DOI:
10.1039/C2IB20100C
(Paper)
Integr. Biol., 2013,
5, 144-150
Plasmonic gold and luminescent silicon nanoplatforms for multimode imaging of cancer cells†
Received
1st May 2012
, Accepted 24th August 2012
First published on 29th August 2012
Abstract
The development of multimodal nanoparticle platforms is desirable for cancer nanotechnology applications. Creating single nanoplatforms with both plasmonic and photoluminescent optical properties has remained a challenge, because combining discrete entities each having one of these unique properties typically results in the attenuation of one of the desirable properties. Here, we overcome challenges associated with combining plasmonic gold with luminescent silicon nanocrystals for biological imaging applications by incorporating multiple silicon quantum dots into the core of a micelle and then depositing gold on the surface of the nanostructure. Within the newly developed nanoconstruct, the gold shell exhibits plasmonic light scattering properties useful for dark field imaging, while the silicon nanocrystals maintain their photoluminescence. The result is a nanoplatform with both plasmonic and luminescent properties in a useful form. Multimodal imaging of pancreatic cancer cells demonstrates overlap of luminescence from the silicon quantum dots with light scattering from the gold shell. This approach can be tailored to other formulations and address the challenge of fluorescence attenuation that is typically observed when quantum dots are combined with plasmonic materials. The usefulness of these particles may eventually extend beyond multimodal imaging to include photothermal treatment.
Insight, innovation, integration
The phenomenon examined here is the imaging performance of multimodal nanostructures in a pancreatic cancer cell environment. We find that cancer cells can be imaged in both dark field and fluorescence optical microscopy modes using a single probe that possesses strong light scattering and photoluminescent emission capabilities. The key technical innovation is in the design of the nanoplatform, which combines silicon nanocrystals with gold, without bringing the two materials into direct contact, to provide multifunctionality in a single optical nanoprobe. The benefit is that such a probe could be used for early detection of cancer and with further development, may be used for photothermal treatment. The biocompatibility of the components of these nanoprobes enhances their potential for ultimate clinical application.
|
Introduction
Quantum dots (QDs) have been extensively studied for biological applications such as bio-imaging,1 bio-labeling,1b,d,2 and diagnostics1a because of their unique optical properties, including tunable emission, photostability, and brightness. Combining QDs with other materials, such as gold and iron oxide, which have complementary properties, creates multi-functional nanoplatforms with unique combinations of optical, electronic, plasmonic and magnetic properties.3 Investigations aimed at combining fluorescent QDs with the plasmonic gold as a multi-component nanostructure have illustrated persistent challenges. Mokari and Banin4 successfully grew gold on the tips of quantum rods and quantum dots. Also, Yang et al.5 and Shi et al.5c synthesized different hybrid QD-Au nanostructures. However, the direct combination of gold with QDs seriously attenuates the luminescence of the QDs, thereby resulting in particles that would be considered ineffective for biological imaging applications.6 To overcome this obstacle of fluorescence quenching, Jin and Gao7 combined CdSe and Au into a single nanostructure by tuning the distance between the QDs core and the gold shell through layer-by layer self-assembly, using an approach similar to Hong's method8 for synthesizing magnetic luminescent nanocomposites. Unfortunately, the cellular toxicity of cadmium-containing QDs9 has raised concerns that have not been clearly resolved. These toxicity concerns are a partial reason for the development of silicon quantum dots as a more biocompatible alternative.10
Combining silicon quantum dots with other bio-relevant materials is still in its infancy, yet the field shows promise. Silica, which is frequently confused with silicon, has been used extensively in nano-biotechnology. Silica is normally used as a nano-carrier, while silicon is generally investigated because of its size-dependent optical properties and its superior biocompatibility in comparison to heavy metals. Some general differences between silica (SiO2) and silicon (Si) are listed in Table S1 (ESI†). Silicon quantum dots have been combined with iron-oxide nanoparticles,11 manganese,12 and gadolinium13 to incorporate magnetic and optical properties into a single nanoconstruct. Even though the method used to combine iron-oxide with silicon quantum dots successfully showed that their individual properties can be incorporated into a single nanoplatform, the luminescence of the silicon quantum dots was attenuated by the presence of the iron oxide particles in close proximity.14 Manganese doping of silicon quantum dots resulted in a lower quantum yield of the multimodal particles emission but they were still useful for biological applications.15 We recently conjugated silicon quantum dots with gadolinium ions to create nanoparticles that have a relaxivity of 6000 mM−1 s−1 per nanoconstruct for potential use in MRI imaging applications.13 The external chelation of gadolinium resulted in an increase in photoluminescence intensity. Silicon quantum dots have also been successfully combined with 64Cu2+ for use as a multimodal PET imaging agent.15 A few studies have attempted to investigate interactions between silver and silicon; however, they are not related to biological applications.16 We tried incorporating gold into the core of a micelle, along with silicon quantum dots, by modifying the method we used for creating magnetofluorescent nanoconstructs.14 However, the gold quenched the luminescence of silicon quantum dots, making it impossible to take advantage of their desirable optical properties for biological applications. To our knowledge, gold has not been previously combined with silicon quantum dots for biological applications.
