our experimental results, the different interactions observed between ABBB and the 21 nm TiO2 nanoparticles and the larger 160 nm TiO2 nanoparticle aggregates correlated well with the expected number of defect sites. It is important that the details of these interactions are studied further to elucidate how the ABBB–TiO2 nanoparticle platform functions and can be manipulated. Additionally, a more complete understanding of the interactions between ABBB and different sized TiO2 nanoparticles and nanoparticle aggregates may help explain the observed photobleaching and define the longevity of photoactivation these nanoparticles possess.
Recently, it has been demonstrated that TiO2 nanoparticles can be modified with fluorescent dyes, and this allows fluorescence visualization of TiO2 nanoparticles both in vitro and within a cell.3c,7 Herein, we have demonstrated that a dye (ABBB) coordinating to TiO2 nanoparticles provides the means to discriminate 21 nm ABBB–TiO2 nanoparticles from their larger 160 nm aggregate counterparts via induced variations in spectral emissions. This provides a manner to monitor TiO2 nanoparticle aggregation both in vitro and intracellular via fluorescence rather than through other more invasive means such as transmission electron microscopy, X-ray fluorescence microscopy, and atomic force microscopy. Measuring TiO2 nanoparticle aggregation through alterations in fluorescence emission spectra will enable monitoring of this parameter in real time in live cells and benefit the study nanoparticle-cellular interactions in cancer nanotechnology research through enhanced imaging.
We have also shown that the ABBB modification of TiO2 nanoparticles enables enhanced visible light activation of TiO2 nanoparticles, and this localized production of reactive oxygen species (ROS) induces plasmid cleavage in vitro and alterations in DNA condensation and emerin distribution in HeLa cells. ROS possess relatively short half-lives, travel short distances, and can affect cells by destroying critical biological structures;22 so, their targeted generation can be particularly useful in cancer therapeutics. Identification of the types of ROS generated by various dye–TiO2 nanoparticles requires further investigation during the development of this platform. These properties of enhanced visualization and photoreactivity are desirable when targeting various superficial biological agents in cancer nanotechnology,6,7 but may be attenuated upon nanoparticle aggregation as suggested by our current study.
Future studies will need to further elucidate the cellular responses which were observed in the current investigation, as there are many possible explanations. Many biochemical events lead to characteristic changes in cell morphology, and if a cell cannot correct these changes, death often occurs. For example, apoptosis is one of the main types of programmed cell death, which involves a series of biochemical events leading to specific cell morphology changes, and ultimately cellular death.23 This is a multi-step, multi-pathway program that is found in every cell of the body. Upon receiving specific signals instructing a cell to undergo apoptosis many distinctive changes occur within a cell. Signs of apoptosis include: blebbing (changes to the cell membrane such as loss of membrane asymmetry and attachment) cell shrinkage, nuclear fragmentation, chromatin condensation, and chromosomal DNA fragmentation.23 In this current study, HeLa cells that were exposed to visible light activated ABBB–TiO2 nanoparticles, exhibited some of these aforementioned classic apoptotic signs such as nuclear condensation and fragmenting. However, other cells exhibited alternative reactions such as nuclear swelling, so the mechanisms behind the observed cellular responses are currently inconclusive and require further investigation focusing on specific protein response markers. For example, caspases are a family of proteases which are responsible for the morphological and biochemical changes behind apoptosis, may be an important factor to monitor in future studies. Caspases are typically activated in the early stages of apoptosis and continue to play an important role throughout the entire process.24 These proteases are present as inactive zymogens in essentially all animal cells, but can be triggered to assume active states that generally involve their proteolytic processing at conserved aspartic acid residues.25 It is possible that localized production of ROS by visible light activated ABBB–TiO2 nanoparticles could trigger such caspase activity, so this (along with other protein response markers) should also be investigated in future studies.
Conclusions
The in vitro and intracellular aggregation of TiO2 nanoparticles can be monitored via incorporation of the fluorescent dye, alizarin blue black B (ABBB) into the resulting nanoparticle. This same dye coordination enables visible light activation of the nanoparticles which can lead to DNA cleavage in vitro and DNA condensation and abnormalities in a nuclear membrane associated protein (emerin) within HeLa cells. ABBB–TiO2 nanoparticles possess the necessary characteristics to be a useful platform in cancer nanotheranostics at superficial sites or other locations where visible light can be applied.
