ZnO nanoparticle fate in soil and zinc bioaccumulation in corn plants (Zea mays) influenced by alginate
Received
27th July 2012
, Accepted 24th October 2012
First published on 23rd November 2012
Abstract
Nanoparticles (NPs) can interact with naturally occurring inorganic and organic substances in soils, which may change their transport behavior in soil and plants. This study was performed in two steps. In the first step, corn (Zea mays) plants were cultivated for one month in soil amended with 10 nm commercial spheroid ZnO NPs at 0–800 mg kg−1 and sodium alginate at 10 mg kg−1. In the second step, the plants were grown with ZnO NPs at 400 mg kg−1 and alginate at 0, 10, 50, and 100 mg kg−1. The dynamics of Zn concentrations in soil solution and Zn accumulation in plant tissues were determined by ICP-OES. Biomass accumulation, chlorophyll concentration, and the activity of antioxidant enzymes in leaves were also quantified. Results indicate that ZnO NPs coexisting with Zn dissolved species were continuously released to the soil solution to replenish the Zn ions or ZnO NPs scavenged by roots. At 400 and 800 mg kg−1, without alginate, ZnO NPs significantly reduced the root and shoot biomass production; however, plants treated with these NP concentrations, plus alginate, had significantly more Zn in tissues with no reduction in biomass production. Alginate significantly reduced the activity of stress enzymes catalase and peroxidase, which could indicate damage in the defense system. The effects of ZnO NPs in a food crop grown in alginate enriched soil, showing an excess of Zn in the aerial parts, are yet to be reported.
Environmental impact
The environmental impacts of ZnO nanoparticles are still not well understood. The data on the effects of ZnO nanoparticles in terrestrial plants are very limited. Moreover, the effects of organic matter on the fate in soil of the ZnO NPs and the Zn uptake by a food crop are yet to be reported. Alginate, a component of the natural organic matter, can modulate the fate and effects of ZnO NPs in plants. In this research we found that alginate changes the fate of ZnO nanoparticles in both soil and plants. Alginate increases the release of Zn ions to soil solution. We also found that alginate significantly reduced the activity of the stress enzymes catalase and peroxidase, which could indicate damage in the defense system.
|
1. Introduction
Zinc oxide nanoparticles (ZnO NPs) are profusely used in personal care products, environmental remediation, pharmaceuticals, and catalysis.1,2 The increased use of ZnO NPs has raised concerns regarding their fate and behavior in the natural ecosystem and possible toxicity to producers, consumers, and primary detritus feeders.3–6 The natural ecosystem contains natural organic matter (NOM) mostly comprised of humic substances and polysaccharides.7 As previously reported, NOM adsorbs to the surface of natural colloids and engineered nanomaterials (ENMs), influencing their surface properties, fate, and transport.8,9 Alginic acid, a component of NOM, is a prevailing environmental polymer produced by bacteria like Azotobacter vinelandii and the omnipresent environmental bacterium Pseudomonas aeruginosa.10,11 It is a hydrophilic, colloidal, polyuronic acid set in three combinations of disaccharide subunits.10 Functional groups in alginate species can exhibit strong affinity to Zn surface atoms or certain crystallographic planes.12 The sodium salt of alginic acid, sodium alginate, has been used as a capping agent to stabilize NPs in suspensions.8 Sodium alginate can stabilize the hydrodynamic particle diameter of Co3O4, CuO, NiO, and ZnO NPs in suspensions to approximately 200–400 nm.8 Researchers have also used sodium alginate to stabilize NPs for several applications in soils.13–16 However, to the authors' knowledge, the fate and behavior of ENMs in soil and plants in the presence of alginate has not been studied yet. Alginate can wrap the NPs entering into soils, modifying their behavior and toxicity to plants.8,17,18
Understanding the interactions between ENMs and plants is crucial in comprehending the impact of nanotechnology on the environment and agriculture.19 Previous reports have revealed that in hydroponically grown plants, ZnO NPs reduce seed germination20 and enter root cells, damaging tissues.4 Other reports have shown that ZnO NPs reduce root elongation in mesquite (Prosopis juliflora-velutina), blue palo verde (Parkinsonia florida), and tumbleweed (Salsola tragus), with a 50% inhibitory concentration for mesquite root elongation between 1000 and 2000 mg ZnO NPs per L. However, it seems that the results from soil studies are associated with the soil condition and plant species. Kim et al.21 reported that ZnO NPs at 2000 mg kg−1 did not affect the root length and biomass production of Cucumis sativus grown in a loamy sand soil (pH 5.5). However, ZnO NPs at 1000 mg kg−1 reduced the shoot length in buckwheat (Fagopyrum esculentum) also grown in a loamy sand soil (pH 5.5 ± 0.2).24 Another report indicates that ZnO NPs at 286 mg kg−1 affected the root elongation in Lipidium sativum sown in an artificial standard soil.22 But at only 45.45 mg kg−1 (5 g per 110 kg soil), these NPs reduced the biomass production of wheat (Triticum aestivum) cultivated in loamy clay soil (pH 7.36).23 In some of these previous studies, X-ray absorption spectroscopy (XAS) analyses demonstrated no presence of ZnO NPs within roots.25–27 However, Zhao et al., by using a confocal microscope, imaged the presence of FITC-stained ZnO NPs in the stele of corn roots, although no presence of ZnO NPs was found in the shoots.28 This could indicate that ZnO NPs would be absorbed and incorporated into the transport system of plants, reaching the harvestable food supply.