In this paper, we employed a facile method to prepare Si QD/Au core–shell nanostructures with controlled separation of the Au and Si components that preserves the optical properties of the components. We prepared luminescent Si QDs by following well-established procedures that have resulted in their biological application.11b,14,17 Silicon powder is synthesized by laser pyrolysis, followed by an etching procedure that establishes bright luminescence. The surface of the Si nanoparticles is functionalized with FDA-approved ethyl undecylenate and then multiple silicon quantum dots are encapsulated in micelles self-assembled from FDA-approved pluronic F127 for evaluation. For preparation of plasmonic luminescent silicon quantum dots, a COOH modified version of F127 is used to encapsulate the silicon nanoparticles. This water dispersible form of encapsulated silicon quantum dots is referred to as SiQD-COOH from this point. Two major advantages of using SiQD-COOH instead of single Si nanoparticle for synthesizing Si/Au core–shell nanostructure are: (i) the fluorescence of SiQD-COOH stems from multiple silicon nanocrystals in the core of the micelle resulting in much brighter fluorescence per nanoconstruct than can be obtained from individual silicon nanocrystals dispersed in water; (ii) some silicon nanocrystals in the pluronic micelle will always be significantly distant from the surface of the micelle to ensure that their luminescence will not be attenuated when an additional component is added as a shell. These distant silicon quantum dots will maintain their original luminescence or even exhibit increased luminescence due to plasmonic enhancement of local electric fields by the gold shell.
The challenges of synthesizing plasmonic QD nanocrystals were illustrated by Jin.7 However, these limitations are largely overcome using SiQD-COOH. Key advantages are: (i) accurate control of the gold shell thickness is no longer required because the emission from the SiQD-COOH nanoconstruct is much brighter than emission from individual SiQDs in water; (ii) the concern of small particles preventing the growth of a gold shell also disappears because the diameter of the SiQD-COOH nanoconstruct supplies a much better scaffold for the formation of a gold shell. (iii) complicated and repetitive layer-by-layer growth procedures that require polyelectrolytes such as polyallylamine hydrochloride (PAH) and polystyrene sulfonate (PSS) used for increasing the spacing between QDs core and gold shell are not needed.
Fig. 1 shows the strategy for preparing Si–Au hybrid core–shell nanostructure. After the self-assembly procedure, the surface of SiQD-COOH is modified by electrostatic interactions with poly-L-histidine (PLH) which can efficiently immobilize the gold ions at high density.18 We employ a mixture of auric chloride (HAuCl4) and sodium hydroxide (NaOH) as the gold shell precursor, keeping the pH value at 8.5–10. A facile reductive reaction is initiated at room temperature using hydroxylamine as a reducer under vigorous stirring. This results in a gold coated micelle with luminescent silicon in the core.
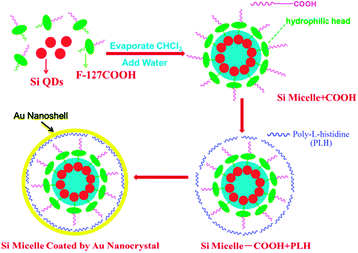 |
| Fig. 1 Schematic illustration of the synthesis of Si/Au hybrid nanostructure (golden-SiQD). Functionalized Si QDs (red) dispersed with F127-COOH (green representing F127 and purple representing the COOH group) in chloroform were evaporated and resuspended as micelles in HPLC water. Subsequently, PLH (blue curves) was grafted to the surface of the SiQD-COOH micelle through electrostatic interaction for effective immobilization of Au3+ at a high packing density. NH2OH was used to reduce Au3+ to produce a gold shell (yellow circle). The representative image is not drawn to scale. | |
Experiment methods
Materials
Silane (SiH4, Voltaix, electronic grade, 99.999%), hydrofluoric acid (HF, Acros Organic, 48–51%), nitric acid (HNO3, EMD, 68–70%), ethyl undecylenate (Sigma-Aldrich 95%), Pluronic F127 (BASF), and Auric Chloride (Sigma-Aldrich) were used as received if not otherwise noted. All solvents (chloroform, HPLC water, methanol) were of reagent grade and were used without further purification.