Acknowledgements
The authors would like to thank the University of Wisconsin-Whitewater Undergraduate Research Program for supporting this work through a High Cost Undergraduate Research Grant awarded to Kara Kamps. The authors are also appreciative to the Tri Beta Biological Honors Society and the Microscopy Society of America for supporting this work through Undergraduate Research Scholarships awarded to Rachael Leek and Lanette Luebke respectively. Additionally, the authors would like to thank the College of Letters and Sciences and the Office of Research and Sponsored Programs at the University of Wisconsin-Whitewater for supporting this work respectively through a Faculty Start-Up Grant, a Professional Development Partial Reassignment Grant, and a Library Research Fellowship awarded to Eric Brown. Finally, the authors would like to thank Wisys for funding this work through an Applied Research Grant-Witag Match Grant 106-SYS-06-8000-4 awarded to Eric Brown. This research was supported in part by National Science Foundation Grant CHE-0840494.
References
-
(a) Magforce Nanotechnologies AG, http://www.cytimmune.com/go.cfm?do=page.view&pid=26, April 24, 2012;
(b) Cytimmune Sciences Inc., http://www.cytimmune.com/go.cfm?do=page.view&pid=26, April 24, 2012;
(c) Nanospectra, http://www.nanospectra.com/index.html, April 23, 2012;
(d) T. Osaka, T. Matsunaga, T. Nakanishi, A. Arakaki, D. Niwa and H. Iida, Synthesis of magnetic nanoparticles and their application to bioassays, Anal. Bioanal. Chem., 2006, 384, 593–600 CrossRef CAS.
- National Nanotechnology Intiative. What is nanotechnology? 2006. [cited; Available from: http://www.nano.gov/html/facts/whatIsNano.html].
-
(a) J. Blatnik, L. Luebke, S. Simonet, M. Nelson, R. Price, R. Leek, L. Zeng, A. Wu and E. M. B. Brown, Dye Surface Coating Enables Visible Light Activation of TiO2 Nanoparticles Leading to Degradation of Neighboring Biological Structures, Microsc. Microanal., 2012, 18, 134–142 CrossRef CAS;
(b) A. G. Wu, T. Paunesku, E. M. B. Brown, A. Babbo, C. Cruz, M. Aslam, V. Dravid and G. E. Woloschak, Titanium dioxide nanoparticles assembled by DNA molecules hybridization and loading of DNA interacting proteins, NANO, 2008, 3, 27–36 CrossRef CAS;
(c) E. M. B. Brown, T. Paunesku, A. Wu, K. T. Thurn, B. Haley, J. Clark, T. Priester and G. E. Woloschak, Methods for assessing DNA hybridization of peptide nucleic acid-titanium dioxide nanoparticles, Anal. Biochem., 2008, 383, 226–235 CrossRef CAS;
(d) K. T. Thurn, E. M. B. Brown, A. Wu, S. Vogt, B. Lai, J. Maser, T. Paunesku and G. E. Woloschak, Nanoparticles for applications in cellular Imaging, Nanoscale Res. Lett., 2007, 2, 430–441 CrossRef CAS;
(e) T. Paunesku, S. Vogt, B. Lai, J. Maser, N. Stojicevic, K. T. Thurn, C. Osipo, H. Liu, D. Legnini, Z. Wang, C. Lee and G. E. Woloschak, Intracellular distribution of TiO2-DNA oligonucleotide nanoparticles directed to nucleolus and mitochondria indicates sequence specificity, Nano Lett., 2007, 7, 596–601 CrossRef CAS;
(f) T. Paunesku, T. Rajh, G. Wiederrecht, J. Maser, S. Vogt, N. Stojicevic, M. Protic, B. Lai, J. Oryhon, M. Thurnauer and G. Woloschak, Biology of TiO2-oligonucleotide nanocomposites, Nat. Mater., 2003, 2, 343–346 CrossRef CAS;
(g) T. Rajh, L. X. Chen, K. Lukas, T. Liu, M. C. Thurnauer and D. M. Tiede, Surface restructuring of nanoparticles: An efficient route for ligand–metal oxide crosstalk, J. Phys. Chem. B, 2002, 106, 10543–10552 CrossRef CAS.