To the authors' knowledge, it is still unknown how the naturally occurring alginate in soil changes the fate and toxicity of ZnO NPs to plants. The bioavailability of NPs depends on the type of association with soil components and their concentration in soil solution. The objectives of this study were to determine: (1) the influence of sodium alginate on Zn release to soil solution from ZnO NPs, (2) the impact of alginate on ZnO NPs coexisting with Zn dissolved species uptake and translocation from roots to shoot in corn plants, and (3) the effect of ZnO NPs on plant growth and stress response with/without alginate in soil.
2. Materials and methods
2.1 Characteristics of ZnO NP and soil
The ZnO NPs (10 nm commercial spheroid, Meliorum Technologies, New York) were obtained from the University of California Center for Environmental Implications of Nanotechnology (UC CEIN). Hydrodynamic size, ζ-potential and pH of the ZnO NPs at various ionic strengths were determined and reported in a previously published article.28,29 For the present study, the particle size and ζ-potential (Nano-ZS 90, Malvern), as well as the pH of the soil solution, were determined at a NP concentration of 80 mg L−1. Without alginate the size and ζ-potential were 1816 ± 98 nm and −12.4 ± 3.76 mV, respectively. On the other hand, with alginate they were 475 ± 36 nm and −28.2 ± 4.51 mV, respectively.
The soil for this study was collected from Horizon, TX (31°51′59.06′′N; top 20 cm), air-dried and sieved through a 2 mm mesh prior to experimental use. The soil was classified as sandy loam soil with percentages of clay, silt, and sand of 3.73%, 12.15%, and 84.1%, respectively. Because the Horizon soil has a very fine particle size and very low organic matter content, the experiments were performed in a 1
:
1 mixture of the native soil with high organic matter soil (Scotts, premium potting soil) purchased from a nursery store to keep suitable pore size and enhance soil fertility. The soil properties after mixture were: pH 7.9 ± 0.3, CEC 8.07, organic matter content 0.04%, and Zn concentration 28 mg kg−1.
The extraction of soil solution was performed according to Krishnamurti and Naidu30 with minor modifications. Briefly, 10 mL of a stock solution of ZnO NPs at 80 mg L−1 were added to 1 g of soil (without alginate and with alginate at 10 mg kg−1) to bring the soil to water saturation. The initial soil solution pH was 7.62. However, after the addition of the ZnO NPs, the pH increased to 8.18 but decreased to 6.78 in samples treated with ZnO NPs and alginate.
The mixtures were shaken for 14 days and triplicate samples were taken every two days to analyze the Zn concentration. To mimic the Zn extraction by plants, a separate set of samples were used to determine the Zn replenishment to the soil solution from the ZnO NPs. For this experiment, the supernatant was removed every two days from the reaction tubes and refilled with 10 mL of Millipore water (MPW) until the end of the experiment. In both cases the soil solutions were centrifuged at 8000 rpm for 10 min (Marathon 8K, Fisher Scientific); the collected supernatants were passed through 0.45 μm Millipore filters (Whatman) and the Zn concentrations were determined by ICP-OES (Perkin-Elmer Optima 4300 DV, Shelton, CT). The results represent the sum of all zinc in suspension, including zinc ions, ZnO NPs, and other forms of Zn.