Silicon nanocrystals were prepared by high temperature CO2 laser pyrolysis of silane in an aerosol reactor based on the method established by Li et al.17 A procedure developed by Hua et al.20 was modified11b and used to create luminescent silicon nanocrystals. First, 300 mg of silicon nanoparticles was dispersed in 40 mL methanol. A mixture of 100 mL HF (48 wt%) and 10 mL HNO3 (69 wt%) was used to etch the sample for about 60–75 seconds, until the particles of desired luminescence color were created. Once Si NPs approached the desired PL wavelength, 400 mL of methanol was added to terminate the reaction. The particles were then collected on a PTFE filter and washed with 1.25 L of a 1
:
3 methanol–water mixture to remove excess acid. After rinsing with pure methanol four times, the particles were added to ethyl undecylenate for photoinitiated hydrosilylation. All of the above procedures were carried out under nitrogen. Silicon nanoparticles in ethyl undecylenate, in a vial containing a magnetic stirrer, were put in a Rayonet photochemical reactor (Southern New England Ultraviolet Co.) equipped with 16 RPR—2537 Å UV tubes to initiate hydrosilylation. The reaction time was about 24 hours for red particles in ethyl undecylenate. The completeness of reaction was indicated by the optical clarity of the solution. The clear samples were filtered by a PTFE syringe filter with a pore size of 0.45 μm and then centrifuged out after the addition of methanol and water as anti-solvents.
F127 COOH encapsulation
F127-COOH was prepared following the method described by Ding et al.21 Dry particles were dispersed in chloroform at a concentration of 1 mg Si QDs per 1 mL chloroform. In micelle encapsulation, 200 μL of silicon quantum dots and 200 μL of a 20 mg mL−1 solution of F127COOH were added to a 10 mL round bottom flask. After mild sonication, the solvent was evaporated using a rotary evaporator with a 37 °C water bath. 2 mL of HPLC water was used to hydrate the film formed on the reaction vials. The particles were sonicated briefly and then they were centrifuged (13
000 rpm, 15 min) and redispersed 3 times, then stored in the refrigerator at 4 °C for further use.
Preparation of gold-coated F127COOH-silicon (golden-SiQD) samples
The SiQD-COOH samples were dispersed in HPLC water after centrifugation at a concentration of 1 mg mL−1. A modification of the protocol developed by Jin and Gao was adopted.7 Poly-L-histidine (PLH) was allowed to electrostatically bind to the surface of the micelle through slow stirring for 1 hour. Excess PLH molecules were removed by centrifugation and redispersion (3 times). The QD-PLH solution was resuspended in HPLC water and stored for further use. For gold shell growth, a mixture of auric chloride (HAuCl4) and sodium hydroxide (NaOH) in HPLC water adjusted to a pH value of 8.5–9 served as the gold shell precursor. The solution was added to 1 mL of the SiQD-PLH solution and reduced by using hydroxyl amine. Upon hydroxylamine addition, the yellowish solution turned blue in a few minutes.
Characterization of the golden-SiQDs
The QD emission spectra were collected using a RF-5301PC spectrofluorophotometer (SHIMADZU). UV-Vis absorption spectra were acquired using a Shimadzu UV-3600 spectrophotometer. High-resolution transmission electron microscopy (HRTEM) images were obtained using a JEOL model JEM 2010 microscope at an acceleration voltage of 200 kV. The specimens were prepared by drop-coating the sample dispersion onto an amorphous carbon-coated 300 mesh copper grid, which was placed on filter paper to absorb excess solvent. Scanning electron microscopy (SEM) images were obtained using a Hitachi S4000 field emission microscope at an acceleration voltage of 25 kV.
Cell culture
Panc-1, ASPC-1, and MIA PaCa cancer cell lines were obtained from ATCC (American Type Culture Collection). Panc-1 was cultured in Dulbecco's minimum essential media (DMEM) with 10% fetal bovine serum (FBS), 1% penicillin and 1% amphotericin B. MIA PaCa-2 was cultured in DMEM media with pen/strep antibiotics, 10% FBS and 2.5% Horse Serum. ASPC-1 was cultured in RPMI-1640 media with pen/strep antibiotics and 10% FBS. The day before treatment with golden-SiQDs, cells were seeded in 35 mm culture dishes at a confluency of 70–80%. The cells in serum-supplemented media were treated with the golden-SiQDs for twenty four hours at 37 °C before imaging. For targeted cellular labelling studies the cells were treated for two hours before imaging.
Cell viability studies
For each MTS assay, 96 culture wells of Panc-1, ASPC-1, MIA PaCa-2 cell were prepared. Cells were seeded at a density of 5 × 103 cells per well. After 24 hours, the culture medium was replaced with 100 μL of medium containing increasing concentrations of golden-SiQDs from 20 to 250 μg mL−1. The various concentrations were subsequently incubated with the cells for 24 and 48 h at 37 °C under 5% CO2. As described in the literature,22 the absorbance of formazan (produced by the cleavage of MTS by dehydrogenases in living cells) is directly proportional to the number of live cells. After the incubation, 20 μL of the MTS reagent was then added to each well and well mixed. The absorbance of the mixtures at 490 nm was measured. The cell viability was calculated as the ratio of the absorbance of the sample well to that of the control well and expressed as a percentage.
