DOI:
10.1039/C2RA21208K
(Paper)
RSC Adv., 2012,
2, 8061-8070
Quinolinium and isoquinolinium ionic liquid crystals†
Received
19th June 2012
, Accepted 20th June 2012
First published on 2nd August 2012
Abstract
Ionic liquid crystals based on quinolinium and isoquinolinium salts were prepared by quaternization of quinoline and isoquinoline, respectively. In the first place the influence of the alkyl chain on the thermal behaviour was investigated. Two types of (iso)quinolinium salts were synthesized. Long-chained (iso)quinolinium cations (with chain lengths varying from C12H25 to C22H45), with the inclusion of the odd homologues C17H35 and C19H39, were combined with the spherical anions bromide, tetrafluoroborate (BF4−) and hexafluorophosphate (PF6−). Dodecyl sulfate, dioctyl-, dihexyl- and dicyclohexylsulfosuccinate anions allowed the introduction of anions with (long) alkyl chains. It is shown that a subtle balance between strong electrostatic forces and the degree of disorder originating from molten alkyl chains is crucial for mesophase formation. The influence of the anion size on the mesophase behaviour was studied. Smectic A phases were observed for the long chain analogues of the bromide, tetrafluoroborate and hexafluorophosphate salts. The minimum alkyl chain length needed to induce mesomorphism increases when the bromide is exchanged by more voluminous anions, while the transition temperatures are lowered. The introduction of an alkyl chain in the anionic part drastically changes the thermal behaviour. The dodecyl sulfate salts show smectic A phases for cations with much shorter chain lengths than the other anions. For the dioctylsulfosuccinate salts this trend is even more pronounced, as the N-methyl(iso)quinolinium dioctylsulfosuccinate salts exhibited enantiotropic smectic A phases at ambient temperature. Structural models for the packing of the quinolinium and isoquinolinium salts in the liquid-crystalline state are presented, as well as the crystal structures of N-octylquinolinium dodecyl sulfate and N-octylisoquinolinium bromide.
Introduction
Experimental data accumulated over the years give a good insight into the structure–property relationship of ionic liquids.1–4 One of the most important properties of ionic liquids is their melting point, because ionic liquids can only be used as solvents above their melting point. In contrast with other physicochemical properties, melting points are more difficult to accurately predict or calculate.5–11 Although the melting point can be predicted from the ratio of the calculated values of the melting enthalpy and entropy, differences between the calculated and experimental melting points are about ±25 °C, so that accurate experimental determination of the melting points of new ionic liquids is still of prime importance.12 Factors that influence the melting point are the nature of the anion and the cation, and the length of the alkyl chain. In general, the melting point first decreases with increasing chain length and will reach a minimum at a chain length of about four (butyl) to six (hexyl) carbon atoms.13,14 The reason for this decrease in melting point is that longer alkyl chains can adopt more different conformations and this hampers efficient packing of the molecules in a crystal lattice. However, the melting point starts to increase for longer alkyl chains, because the contribution of the van der Waals interactions between the alkyl chains becomes more important for the longer alkyl chains. Interestingly, for very long chains the ionic liquids start to exhibit liquid-crystalline behaviour. This means that upon heating, the crystalline solid first melts to give a liquid-crystalline phase (or mesophase). In the liquid-crystalline phase the molecular order is between that of the highly ordered crystalline solid phase and the disordered liquid phase. Liquid crystals are anisotropic fluids. Upon further heating, the molecular order in the liquid-crystalline phase is suddenly lost at the clearing point and one obtains an isotropic liquid. Typically, ionic liquids form layered liquid-crystalline phases (smectic phases). The most common mesophase for ionic liquid crystals is the smectic A phase. In this phase, no correlation exists between the molecules in the layers. The minimum chain length at which an ionic liquid forms a mesophase depends on the nature of the cation, but also on the nature of the anion. For the 1-alkylimidazolium salts [Cnmim]X, a mesophase is observed for [C12mim][BF4] and higher homologues,13 for [C14mim][PF6] and higher homologues,15 and for [C16mim][TfO] and higher homologues,16 but no liquid-crystalline behaviour has been reported for the [Cnmim][Tf2N] series16 (TfO = triflate, Tf2N = bis(trifluoromethylsulfonyl)imide). For the liquid-crystalline 1-alkyl-3-methylimidazolium salts, the temperature range of the mesophase widens with increasing alkyl chain length. However, two long chains do not have a stabilizing effect on mesophases.17,18 Sometimes, the dependence of the thermal behaviour on the alkyl chain length can be surprising, as in the case of the N-alkylpyridinium tetrachlorocuprate(II) salts.19 The compounds with C12H25, C13H27, and C14H29 chains exhibit a hexagonal columnar mesophase. The derivatives with C16H33, C17H35, and C18H37 chains form a smectic A phase. The compound with a C15H31 chain exhibits a cubic mesophase between the crystalline state and a (partially bilayered) smectic A phase.20 This cubic phase is only observed for the derivative with the pentadecyl chain and not for the derivatives with shorter or longer chains. Highly ordered smectic mesophases have been observed for quaternary ammonium,21,22 pyrrolidinium,23 piperidinium,24 piperazinium,24 morpholinium,24 and 1,10-phenanthrolinium ionic liquid crystals.25 For an overview of ionic liquid crystals, the reader is referred to the review literature.26–29