2.3 Effects of sodium alginate on Zn uptake by corn plants
To evaluate how the alginate in soil influences the uptake and accumulation of Zn in corn (Zea mays, Golden variety), Magenta boxes were filled out with 400 g of soil (with/without alginate) and treated with ZnO NPs at 0, 100, 200, 400, and 800 mg kg−1. For this experiment the alginate content in soil was 10 mg kg−1. Each pot was sown with nine corn kernels and irrigated with tap water when needed to keep the soil close to field capacity. Plants were grown for 30 days at room temperature (23° ± 1°) and kept under a 16/8 h light/dark photoperiod produced by four 40 Watt Phillips lamps. At harvest, plants were thoroughly rinsed with tap water followed by deionized water. Roots and shoots were separated and oven-dried at 60 °C for 24 h and weighed. Dried ground root and shoot samples were microwave digested using a microwave oven (CEM Corporation Mathews, NC) following the EPA method 3051 (USEPA, 1994). Zn concentrations in the digest solution were determined using ICP-OES. The Certified Reference Material (Peach leaves, NIST 1547) was processed as samples and the recovery rate was 100.82 ± 0.48.
2.4 Effects of sodium alginate on chlorophyll content in corn leaves
In order to investigate the effect of ZnO NPs on chlorophyll production under alginate presence, the 400 mg kg−1 concentration was combined with alginate at 0, 10, 50, and 100 mg kg−1. Fresh leaves were collected from one month old corn plants to determine the chlorophyll concentration. Leaves were weighed and homogenized in 2 mL of 80% acetone in the presence of MgCO3 in a dark room at 25 °C ± 1. The extracted solutions were centrifuged at 11
000 rpm for 10 min (Eppendorf 5417R, Hamburg, Germany). Chlorophyll concentration was determined by measuring solution absorbance at 663 and 645 nm using a spectrophotometer (Thermo Spectronic GENESYS, Rochester, NY). The concentration of chlorophyll was calculated according to the following formula:31 | Ca = 12.72A663 − 2.59A645 | (1) |
| Cb = 22.88A645 − 4.67A663 | (2) |
where Ca stands for chlorophyll a, Cb for chlorophyll b, and A for absorbance.
2.5 Effects of sodium alginate on antioxidant enzyme activity
The activity of the antioxidant enzymes catalase (CAT) and ascorbate peroxidase (APOX) was determined in leaves of plants grown for 30 days with ZnO NPs at 400 mg kg−1 and alginate at various concentrations. The CAT enzyme activity was assayed following the procedure described by Gallego et al.,32 modified by de la Rosa et al.27 Briefly, extractions were performed in a cold room at 4 °C. A ratio of 10% w/v of the sample was homogenized with phosphate buffer (25 mM KH2PO4 at pH 7.4). The sample was centrifuged for 5 min at 10
000 rpm on a bench centrifuge (Eppendorf 5417R, Hamburg, Germany). A sample of 50 μL of the supernatant and 950 μL of 10 mM H2O2 was placed in a quartz cuvette to obtain a final volume of 1 mL. This sample was mixed by hand (shaken three times) and the change in the absorbance was measured during a 3 min period at 240 nm using a Perkin Elmer Lambda 14 UV/Vis spectrometer (single beam mode, Perkin Elmer, Uberlinger, Germany). The absorbance values were obtained from the first linear section of slope between 0.5 and 2 min. The extinction coefficient for H2O2 was set at 23.148 mM−1 cm−1.
APOX activity was determined according to Murgia et al.33 In order to attain an increased signal to noise ratio, we changed the volume of the sample from the previous method to 100 μL. A volume of 886 μL of 0.1 M KH2PO4 buffer at pH 7.4, 10 μL of 17 mM H2O2, 100 μL of the sample, and 4 μL of a 25 mM solution of ascorbate were placed in a quartz cuvette for a total volume of 1 mL. The change in absorbance was recorded during a two minute period at 265 nm in a Perkin Elmer Lambda 14 UV/Vis spectrometer. The absorbance was recorded as described above and the extinction coefficient was experimentally set at 22.21 mM−1 cm−1.
2.6 Statistical analysis
Zinc concentrations in soil solution, roots, and shoots are averages of three replicates. A one-way ANOVA test was performed followed by the Tukey-HSD test performed using the statistical package SPSS Version 12.0 (SPSS, Chicago, IL). The statistical significance was based on probabilities of p ≤ 0.05.