Microscopy
The aperture dark field images were recorded using an upright Nikon Eclipse 800 microscope with a high numerical dark field condenser (N.A. 1.20–1.43, oil immersion) and a 100X/1.4 NA oil Iris objective (Cfi Plan Fluor). In the dark field configuration, the condenser delivers a narrow beam of white light from a tungsten lamp and the high NA oil immersion objective collects only the scattered light from the samples. The iris of the objective can be adjusted to optimize the collection and to reduce the leakage of transmitted light. The dark field imaging was captured using a QImaging Micropublisher 3.3 RTV color camera. A 0.5× lens was placed in front of the camera to provide a larger field of view. The Qcapture software from the camera manufacturer was used for image acquisition and has a feature for adjusting the white color balance for accurately capturing the color differences in samples. The luminescent images were taken on the same microscope, using a Nuance imaging software system and camera to acquire fluorescence images. A 400 nm short pass filter was used for the excitation and a 600 nm long pass filter was used for the emission measurements.
Results and discussion
Golden-SiQD nanostructure characterization
Fig. 2 shows transmission electron microscopy (TEM) images of the ethyl undecylenate terminated silicon quantum dots, an F127 based micelle containing the silicon quantum dots, and a gold coated micelle containing the silicon quantum dots (golden-SiQDs). The SiQDs are about 4 to 5 nm in diameter, and can be observed as individual particles in Fig. 2a. A higher magnification image is available in the ESI† (Fig. 1S). The micelle is larger than the silicon nanocrystals and its size can be adjusted by changing the ratio of Si-QDs to F127. Fig. 2(b) shows a micelle that is about 150 nm. A higher concentration of nanoparticles in the core of the micelle results in brighter fluorescence than that of individual SiQDs in water. The Selected Area Electron Diffraction (SAED) of golden-SiQDs presented in Fig. S2 (ESI†) shows the 111, 220 and 311 planes of silicon and the 111, 200, and 220 planes of gold from the same area. This demonstrates that the golden-SiQDs have crystalline components. The nanoparticles fabricated here may be of a desirable size. It is recommended that nanoparticles be up to 100 nm to reach tumor tissues by the enhanced permeation and retention (EPR) mechanism because the size of the gap junction between endothelial cells of leaky tumor vasculature may vary from 100 nm to 600 nm.23 The golden-SiQDs are 150 nm and may be suitable for EPR as has previously been shown for some gold nanostructures.24 The TEM image of the gold coated nanostructure demonstrates that a significant amount of gold can be deposited on the surface of the micelle. In TEM, gold has much stronger contrast than silicon and amorphous material and thus it is easily identifiable as the darker layer surrounding the micelle in Fig. 2c. Energy dispersive X-ray spectroscopy (EDS) (shown in Fig. S2 and Table S2, ESI†) confirmed the presence of gold on the surface of the Si micelle with an atomic ratio of Si
:
Au around 9
:
1. Other elements (C, Cu, O) are expected to come from the carbon-coated copper TEM grid or from the F-127 and PLH polymers.
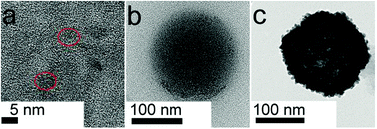 |
| Fig. 2 Transmission electron microscopy (TEM) images of ethyl-undecylenate terminated SiQDs, F127-COOH encapsulated SiQDs, and golden SiQD hybrid nanostructures. (a) TEM images of SiQDs dropcast from chloroform. Red circles indicate the location of some silicon quantum dots (a larger image is in the ESI† – Fig. S1); (b) F127-COOH encapsulated silicon quantum dots; (c) gold-coated F127-COOH encapsulated silicon quantum dots. | |
Fig. 3 shows normalized steady-state optical absorbance and photoluminescence spectra of the SiQD-COOH and the golden-SiQD nanostructures. The absorption of SiQD-COOH shows a peak around 400 nm and monotonically declines at longer wavelengths. The golden-SiQD spectrum exhibits a similar peak around 400 nm and an additional optical absorption peak at 627 nm. This peak is attributed to the presence of the Au nanoshell. The peak observed for the gold shell is consistent with our group's previous investigation of the growth of the Au nanoshell on polystyrene templates.25 Previous studies have reported quenching of photoluminescence in epitaxial semiconductor–metal heterogeneous NCs.6a Quenching was attributed to delocalization of excitons from the semiconductor domain into the metal domain or formation of hybridized electronic states at the semiconductor–metal interface. When we co-localized silicon and gold in the core of a micelle, we observed a similar quenching of photoluminescence. In the current case, by creating a gold shell, the fluorescence quenching effect is almost eliminated. This luminescence preservation is attributed to the SiQDs in the micelle being encapsulated in polymers that allow them to avoid direct contact with the Au nanoshells. There is a slight redshift in the emission spectra; however the integrated area under the SiQD-COOH curve and golden-SiQD curve is approximately the same.