Most ionic liquids are based on imidazolium, pyridinium, pyrrolidinium, quaternary ammonium or phosphonium cations. The quinolinium and isoquinolinium ionic liquids have been investigated to a much lower extent, mainly because they are expected to have higher melting temperatures.30–40 Nevertheless, these ionic liquids could be of interest as the non-aqueous phase in solvent extraction systems for organic compounds.30,31 Although N-alkylquinolinium bromides,39N-alkylisoquinolinium hexafluorophosphates32 and N-alkylisoquinolinium bis(trifluoromethylsulfonyl)imides,32 with an alkyl chain up to octadecyl (C18H37), have been prepared, no liquid-crystalline phases have been reported for these compounds. Recently, Kato and co-workers described new luminescent liquid crystals based on highly substituted tripodal quinolinium salts.41
In this paper, we show that mesomorphism can be observed for N-alkylquinolinium and N-alkylisoquinolinium salts with bromide, tetrafluoroborate, dodecyl sulfate (DOS) and hexafluorophosphate anions, provided that the alkyl chains are long enough, and also that N-methyl(iso)quinolinium sulfosuccinate salts are liquid-crystalline. Quinolinium and isoquinolinium compounds with alkyl chains varying between C12H25 and C22H45 have been synthesized. For the long chain lengths, the odd homologues (C17H35, C19H39) have also been prepared. A structural model for the molecules in the smectic A phase (SmA) is presented. The crystal structures of N-octylquinolinium dodecyl sulfate and N-octylisoquinolinium bromide could be determined by single-crystal X-ray diffraction.
Experimental section
General
Information on the synthesis and characterization of all compounds can be found in the ESI.† Nuclear magnetic resonance (NMR) spectra were recorded on a Bruker Avance 300 spectrometer (operating at 300 MHz for 1H and at 75 MHz for 13C). Elemental analyses were obtained on a CE Instruments EA-1110 elemental analyzer. Defect textures of the mesophases were observed with an Olympus BX60 polarizing optical microscope equipped with a LINKAM THMS600 hot stage and a LINKAM TMS93 programmable temperature controller. DSC traces were recorded with a Mettler-Toledo DSC822e module (heating/cooling rate of 10 °C min−1; helium atmosphere). Powder X-ray diffractograms were recorded with a Bruker AXS D8 Discover diffractometer mounted with a copper X-ray ceramic tube, working at 1.6 kW. The emitted Cu-Kα radiation (λ = 1.5418 Å) was focused on the sample by a Göbel mirror. All the samples (without a thermal history) were prepared by spreading the compounds on a thin cleaned silicon wafer. Diffractograms were recorded using the Bragg–Brentano reflection geometry (θ/2θ setup) at an angular resolution (in 2θ) of 0.03° per step. The deviation between the temperature on the surface of the sample holder and the set temperature was about 3%. The scattering signal was recorded with a one-dimensional detector (LynxEye detector).
Crystallography
Crystals suitable for an X-ray diffraction study of N-octylquinolinium dodecyl sulfate Q8.c and N-octylisoquinolinium bromide IQ8.a could be obtained by slowly evaporating a solution of the compound in acetone and dichloromethane, respectively. X-ray intensity data were collected at RT on a Bruker SMART 6000 diffractometer equipped with a CCD detector using Cu-Kα radiation (λ = 1.54178 Å), using ϕ and ω scans. The images were interpreted and integrated with the program SAINT from Bruker.42 Both structures were solved by direct methods and refined by full-matrix least-squares on F2 using the SHELXTL program package.43,44 Non-hydrogen atoms were anisotropically refined and the hydrogen atoms in the riding mode and isotropic temperature factors fixed at 1.2 times Ueq of the parent atoms (1.5 times for methyl groups and water molecules). CCDC 837736 and 837737 can be found in the ESI.†
Crystal data for compound
Q8.c. C29H49NO4S, M = 507.76, triclinic, P
(No. 2), a = 8.0982(2), b = 8.6854(2), c = 23.0307(5) Å, α = 96.8330(10), β = 98.6960(10), γ = 104.9070(10)°, V = 1526.10(6) Å3, T = 293(2) K, Z = 2, ρcalc = 1.105 g cm−3, μ(Cu-Kα) = 1.179 mm−1, F(000) = 556, crystal size 0.3 × 0.3 × 0.1 mm, 5214 independent reflections (Rint = 0.0759). Final R = 0.0814 for 2936 reflections with I > 2σ(I) and wR2 = 0.1885 for all data. The N-octyl chain was found to be disordered and could be modelled in two parts (refined occupancies of 0.418(11) and 0.582(11)).