3. Results and discussion
3.1 Zn concentration in soil solution
A variation of the total Zn concentration as a function of contact time with soil is shown in Fig. 1. The concentration in control soil (without NPs, not shown in the figure) was 0.24 μg per 10 mL. On the other hand, in ZnO NP treated soil without extraction, Zn concentration in solutions in both alginate and non-alginate treatments increased until the fourth day, remaining constant thereafter, until the end of the experiment (14 days). As shown in Fig. 1A, in all cases the presence of alginate in the soil significantly increased the concentration of Zn in solution. A possible explanation for the high concentration of Zn in the soil solution in alginate treatments is that alginate changed the surface chemistry of ZnO NPs. Moreover, the probable formation of Zn-alginates, based on the well-known fact of gel formation by reaction of alginic acid or Na-alginate with divalent cations, could have also increased Zn in the soil solution. The ζ-potential of ZnO NPs in the soil solution without alginate was −12.4 ± 3.76, while with alginate it was −28.2 ± 4.51. It should be expected that negatively charged soil clay minerals would easily reject the more negative particles in alginate treated samples. These particles in solution can saturate the available binding sites for Zn in the soil clay minerals, thus leaving the Zn in solution. In the present study, the pH value of soil solutions was monitored for 14 days (Table 1). No significant difference was observed between alginate and non-alginate treated samples. Table 1 also shows that the pH of soil solutions slightly decreased as the reaction time increased. The slight change in pH could be associated with the slight increase of Zn in solution (Fig. 1A). The Zn cation is a strong Lewis acid.34
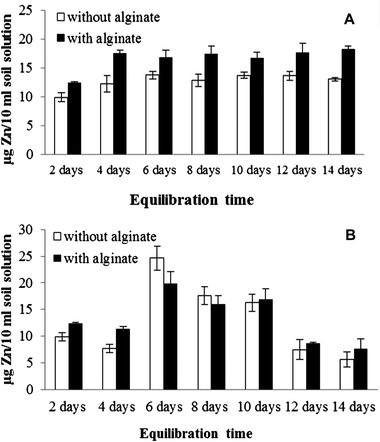 |
| Fig. 1 Variation of the total Zn concentration as a function of contact time in soil solutions spiked with 800 mg kg−1 of ZnO NPs with or without alginate. (A) No addition of fresh water after sample withdrawal and (B) with addition of fresh water after sample withdrawal. Data are the average of three replicates. Error bars stand for standard deviation. | |
Table 1 pH values of soil solutions from soil treated with ZnO NPs at 800 mg kg−1 in the presence/absence of sodium alginate
|
2 days |
4 days |
6 days |
8 days |
10 days |
12 days |
14 days |
ZnO NP without alginate |
8.01 (±0.10) |
8.20 (±0.13) |
8.34 (±0.16) |
8.08 (±0.28) |
7.94 (±0.08) |
7.60 (±0.01) |
7.51 (±0.12) |
ZnO NP with alginate |
7.97 (±0.22) |
8.11 (±0.11) |
8.18 (±0.14) |
7.96 (±0.01) |
7.94 (±0.06) |
7.62 (±0.02) |
7.53 (±0.08) |
To mimic the dynamics of Zn in soil solution under the presence of plants (continuous extraction) the supernatant was removed every two days from the reaction tubes and refilled with MPW (Fig. 1B). As one can see in this figure, no statistically significant changes were observed in the first two sampling times (second and fourth days). However, there was an increase up to the sixth day and, thereafter, there was a reduction trend in the Zn concentration. The highest peak at the sixth day could indicate the highest release of Zn ions from the NPs. The dissolved Zn and the ZnO NPs in soil solution would be utilized by the plants and replenished by ZnO NPs coexisting with Zn dissolved species released from the clay minerals. Electron microprobe mapping showed that ZnO NPs coexisting with Zn dissolved species associate with silica and aluminum, which are indicators of soil clay minerals.35 As should be expected, as the time passed, without the application of more Zn, the plants would reduce the concentration of Zn in the soil solution. In order to further explain this, the total Zn released was calculated. As can be seen from Fig. 2A and B, Zn concentrations without frequent extraction were constant, with/without alginate. However, in both alginate and no alginate treatments, continuous Zn extraction from the soil solution leads to more Zn released into the soil solution. Thus, the removal of Zn from the soil solution induces the release of Zn from the matrix. A schematic representation of the process is shown in Fig. 3. In this figure, the clay particle in the soil solution acts as the matrix (Zn and ZnO NPs repository) and the roots represent the sink.