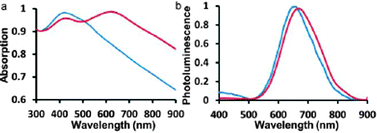 |
| Fig. 3 Optical properties of F127-COOH coated SiQDs before (blue curve) and after encapsulation (red curve) with a gold shell. (a) UV-Vis of the SiQD-COOH before and after encapsulation. The blue curve shows only one noticeable peak at 400 nm in the normalized absorbance profile of water-dispersible F127-COOH coated silicon quantum dots. The red curve shows two peaks at 400 and 630 nm in the normalized absorbance profile of gold coated F127-COOH coated SiQDs. (b) Photoluminescence emission spectra of the SiQD-COOH before and after encapsulation. The blue curve shows the normalized (to the maximum value of silicon emission) photoluminescence spectra of water-dispersible F127-COOH coated SiQDs. The red curve shows the normalized (to the maximum value of silicon emission) absorbance profile of gold coated F127-COOH coated SiQDs. The samples were excited at a wavelength of 365 nm. | |
Dark-field and cellular imaging
The dual-modality of the imaging probe was investigated by using dark field imaging and fluorescence microscopy. Fig. 4 shows images of small clusters of golden-SiQDs that were captured by 2 different camera systems on the same microscope system. After dilute samples were spread on glass coverslips, a nuance imaging system and a QImaging Micropublisher 3.3 RTV color camera were used to obtain fluorescence and light scattering images respectively. Upon close observation, the luminescence (Fig 4a) and the dark field micrographs (Fig 4b) show patterns of contrast overlap which confirm that the source of the scattering and luminescence are from the successful combination of the silicon and gold. The dark field image with 40% transparency was laid over the fluorescence image and is depicted in Fig. 4c.
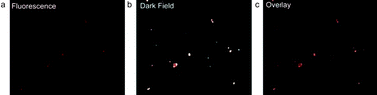 |
| Fig. 4 Fluorescence and dark-field imaging of single nanoparticles spread between two glass coverslips, (a) the fluorescence micrograph obtained with a 400 nm short pass filter for excitation and a 600 nm long pass filter for emission, (b) dark-field microscopy, (c) dark field micrograph overlay (at 40% transparency) on the fluorescence micrograph. | |
Golden-SiQDs were further evaluated for in vitro imaging. Pancreatic cancer cells were chosen as the cell line to demonstrate the multimodal potential of the golden-SiQDs in biological applications. Robust optical signals are seen in the cells treated with golden-SiQDs in Fig. 5a. Fig. 5b shows the dark-field images of the pancreatic cancer cells treated with 100 μg mL−1 of golden-SiQcsDs. There is significant overlap when luminescence micrographs are compared to the corresponding light scattering image in the dark-field micrograph as depicted in Fig. 5c. The phenomena examined here, the capabilities of golden-SiQDs to provide image contrast in a pancreatic cancer cell environment, reveal that this multimodal structure may be useful for obtaining photonic information from cancer cells. A few areas show scattering without clear evidence of corresponding luminescence overlap. Plasmon-enhanced scattering from gold is known to be much brighter than fluorescence from most fluorophores,22 thus it is detectable at probe concentrations where the fluorescence is not. Even though the study of gold nanostructures has been an active field of research for many years,26 their localization and penetration into cancer cells is still an active area of exploration.27 Further studies will seek to understand the dynamics of cell penetration of the golden-SiQDs. Confocal images of SiQDs targeted to different types of pancreatic cancer cells (Panc-1, MIA PaCa-2 and ASPC-1) are included in the ESI† (Fig. S3 and S4). Silicon is mildly effective when functionalized with hyaluronic acid to target CD44 receptors on the surface of the pancreatic cancer cells (Fig. S3, ESI†). Localization on the membrane can be accomplished more readily by using Anti-claudin-4, Anti-mesothelin and AntiGCTM-5 on panc-1 cancer cell lines (Fig. S4, ESI†). More advanced studies are needed to optimize targeting and to explore localization and penetration, even though the potential of SiQDs is clearly demonstrated in these images. Fig. 6 shows the cell viability of the golden-SiQDs in different cell lines at different concentrations. The golden SiQDs appear to be non-toxic over a 48 hour time period.
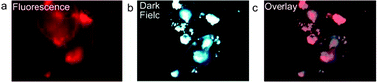 |
| Fig. 5 Fluorescence and dark-field imaging of pancreatic cancer cells treated with golden SiQDs, (a) the fluorescence micrograph obtained with a 400 nm short pass filter for excitation and a 600 nm long pass filter for emission, (b) dark-field microscopy micrograph, (c) dark field micrograph overlay (at 40% transparency) on the fluorescence micrograph. | |
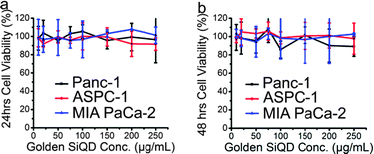 |
| Fig. 6 Cytotoxicity studies of golden SiQDs in different pancreatic cancer cell lines. Panc-1, ASPC-1, and MIA PaCa-2 cell-lines were treated with different concentrations of micelles and incubated for (a) 24 hours and (b) 48 hours. | |
Nanoprobes have limitations that accompany their unique properties. This combination of properties derived when silicon is combined with gold allows for multimodal imaging in pancreatic cancer cells. In addition to simultaneous imaging by carrying fluorescent and light scattering properties, it may allow for therapeutic properties such as photothermal treatment of cancer. Jin and Gao7 reported that gold shells may have improved biocompatibility compared to uncoated water dispersible CdSe QDs. This work shows a significant advancement because it creates gold shells without loss of luminescence in cadmium-free quantum dots and demonstrates that they can be used in biological applications. The size may be large for some applications, but for cancer they may be desirable for enhanced permeation and retention, which has been successfully demonstrated with larger particles.