Crystal data for compound
IQ8.a. C17H25.26BrNO0.63, M = 333.59, monoclinic, P21/n (No. 14), a = 9.8423(5), b = 13.4447(7), c = 13.1983(7) Å, β = 101.539(2)°, V = 1711.19(15) Å3, T = 293(2) K, Z = 4, ρcalc = 1.295 g cm−3, μ(Cu-Kα) = 3.204 mm−1, F(000) = 697, crystal size 0.6 × 0.3 × 0.2 mm, 3027 independent reflections (Rint = 0.0469). Final R = 0.0537 for 2389 reflections with I > 2σ(I) and wR2 = 0.1380 for all data. The occupancy of the water solvent molecule was refined to 0.628(9).
Results and discussion
Synthesis and characterization
An overview of the quinolinium and isoquinolinium salts is given in Chart 1. The N-alkylquinolinium bromides Qn.a, the N-alkylisoquinolinium bromides IQn.a and the N-methyl(iso)quinolinium iodides Q1.e and IQ1.e were synthesized via a quaternization reaction (Menschutkin reaction) of the quinoline or isoquinoline base with the appropriate bromoalkane or iodomethane. The length of the alkyl chain was varied between four (C4H9) and twenty-two carbon atoms (C22H45). The tetrafluoroborate salts Qn.b and IQn.b were synthesized by means of a metathesis reaction between the bromides Qn.a and IQn.a and sodium tetrafluoroborate in acetone. The dodecyl sulfate (DOS) salts Qn.c and IQn.c were synthesized by the reaction of the bromide salt Qn.a and IQn.a and sodium dodecyl sulfate in water. The hexafluorophosphate salts Qn.d and IQn.d were synthesized via a metathesis reaction of the bromide salt Qn.a and IQn.a and potassium hexafluorophosphate in a methanol–water mixture (6
:
4). The N-alkyl(iso)quinolinium sulfosuccinates Q1.f–h, Q4.f, Q8.f and IQ.f–h were synthesized in a similar fashion to the DOS salts using the iodide or bromide salts as starting materials. The purity of the compounds was verified using 1H NMR and 13C NMR spectroscopy and CHN elemental analysis.
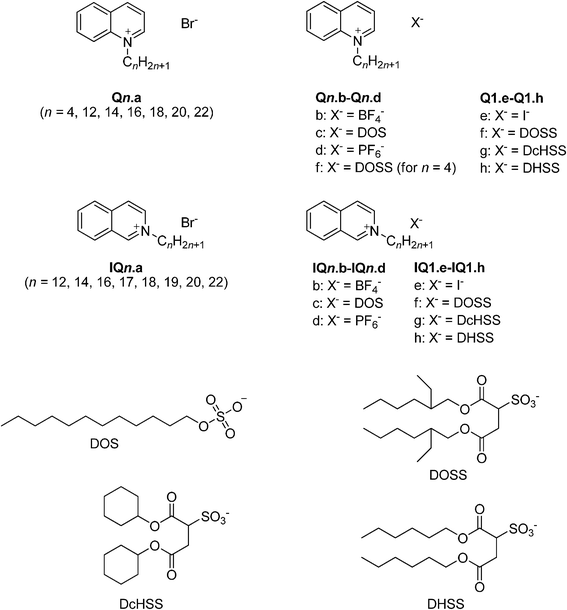 |
| Chart 1 Overview of the quinolinium and isoquinolinium compounds. | |
Single crystal X-ray diffraction
In order to study the crystal structure of the quinolinium and isoquinolinium salts, single crystals of two shorter-chain analogues were grown, namely of N-octylquinolinium dodecyl sulfate Q8.c and N-octylisoquinolinium bromide IQ8.a. Compound Q8.c crystallized into the centrosymmetric triclinic space group P
. The asymmetric unit consists of one N-octylquinolinium–dodecyl sulfate cation–anion pair (Fig. 1). The N-octyl chain substituent of the quinolinium cation shows a C1–N1–C10–C11 torsion angle of −97.6(4)°, orienting most of the staggered chain perpendicular to the quinolinium rings. The end propyl group is found disordered over two positions. Interactions between the dodecyl sulfate anion and the quinolinium cations are observed. Eight close contacts between the sulfate group and five different quinolinium cations are found (in the range 2.35–2.65 Å). In the packing, π–π ring interactions occur between the quinolinium rings. Ring C1–C5/N1 interacts with the symmetry equivalent ring C1–C5/N1 (1 − x, 1 − y, 2 − z) and with ring C4–C9 (1 − x, 1 − y, 2 − z) (centroid–centroid distances of 3.836(2) and 3.946(2) Å, respectively). Furthermore, layers in the (110) plane are formed by the closely packed hydrophobic