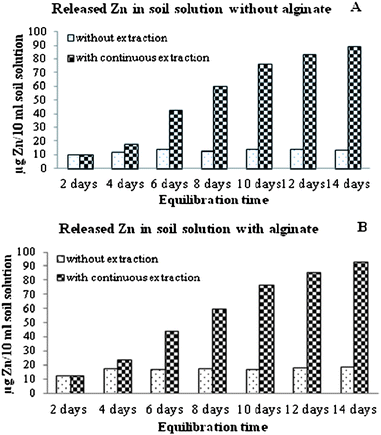 |
| Fig. 2 Variation in the accumulative total Zn concentration in soil solution as a function of contact time. (A) without alginate and (B) with alginate in soil. | |
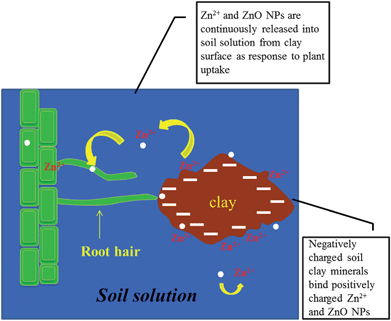 |
| Fig. 3 Schematic representation of the dynamics of Zn in soil solution and the uptake process by plants. | |
3.2 Uptake of Zn by corn plants
Concentrations of Zn in corn roots and shoots are shown in Fig. 4A and B, respectively. As shown in these figures, total Zn in roots and shoots increased as the ZnO NP concentration in soil increased. Zn concentrations in roots were 187–382 mg kg−1 and 112–769 mg kg−1 for non-alginate and alginate treated soil with ZnO NPs at 100 to 800 mg kg−1, respectively, in one month cultivation. Zn accumulation in shoot was 148–400 mg kg−1 and 174–495 mg kg−1, respectively, in soil without alginate and with alginate, when ZnO NPs varied from 100 to 800 mg kg−1. The translocation factors of Zn were calculated to be 0.78–0.91 in the present study, which were higher than those reported in a previous study (0.02–0.01).4 A possible reason is that Zn/ZnO NPs are not easily transported from root to shoot in ryegrass (Lolium perenne).4 It is noted that in the present work, the translocation factors of Zn under all ZnO NP treatments was lower than the control (1.78). Since there were no ZnO NPs in the control soil, the accumulated Zn in the control plants must have come from indigenous Zn ions. In our previous work, by using confocal microscopy, we found that FITC-stained NPs penetrate the transport system of corn roots, but there was no presence of ZnO NPs in the leaf tissues, which suggests that ZnO NPs dissolve inside corn roots, transporting the ionic Zn to the upper part of the plants.28 It is possible that the NPs within corn roots have a very slow dissolution rate, resulting in a poor Zn translocation to the shoots.
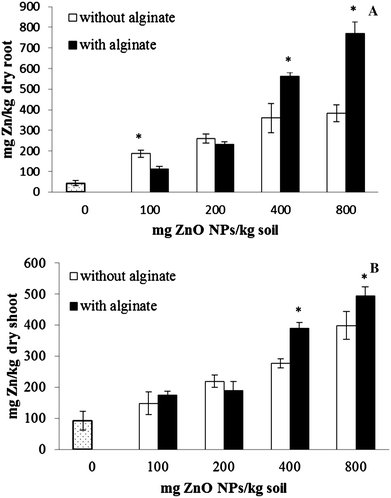 |
| Fig. 4 Average Zn concentrations in roots (A) and shoots (B) of corn plants grown for 30 days in soil treated with ZnO NPs from 0 to 800 mg kg−1 with and without alginate. Data are the average of three replicates. Error bars stand for standard deviation. *Significantly different (p ≤ 0.05). | |
The results shown in Fig. 4 suggest that the alginate amendment to the soil influences the uptake of Zn by corn plants. At low ZnO NP concentrations (100 mg kg−1) the roots of the plants treated with NPs in soil without alginate had significantly more Zn compared to roots of plants grown in soil with alginate (Fig. 4A). However, at high concentrations (400 and 800 mg kg−1), the roots of plants grown with the same ZnO NP concentrations, in the presence of alginate, had significantly more Zn (p ≤ 0.05) than the roots of plants grown without alginate. This could be due to differences in particle size. Without alginate the size of the particles in solution was 1816 ± 98 nm and under alginate treatment the size was 475 ± 36 nm. A similar trend was observed in shoot results (Fig. 4B), except for the treatment of 100 mg kg−1. The data suggest that naturally occurring organic matter (polysaccharides) in soil might play an important role in the uptake and transport of Zn in plants exposed to ZnO NPs. Zeng et al.36 reported that Zn concentration in rice straw was significantly and positively correlated with soil organic matter content.