Conclusions
In conclusion, we provide the first demonstration of a gold–silicon quantum dot complex that maintains the desirable optical properties of both components. This work overcomes the previously known challenges of luminescence quenching and interference from single nanoparticles with the creation of a gold shell. The golden-SiQDs are promising as a dual-modality probe in vivo because gold shells can be expected to degrade,7,8 silicon can be expected to degrade to silicic acid, while ethyl undecylenate and F127 pluronic are FDA approved. This is the only gold shell report for silicon and may be the best formulation for cancer nanotechnology because of the non-toxic components. This method can be applied to other non-toxic nanostructures and can form the basis for more advanced studies in cancer nanotechnology.
Acknowledgements
This study was supported by grants from the Ford Foundation Fellowship 10995602-1-57847, Theranostics Lab and the National Cancer Institute NCI RO1CA119397.
Notes and references
-
(a) X. Michalet, F. F. Pinaud, L. A. Bentolila, J. M. Tsay, S. Doose, J. J. Li, G. Sundaresan, A. M. Wu, S. S. Gambhir and S. Weiss, Quantum Dots for Live Cells, In Vivo Imaging, and Diagnostics, Science, 2005, 307, 538–544 CrossRef CAS;
(b) I. L. Medintz, H. T. Uyeda, E. R. Goldman and H. Mattoussi, Quantum dot bioconjugates for imaging, labelling and sensing, Nat. Mater., 2005, 4, 435–446 CrossRef CAS;
(c) B. Dubertret, P. Skourides, D. J. Norris, V. Noireaux, A. H. Brivanlou and A. Libchaber,
In Vivo Imaging of Quantum Dots Encapsulated in Phospholipid Micelles, Science, 2002, 298, 1759–1762 CrossRef CAS;
(d) X. Gao, Y. Cui, R. M. Levenson, L. W. K. Chung and S. Nie,
In vivo cancer targeting and imaging with semiconductor quantum dots, Nat. Biotechnol., 2004, 22, 969–976 CrossRef CAS.
-
(a) U. Resch-Genger, M. Grabolle, S. Cavaliere-Jaricot, R. Nitschke and T. Nann, Quantum dots versus organic dyes as fluorescent labels, Nat. Methods, 2008, 5, 763–775 CrossRef CAS;
(b) M. Bruchez, Jr., M. Moronne, P. Gin, S. Weiss and A. P. Alivisatos, Semiconductor Nanocrystals as Fluorescent Biological Labels, Science, 1998, 281, 2013–2016 CrossRef.
-
(a) X. Li, F.-J. Kao, C.-C. Chuang and S. He, Enhancing fluorescence of quantum dots by silica-coated gold nanorods under one- and two-photon excitation, Opt. Express, 2010, 18, 11335–11346 CrossRef CAS;
(b) N. Liu, B. S. Prall and V. I. Klimov, Hybrid Gold/Silica/Nanocrystal-Quantum-Dot Superstructures: Synthesis and Analysis of Semiconductor–Metal Interactions, J. Am. Chem. Soc., 2006, 128, 15362–15363 CrossRef CAS.
-
(a) T. Mokari, E. Rothenberg, I. Popov, R. Costi and U. Banin, Selective Growth of Metal Tips onto Semiconductor Quantum Rods and Tetrapods, Science, 2004, 304, 1787–1790 CrossRef CAS;
(b) T. Mokari, C. G. Sztrum, A. Salant, E. Rabani and U. Banin, Formation of asymmetric one-sided metal-tipped semiconductor nanocrystal dots and rods, Nat. Mater., 2005, 4, 855–863 CrossRef CAS.
-
(a) J. Yang, E. Sargent, S. Kelley and J. Y. Ying, A general phase-transfer protocol for metal ions and its application in nanocrystal synthesis, Nat. Mater., 2009, 8, 683–689 CrossRef CAS;
(b) J. Yang, H. I. Elim, Q. Zhang, J. Y. Lee and W. Ji, Rational Synthesis, Self-Assembly, and Optical Properties of PbS–Au Heterogeneous Nanostructures via Preferential Deposition, J. Am. Chem. Soc., 2006, 128, 11921–11926 CrossRef CAS;
(c) W. Shi, H. Zeng, Y. Sahoo, T. Y. Ohulchanskyy, Y. Ding, Z. L. Wang, M. Swihart and P. N. Prasad, A General Approach to Binary and Ternary Hybrid Nanocrystals, Nano Lett., 2006, 6, 875–881 CrossRef CAS.