-
(a) T. Rajh,
et.al. Reactions of hydrous titanium-oxide colloids with strong oxidizing agents, Langmuir, 1992, 8, 1265–1270 CrossRef CAS;
(b) G. B. Hoflund, H. L. Yin, A. L. Grogan, D. A. Asbury, H. Yoneyama, O. Ikeda and H. Tamura, Surface Study of the Oxidative and Reductive Properties of TiO2(001), Langmuir, 1988, 4, 346–350 CrossRef CAS.
-
(a) J. W. Seo, H. Chung, M. Y. Kim, J. Lee, I. H. Choi and J. Cheon, Development of water-soluble single-crystalline TiO2 nanoparticles for photocatalytic cancer-cell treatmentm, Small, 2007, 3, 850–853 CrossRef CAS;
(b) A. Michelmore, W. Q. Gong, P. Jenkins and J. Ralston, The interaction of linear polyphosphates with titanium dioxide surfaces, Phys. Chem. Chem. Phys., 2000, 2, 2985–2992 RSC.
- K. T. Thurn, H. Arora, T. Paunesku, A. Wu, E. M. Brown, C. Doty, J. Kremer and G. Woloschak, Endocytosis of titanium dioxide nanoparticles in prostate cancer PC-3M cells, Nanomedicine, 2011, 7, 123–130 CAS.
- K. T. Thurn, T. Paunesku, A. G. Wu, E. M. B. Brown, B. Lai, S. Vogt, J. Maser, M. Aslam, V. Dravid, R. Bergan and G. E. Woloschak, Labeling TiO2 Nanoparticles with Dyes for Optical Fluorescence Microscopy and Determination of TiO2-DNA Nanoparticle Stability, Small, 2009, 5, 1318–1325 CrossRef CAS.
- A. Wu, T. Paunesku, E. M. B. Brown, A. Babbo, C. Cruz, M. Aslam, V. Dravid and G. E. Woloschak, Titanium dioxide nanoparticles assembled by DNA molecules hybridization and loading of DNA interacting proteins, NANO, 2008, 3(1), 27–36 CrossRef CAS.
- A. L. Zelikoff, J. Kopilov, I. Goldberg, G. W. Coates and M. Kol, New facets of an old ligand: titanium and zirconium complexes of phenylenediamine bis(phenolate) in lactide polymerisation catalysis, Chem. Commun., 2009, 6804–6806 RSC.
- C. Peng, P. Y. Ayala, H. B. Schlegel and M. J. Frisch, Using redundant internal coordinates to optimize equilibrium geometries and transition states, J Comput. Chem., 1996, 17, 49–56 CrossRef CAS.
- W. Kohn and L. J. Sham, Self-Consistent Equations Including Exchange and Correlation Effects, Phys. Rev., 1965, 140, A1133–A1138 CrossRef.
- A. D. Becke, Density-functional thermochemistry. III. The role of exact exchange, J. Chem. Phys., 1993, 98, 5648–5652 CrossRef CAS.
- C. Lee, W. Yang and R. G. Parr, Development of the Colle-Salvetti correlation-energy formula into a functional of the electron density, Phys. Rev. B: Condens. Matter Mater. Phys., 1988, 37, 785–789 CrossRef CAS.