3.3 Effect of alginate on corn plant biomass production
Biomass production is usually an indicator of plant health. As shown in Fig. 5A and B, the dry weight of plants grown in soil treated with 400 to 800 m kg−1 of ZnO NPs decreased by 34% and 30% in root and 21% and 26% in shoot, respectively, in soil without alginate. This suggests that high amounts of ZnO NPs are toxic to plants as the biomass production was diminished. Lin and Xing4 also reported that uncoated ZnO NPs reduced the biomass production in ryegrass. In the present study, ZnO NPs did not reduce the biomass production in plants grown in soil amended with alginate. This could be due to the fact that alginate significantly increased the level of chlorophyll a (p ≤ 0.05) (Fig. 6). Thus, the data suggest that the biomass production was not affected by ZnO NPs in alginate enriched soil because it helps to maintain photosynthesis. Previous reports indicate that alginate promotes plant growth.37
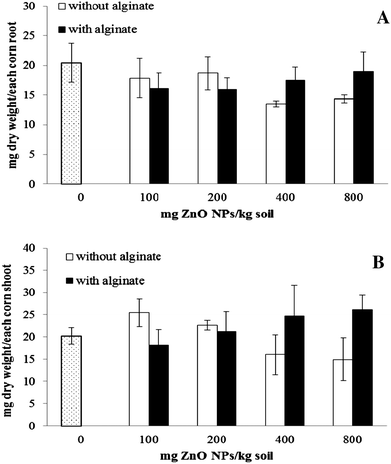 |
| Fig. 5 Biomass accumulation in root (A) and shoot (B) of corn plants grown for 30 days in soil treated with ZnO NPs at 0 to 800 mg kg−1 with and without alginate. Error bars stand for standard deviation. | |
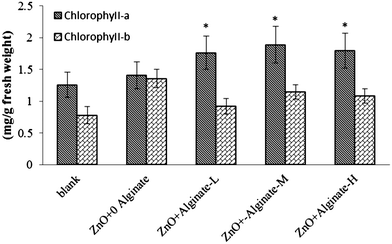 |
| Fig. 6 Chlorophyll concentration of corn plant leaves treated with 400 mg kg−1 ZnO NPs at low (10 mg kg−1, L), medium (50 mg kg−1, M) and high (100 mg kg−1, H) alginate levels. *Significantly different (p ≤ 0.05). | |
3.4 Effect of alginate on chlorophyll concentration in corn plants
To elucidate if the un-suppressed biomass production under the presence of alginate might be attributed to positive effects of alginate on chlorophyll production, corn plants were allowed to grow for one month with different alginate levels, and the fresh leaves were collected to measure chlorophyll concentration in leaves. From Fig. 6 it can be seen that alginate significantly increased the level of chlorophyll a (p ≤ 0.05). This could be a reason why the biomass production was not reduced in alginate treated corn plants. Foliar applications of irradiated sodium alginate improved the growth attributes, photosynthetic capability, and enzyme activities in Artemisia annua L.38
3.5 Antioxidant enzyme activity assays
Studies have shown that the formation of reactive oxygen species (ROS) can be triggered by NPs.39,40 The generated ROS molecules can damage DNA, proteins, and lipids. The enzymes CAT and APOX are involved in the detoxification of H2O2, thereby preventing the formation of ˙OH radicals.41 In mesquite, a shrub/tree, Hernandez-Viezcas et al.26 found that ZnO NPs increased CAT in roots, stems, and leaves, while APOX increased only in stems and leaves. In the present study, CAT and APOX activity in all corn plants treated with the ZnO NPs was reduced compared with the control, which could indicate damage in the defense system of corn plants. CAT and APOX activity was further reduced in alginate treated soil (Fig. 7). This suggests that alginate can maintain the photosynthesis but it could magnify the damage in the defensive system. This could be explained by the increase in Zn concentration in plant tissues promoted by alginate. Previous studies42 have shown that CAT activity was significantly decreased in maize plants treated with 1000 mg Zn kg−1. Further research is needed in order to increase the knowledge on the physiological impacts of ZnO NPs in plants grown in an organic matter enriched soil.
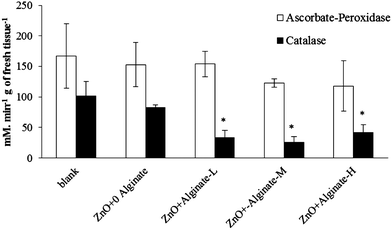 |
| Fig. 7 Antioxidant enzyme (catalase and ascorbate peroxidase) level in corn leaves treated with 400 mg kg−1 ZnO NPs and low (10 mg kg−1, L), medium (50 mg kg−1, M) and high (100 mg kg−1, H) alginate levels. *Significantly different (p ≤ 0.05). | |
4. Conclusion
Results of this research have shown that, in ZnO NP impacted soil, alginate increases the release of Zn ions to soil solution and promotes the bioaccumulation of Zn in corn plant tissues. However, the decrease in CAT and APOX activities could compromise the defense against the ZnO NPs toxicity. This study has shown, for the first time, that alginate, a natural component of natural ecosystems, could affect the fate of ZnO NPs in both soil and plants. Further experiments are needed to determine the effects of alginate on the impacts of ZnO NPs in plants grown to maturity.