-
(a) B. Dubertret, M. Calame and A. J. Libchaber, Single-mismatch detection using gold-quenched fluorescent oligonucleotides, Nat. Biotechnol., 2001, 19, 365–370 CrossRef CAS;
(b) T. Pons, I. L. Medintz, K. E. Sapsford, S. Higashiya, A. F. Grimes, D. S. English and H. Mattoussi, On the Quenching of Semiconductor Quantum Dot Photoluminescence by Proximal Gold Nanoparticles, Nano Lett., 2007, 7, 3157–3164 CrossRef CAS.
- Y. Jin and X. Gao, Plasmonic fluorescent quantum dots, Nat. Nanotechnol., 2009, 4, 571–576 CrossRef CAS.
- X. Hong, J. Li, M. Wang, J. Xu, W. Guo, J. Li, Y. Bai and T. Li, Fabrication of Magnetic Luminescent Nanocomposites by a Layer-by-Layer Self-assembly Approach, Chem. Mater., 2004, 16, 4022–4027 CrossRef CAS.
-
(a) A. M. Derfus, W. C. W. Chan and S. N. Bhatia, Probing the Cytotoxicity of Semiconductor Quantum Dots, Nano Lett., 2003, 4, 11–18 CrossRef;
(b) A. Hoshino, K. Fujioka, T. Oku, M. Suga, Y. F. Sasaki, T. Ohta, M. Yasuhara, K. Suzuki and K. Yamamoto, Physicochemical Properties and Cellular Toxicity of Nanocrystal Quantum Dots Depend on Their Surface Modification, Nano Lett., 2004, 4, 2163–2169 CrossRef CAS;
(c) C. Kirchner, T. Liedl, S. Kudera, T. Pellegrino, A. Munoz Javier, H. E. Gaub, S. Stolzle, N. Fertig and W. J. Parak, Cytotoxicity of Colloidal CdSe and CdSe/ZnS Nanoparticles, Nano Lett., 2004, 5, 331–338 CrossRef.
-
(a) F. Erogbogbo, K.-T. Yong, I. Roy, G. Xu, P. N. Prasad and M. T. Swihart, Biocompatible Luminescent Silicon Quantum Dots for Imaging of Cancer Cells, ACS Nano, 2008, 2, 873–878 CrossRef CAS;
(b) J. H. Warner, A. Hoshino, K. Yamamoto and R. D. Tilley, Water-Soluble Photoluminescent Silicon Quantum Dots13, Angew. Chem., Int. Ed., 2005, 44, 4550–4554 CrossRef CAS.
-
(a) K. Sato, S. Yokosuka, Y. Takigami, K. Hirakuri, K. Fujioka, Y. Manome, H. Sukegawa, H. Iwai and N. Fukata, Size-Tunable Silicon/Iron Oxide Hybrid Nanoparticles with Fluorescence, Superparamagnetism, and Biocompatibility, J. Am. Chem. Soc., 2011, 133, 18626–18633 CrossRef CAS;
(b) F. Erogbogbo, K.-T. Yong, I. Roy, R. Hu, W.-C. Law, W. Zhao, H. Ding, F. Wu, R. Kumar, M. T. Swihart and P. N. Prasad,
In Vivo Targeted Cancer Imaging, Sentinel Lymph Node Mapping and Multi-Channel Imaging with Biocompatible Silicon Nanocrystals, ACS Nano, 2010, 5, 413–423 CrossRef.
- X. Zhang, M. Brynda, R. D. Britt, E. C. Carroll, D. S. Larsen, A. Y. Louie and S. M. Kauzlarich, Synthesis and Characterization of Manganese-Doped Silicon Nanoparticles: Bifunctional Paramagnetic-Optical Nanomaterial, J. Am. Chem. Soc., 2007, 129, 10668–10669 CrossRef CAS.
- F. Erogbogbo, C.-W. Chang, J. L. May, L. Liu, R. Kumar, W.-C. Law, H. Ding, K. T. Yong, I. Roy, M. Sheshadri, M. T. Swihart and P. N. Prasad, Bioconjugation of luminescent silicon quantum dots to gadolinium ions for bioimaging applications, Nanoscale, 2012, 4, 5483–5489 RSC.
- F. Erogbogbo, K.-T. Yong, R. Hu, W.-C. Law, H. Ding, C.-W. Chang, P. N. Prasad and M. T. Swihart, Biocompatible Magnetofluorescent Probes: Luminescent Silicon Quantum Dots Coupled with Superparamagnetic Iron(III) Oxide, ACS Nano, 2010, 4, 5131–5138 CrossRef CAS.
- C. Tu, X. Ma, P. Pantazis, S. M. Kauzlarich and A. Y. Louie, Paramagnetic, Silicon Quantum Dots for Magnetic Resonance and Two-Photon Imaging of Macrophages, J. Am. Chem. Soc., 2010, 132, 2016–2023 CrossRef CAS.