alkyl chains of both cations and anions, with their hydrophilic parts pointing outwards. Compound IQ8.a crystallized into the centro-symmetric monoclinic space group P21/n. The asymmetric unit consists of one N-octylisoquinolinium–bromide cation–anion pair and one water molecule (Fig. 2). Although there was no water used in the synthesis, nor in the crystallization conditions, the observed water molecule is probably due to the highly hygroscopic character of the compound, resulting in binding of residual water molecules present in the solvents used for synthesis and crystallization. The bromide salts with longer alkyl chains are more hydrophobic and have a lower tendency to bind water molecules. The alkyl chain of compound IQ8.a shows a C1–N1–C10–C11 torsion angle of 78.0(4)°, with the C10–C11 bond almost perpendicular to the isoquinolinium rings, whereas the rest of the alkyl chain is found staggered and approximately in the same plane as the isoquinolinium rings. C–H⋯Br− interactions are observed between the N-octylisoquinolinium cation and the bromide anion. Four close contacts between the Br− anion and three different isoquinolinium cations are observed (in the range 2.86–2.99 Å). Hydrogen bonds are formed between the water molecule and two Br− anion symmetry equivalents (1/2 − x, −1/2 + y, 1/2 − z; −1/2 + x, 3/2 − y, −1/2 + z), related by an inversion centre (O(H)⋯Br− distances of 3.391(7) and 3.171(7) Å, respectively), creating a cyclic hydrogen bond network. No other structures containing the N-octylquinolinium or the N-octylisoquinolinium cation were found in the Cambridge Structural Database (CSD).45 However, other structures containing quinolinium cations with short linear N-alkyl chain substituents were found in the CSD: N-pentylquinolinium,46N-butylquinolinium,47–51N-propylquinolinium,46,52,53N-ethylquinolinium,54 and methylquinolinium.55–57 None of these structures contained a dodecyl sulfate anion. Also, structures containing isoquinolinium cations with linear N-alkyl chain substituents were found in the CSD: N-butylisoquinolinium,32,58N-propylisoquinolinium,59N-ethylisoquinolinium,32 and N-methylisoquinolinium.60 None of these structures contained a bromide anion.
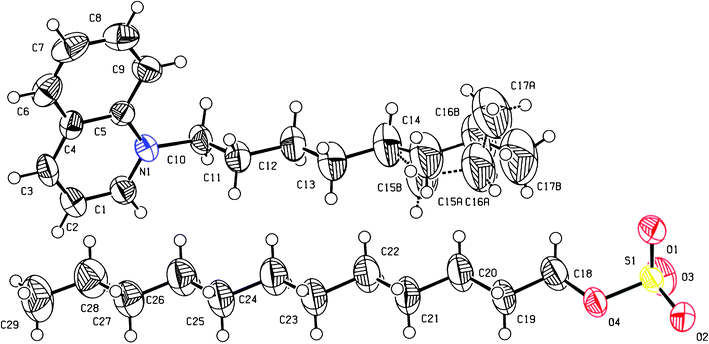 |
| Fig. 1 ORTEP representation of the molecular structure of compound Q8.c, with atom labelling scheme. Thermal ellipsoids are drawn at the 50% probability level. The disorder of the N-octyl chain is shown. | |
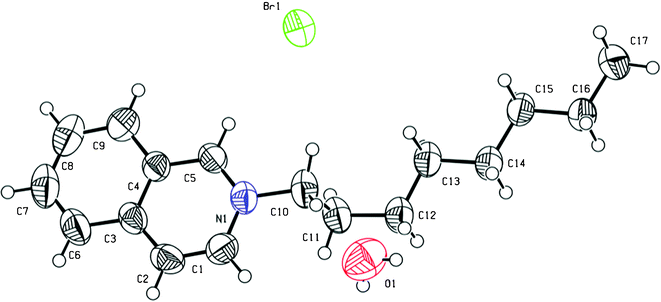 |
| Fig. 2 ORTEP representation of the molecular structure of compound IQ8.a, with atom labelling scheme. Thermal ellipsoids are drawn at the 50% probability level. | |
Thermal properties
The thermal properties of the quinolinium and isoquinolinium salts were investigated by polarizing optical microscopy (POM), differential scanning calorimetry (DSC), and also, for compounds Q18.a, IQ18.a, Q16.c and IQ16.c, by X-ray powder diffraction at elevated temperatures. In Fig. 3, the DSC traces of compounds IQ19.b and Q22.d are shown. Due to the complex thermal behaviour and the hygroscopicity of the quinolinium and isoquinolinium salts, the first and second heating runs were not