-
M. J. Frisch, G. W. Trucks, H. B. Schlegel, G. E. Scuseria, M. A. Robb, J. R. Cheeseman, G. Scalmani, V. Barone, B. Mennucci, G. A. Petersson, H. Nakatsuji, M. Caricato, X. Li, H. P. Hratchian, A. F. Izmaylov, J. Bloino, G. Zheng, J. L. Sonnenberg, M. Hada, M. Ehara, K. Toyota, R. Fukuda, J. Hasegawa, M. Ishida, T. Nakajima, Y. Honda, O. Kitao, H. Nakai, T. Vreven, J. A. J. Montgomery, J. E. Peralta, F. Ogliaro, M. Bearpark, J. J. Heyd, E. Brothers, K. N. Kudin, V. N. Staroverov, R. Kobayashi, J. Normand, K. Raghavachari, A. Rendell, J. C. Burant, S. S. Iyengar, J. Tomasi, M. Cossi, N. Rega, N. J. Millam, M. Klene, J. E. Knox, J. B. Cross, V. Bakken, C. Adamo, J. Jaramillo, R. Gomperts, R. E. Stratmann, O. Yazyev, A. J. Austin, R. Cammi, C. Pomelli, J. W. Ochterski, R. L. Martin, K. Morokuma, V. G. Zakrzewski, G. A. Voth, P. Salvador, J. J. Dannenberg, S. Dapprich, A. D. Daniels, O. Farkas, J. B. Foresman, J. V. Ortiz, J. Cioslowski and D. J. Fox, Gaussian 09, Gaussian Inc, Wallingford, CT, 2009 Search PubMed.
-
(a) P. J. Hay and W. R. Wadt,
Ab initio effective core potentials for molecular calculations. Potentials for transition metal atoms Sc to Hg, J. Chem. Phys., 1985, 82, 270–283 CrossRef CAS;
(b) P. J. Hay and W. R. Wadt,
Ab initio effective core potentials for molecular calculations. Potentials for potassium to gold including the outermost core orbitals, J. Chem. Phys., 1985, 82, 299–310 CrossRef CAS.
- S. Manzhos, R. Jono, K. Yamashita, J.-i. Fujisawa, M. Nagata and H. Segawa, Study of Interfacial Charge Transfer Bands and Electron Recombination in the Surface Complexes of TCNE, TCNQ, and TCNAQ with TiO2, J. Phys. Chem. C, 2011, 115, 21487–21493 CAS.
- W. J. Hehre, R. Ditchfield and J. A. Pople, Theoretical investigations on the solvation process, J. Chem. Phys., 1972, 56, 2257–2261 CrossRef CAS.
- C. Gonzalez and H. B. Schlegel, An improved algorithm for reaction path following, J. Chem. Phys., 1989, 90, 2154–2161 CrossRef CAS.
- H. Reiss and A. Heller, The Absolute Potential of the Standard Hydrogen Electrode: A New Estimate, J. Phys. Chem., 1985, 89, 4207–4213 CrossRef CAS.
- D. M. Peters, J. B. Griffin, J. S. Stanley, M. M. Beck and J. Zempleni, Exposure to UV light causes increased biotinylation of histones in Jurkat cells, Am. J. Physiol., 2002, 283, C878–C884 CAS.
- American Cancer Society, Retrieved from www.cancer.org (30 November 2011).
- T. C. Long, N. Saleh, R. D. Tilton, G. V. Lowry and B. Veronesi, Titanium dioxide (P25) produces reactive oxygen species in immortalized brain microglia (BV2): implications for nanoparticle neurotoxicity, Environ. Sci. Technol., 2006, 40, 4346–4352 CrossRef CAS.
- E. J. Park, J. Yi, K. H. Chung, D. Y. Ryu, J. Choi and K. Park, Oxidative stress and apoptosis induced by titanium dioxide nanoparticles in cultured BEAS-2B cells, Toxicol. Lett., 2008, 180, 222–229 CrossRef CAS.
- L. J. Martin, Neuronal death in amyotrophic lateral sclerosis is apoptosis: possible contribution of a programmed cell death mechanism, J. Neuropathol. Exp. Neurol., 1999, 58, 459–471 CrossRef CAS.
- M. Weller and J. Reed, Apoptosis 2000 – Preface, Cell Tissue Res., 2000, 301, 3–3 CrossRef.
Footnote |
† Electronic supplementary information (ESI) available. See DOI: 10.1039/c2ib20166f |
|
This journal is © The Royal Society of Chemistry 2013 |
Click here to see how this site uses Cookies. View our privacy policy here.