-
(a) J. S. Biteen, L. A. Sweatlock, H. Mertens, N. S. Lewis, A. Polman and H. A. Atwater, Plasmon-Enhanced Photoluminescence of Silicon Quantum Dots: Simulation and Experiment, J. Phys. Chem. C, 2007, 111, 13372–13377 CrossRef CAS;
(b) J. Kalkman, H. Gersen, L. Kuipers and A. Polman, Excitation of surface plasmons at a SiO2Ag interface by silicon quantum dots: experiment
and theory, Phys. Rev. B: Condens. Matter Mater. Phys., 2006, 73, 075317 CrossRef.
-
(a) X. Li, Y. He, S. S. Talukdar and M. T. Swihart, Process for Preparing Macroscopic Quantities of Brightly Photoluminescent Silicon Nanoparticles with Emission Spanning the Visible Spectrum, Langmuir, 2003, 19, 8490–8496 CrossRef CAS;
(b) X. Li, Y. He and M. T. Swihart, Surface Functionalization of Silicon Nanoparticles Produced by Laser-Driven Pyrolysis of Silane followed by HF–HNO3 Etching, Langmuir, 2004, 20, 4720–4727 CrossRef CAS.
-
(a) S. R. Whaley, D. S. English, E. L. Hu, P. F. Barbara and A. M. Belcher, Selection of peptides with semiconductor binding specificity for directed nanocrystal assembly, Nature, 2000, 405, 665–668 CrossRef CAS;
(b) J. M. Slocik, J. T. Moore and D. W. Wright, Monoclonal Antibody Recognition of Histidine-Rich Peptide Encapsulated Nanoclusters, Nano Lett., 2002, 2, 169–173 CrossRef CAS;
(c) R. Djalali, Y.-f. Chen and H. Matsui, Au Nanocrystal Growth on Nanotubes Controlled by Conformations and Charges of Sequenced Peptide Templates, J. Am. Chem. Soc., 2003, 125, 5873–5879 CrossRef CAS.
- W. Pham, W.-F. Lai, R. Weissleder and C.-H. Tung, High efficiency synthesis of a bioconjugatable near-infrared fluorochrome, Bioconjugate Chem., 2003, 14, 1048–1051 CrossRef CAS.
- F. J. Hua, M. T. Swihart and E. Ruckenstein, Efficient surface grafting of luminescent silicon quantum dots by photoinitiated hydrosilylation, Langmuir, 2005, 21, 6054–6062 CrossRef CAS.
- H. Ding, K.-T. Yong, W.-C. Law, I. Roy, R. Hu, F. Wu, W. Zhao, K. Huang, F. Erogbogbo, E. J. Bergey and P. N. Prasad, Non-invasive tumor detection in small animals using novel functional Pluronic nanomicelles conjugated with anti-mesothelin antibody, Nanoscale, 2011, 3, 1813–1822 RSC.
- H. Ding, K.-T. Yong, I. Roy, H. E. Pudavar, W. C. Law, E. J. Bergey and P. N. Prasad, Gold Nanorods Coated with Multilayer Polyelectrolyte as Contrast Agents for Multimodal Imaging, J. Phys. Chem. C, 2007, 111, 12552–12557 CAS.
-
(a) K. Cho, X. Wang, S. Nie, Z. Chen and D. M. Shin, Therapeutic Nanoparticles for Drug Delivery in Cancer, Clin. Cancer Res., 2008, 14, 1310–1316 CrossRef CAS;
(b) F. Yuan, M. Dellian, D. Fukumura, M. Leunig, D. A. Berk, V. P. Torchilin and R. K. Jain, Vascular Permeability in a Human Tumor Xenograft: Molecular Size Dependence and Cutoff Size, Cancer Res., 1995, 55, 3752–3756 CAS.
- K. Atcha, Y. Ken-Tye, H. Rui, R. Indrajit, D. Hong, A. V. Lisa, J. B. Earl and N. P. Paras, Biocompatible PEGylated gold nanorods as colored contrast agents for targeted in vivo cancer applications, Nanotechnology, 2010, 21, 315101 CrossRef.
- W. Shi, Y. Sahoo, M. T. Swihart and P. N. Prasad, Gold Nanoshells on Polystyrene Cores for Control of Surface Plasmon Resonance, Langmuir, 2005, 21, 1610–1617 CrossRef CAS.
- M.-C. Daniel and D. Astruc, Gold Nanoparticles: Assembly, Supramolecular Chemistry, Quantum-Size-Related Properties, and Applications toward Biology, Catalysis, and Nanotechnology, Chem. Rev., 2003, 104, 293–346 CrossRef.
- K. Huang, H. Ma, J. Liu, S. Huo, A. Kumar, T. Wei, X. Zhang, S. Jin, Y. Gan, P. C. Wang, S. He, X. Zhang and X.-J. Liang, Size-Dependent Localization and Penetration of Ultrasmall Gold Nanoparticles in Cancer Cells, Multicellular Spheroids, and Tumors in Vivo, ACS Nano, 2012, 6, 4483–4493 CrossRef CAS.
|
This journal is © The Royal Society of Chemistry 2013 |
Click here to see how this site uses Cookies. View our privacy policy here.