Acknowledgements
This material is based on work supported by the National Science Foundation and the Environmental Protection Agency under Cooperative Agreement Number DBI-0830117. Any opinions, findings, and conclusions or recommendations expressed in this material are those of the author(s) and do not necessarily reflect the views of the National Science Foundation or the Environmental Protection Agency. This work has not been subjected to EPA review and no official endorsement should be inferred. The authors also acknowledge USDA grant number 2011-38422-30835 and NSF Grant # CHE-0840525. J. L. Gardea-Torresdey acknowledges the Dudley family for the Endowed Research Professorship in Chemistry.
References
- N. M. Franklin, N. J. Rogers, S. C. Apte, G. E. Batley, G. E. Gadd and P. S. Casey, Environ. Sci. Technol., 2007, 41, 8484 CrossRef CAS.
- K. G. Scheckel, T. P. Luxton, A. M. E. Badawy, C. A. Impellitteri and T. M. Tolaymat, Environ. Sci. Technol., 2010, 44, 1307 CrossRef CAS.
- C. Buzea, I. Pacheco and K. Robbie, Biointerphases, 2007, 2, 17 CrossRef.
- D. Lin and B. Xing, Environ. Sci. Technol., 2008, 42, 5 Search PubMed.
- C. M. Rico, S. Majumdar, M. Duarte-Gardea, J. R. Peralta-Videa and J. L. Gardea-Torresdey, J. Agric. Food Chem., 2011, 59, 3 CrossRef.
- J. R. Peralta-Videa, L. Zhao, M. L. Lopez-Moreno, G. de la Rosa, J. Hong and J. L. Gardea-Torresdey, J. Hazard. Mater., 2011, 186, 1 CrossRef CAS.
- K. J. Wilkinson, E. Balnois, G. G. Leppard and J. Buffle, Colloids Surf., A, 1999, 155, 287 CrossRef CAS.
- K. L. Chen, S. E. Mylon and M. Elimelech, Environ. Sci. Technol., 2006, 40, 1516 CrossRef CAS.
- D. P. Stankus, S. E. Lohse, E. J. Hutchison and J. A. Nason, Environ. Sci. Technol., 2011, 45, 3238 CrossRef CAS.
- T. D. Perry, R. T. Cygan and R. Mitchell, Geochim. Cosmochim. Acta, 2006, 70, 3508 CrossRef CAS.
- K. L. Chen and M. Elimelech, Environ. Sci. Technol., 2008, 42, 7607 CrossRef CAS.
- S. Lin, Y. Zhao, T. Xia, H. Meng, Z. Ji, R. Liu, S. George, S. Xiong, X. Wang, H. Zhang, S. Pokhrel, L. Madler, R. Damoiseaux, S. Lin and A. E. Nel, ACS Nano, 2010, 5, 7284 CrossRef.
- X. Li, X. Kong, S. Shi, X. Zheng, G. Guo, Y. Wei and Z. Qian, Biotechnology, 2008, 8, 1 Search PubMed.
- B. Chico, C. Camacho, M. Pérez, M. A. Longo, M. A. Sanromán, J. M. Pingarrón and R. Villalonga, J. Electroanal. Chem., 2009, 629, 126 CrossRef CAS.
- M. Fayaz, K. Balaji, M. Girilal, P. T. Kalaichelvan and R. Venkatesan, J. Agric. Food Chem., 2009, 57, 6246 CrossRef.
- M. dos Santos Silva, D. Sgarbi Cocenza, R. Grillo, N. Ferreira Silva de Melo, P. Sérgio Tonello, L. Camargo de Oliveira, D. Lopes Cassimiro, A. H. Rosa and L. Fernandes Fraceto, J. Hazard. Mater., 2011, 190, 366 CrossRef.
- F. Mafune, J. Kohno, Y. Takeda, T. Kondow and H. Sawabe, J. Phys. Chem. B, 2000, 104, 8333 CrossRef CAS.
- S. Lin, J. Reppert, Q. Hu, J. S. Hunson, M. L. Reid and T. Ratnikova, Small, 2009, 5, 1128 CrossRef CAS.
- M. V. Khodakovskaya, K. de Silva, D. A. Nedosekin, E. Dervishi, A. S. Biris, E. V. Shashkov, E. I. Galanzha and V. P. Zharov, Proc. Natl. Acad. Sci. U. S. A., 2011, 108, 1028 CrossRef CAS.
- D. Lin and B. Xing, Environ. Pollut., 2007, 150, 243 CrossRef CAS.
- S. Kim, J. Kim and I. Lee, Chem. Ecol., 2011, 27, 49 CrossRef CAS.
- S. Manzo, A. Rocco, R. Carotenuto, F. DeLuca Picione, M. L. Miglietta, G. Rametta and G. Di Francia, Environ. Sci. Pollut. Res., 2011, 18, 756 CrossRef CAS.
- W. Du, Y. Sun, R. Ji, J. Zhu, J. Wu and H. Guo, J. Environ. Monit., 2011, 13, 822 RSC.
- S. Lee, S. Kim, S. Kim and I. Lee, J. Microbiol. Biotechnol., 2012, 22, 12–64 Search PubMed.
- M. L. Lopez-Moreno, G. de la Rosa, J. A. Hernandez-Viezcas, H. Castillo-Michel, C. E. Botez, J. R. Peralta-Videa and J. L. Gardea-Torresdey, Environ. Sci. Technol., 2010, 44, 7315 CrossRef CAS.
- J. A. Hernandez-Viezcas, H. Castillo-Michel, A. D. Servin, J. R. Peralta-Videa and J. L. Gardea-Torresdey, Chem. Eng. J., 2011, 170, 346 CrossRef CAS.
- G. de la Rosa, M. L. López-Moreno, J. Hernández-Viescaz, J. R. Peralta-Videa and J. L. Gardea-Torresdey, Int. J. Nanotechnol., 2011, 8, 492 CrossRef CAS.
- L. Zhao, J. R. Peralta-Videa, M. Ren, A. Varela-Ramirez, C. Li, J. A. Hernandez-Viezcas, R. J. Aguilera and J. L. Gardea-Torresdey, Chem. Eng. J., 2012, 184, 1 CrossRef CAS.
- A. A. Keller, H. Wang, D. Zhou, H. S. Lenihan, G. Cherr, B. J. Cardinale, R. Miller and Z. Ji, Environ. Sci. Technol., 2010, 44, 1962 CrossRef CAS.
- G. S. R. Krishnamurti and R. Naidu, Environ. Sci. Technol., 2002, 36, 2645 CrossRef CAS.
-
H. S. Li, Principles and Techniques of Plant Physiological Biochemical Experiment, Higher Education Press, Beijing, China, 2000 Search PubMed.
- S. M. Gallego, M. P. Benavides and M. L. Tomaro, Plant Sci., 1996, 121, 151 CrossRef CAS.
- I. Murgia, D. Tarantino, C. Vannini, M. Bracale, S. Carravieri and C. Soave, Plant J., 2004, 38, 940 CrossRef CAS.
-
G. Rayner-Canham, Descriptive Inorganic Chemistry, W. H. Freeman, New York, 2nd edn, 1999 Search PubMed.
- L. Zhao, J. R. Peralta-Videa, J. A. Hernandez-Viezcas, J. Hong and J. L. Gardea-Torresdey, J. Nano Res., 2012, 17, 229 CrossRef CAS.
- F. Zeng, S. Ali, H. Zhang, Y. Ouyang, B. Qiu, F. Wu and G. Zhang, Environ. Pollut., 2011, 159, 84 CrossRef CAS.
- N. Q. Hien, N. Nagasawa, L. X. Tham, F. Yoshii, V. H. Dang, H. Mitomo, K. Makuuchi and T. Kume, Radiat. Phys. Chem., 2000, 59, 97 CrossRef CAS.
- T. Aftab, M. M. Khan, M. Idrees, M. Naeem, Moinuddin, N. Hashmi and L. Varshney, Radiat. Phys. Chem., 2011, 80, 833 CrossRef CAS.
- G. Oberdorster, E. Oberdorster and J. Oberdorster, Environ. Health Perspect., 2005, 113, 823 CrossRef CAS.
- A. Nel, T. Xia, L. Madler and N. Li, Science, 2006, 311, 622 CrossRef CAS.
- M. Rodriguez-Serrano and M. C. Romero-Puertas, Plant Physiol., 2009, 150, 229 CrossRef CAS.
- Y. Cui and N. Zhao, Plant, Soil Environ., 2011, 57, 34 CAS.
|
This journal is © The Royal Society of Chemistry 2013 |