identical for most compounds. The first heating run values were used if no melting peak was observed in the second heating run (the salts did not crystallize on cooling down to −20 °C) and if the second heating showed several unresolved endothermic and exothermic peaks (i.e. a sequence of recrystallization processes and crystal-to-crystal transitions) before melting. It must be noted that even though different crystalline conformations were adapted in the first and second heating run, the melting temperature was identical in both cases. Compounds that were heated above 200 °C decomposed slightly. This was observed in the DSC thermogram as a deviation from the baseline at the end of the first heating run and confirmed by POM. In general, the quinolinium and isoquinolinium salts showed up to three different crystalline phases. The occurrence of polymorphism in the solid state is not unusual for ionic liquids.61–66 The thermal behaviour of the quinolinium and isoquinolinium salts is a nice example of the subtle balance between volume effects, electrostatic interactions and separation between ionic and aliphatic parts of the molecules (microsegregation)67 that are required for the formation of liquid-crystalline phases.
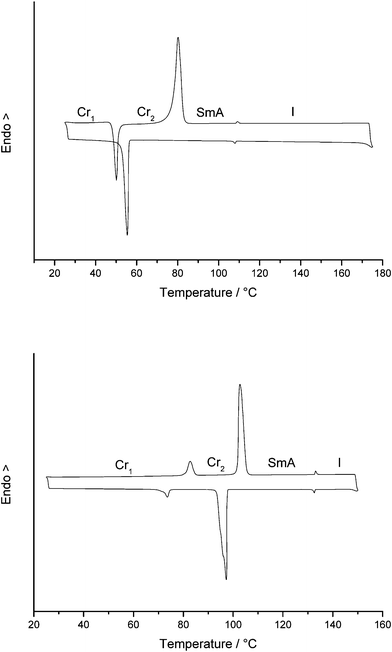 |
| Fig. 3 Top: DSC-trace of IQ19.b—second heating run. Bottom: DSC-trace of Q22.d—second heating run. | |
An overview of the mesophase behaviour of the liquid-crystalline compounds is shown in Fig. 4–7. Tables with the transition temperatures and enthalpy changes for all the compounds are given in the ESI (Tables S1 and S2).† The quinolinium bromides Qn.a with tetradecyl and shorter alkyl chain lengths were not liquid-crystalline (Fig. 4). It is not surprising that mesomorphism is observed only for the long chain analogues, since a minimum alkyl chain length of twelve carbon atoms is needed for imidazolium salts and pyridinium salts in order to obtain microphase separation.13–16,68 In addition, the ionic head group is extended in (iso)quinolinium salts compared to pyridinium, so that longer alkyl chains are required for the induction of a mesophase. This case is similar to that of the imidazolium and benzimidazolium salts.17 A monotropic smectic A phase was observed for bromides Q16.a and Q17.a. Compounds Q18.a–Q22.a showed a smectic A phase over a temperature range 58 to 98 °C. The melting point increases with increasing chain length in the series Q12.a–Q17.a. For compounds Q18.a–Q20.a, the melting point varies only slightly with the chain length, while the clearing point sharply increases. The mesophase range and transition temperatures of the bromide salt Q22.a are similar to those of Q20.a. For pyridinium ionic liquid crystals, the same tendency has been observed.68 At this point the ionic interactions can no longer efficiently compensate for the large volume that the molten alkyl chain occupies at high temperatures, and no further increase in mesophase stability is observed. Compounds Q17.a and Q19.a melt at slightly higher temperatures than their analogues with an even number of carbon atoms in the alkyl chain. Such odd–even effects have also been observed for imidazolium and pyridinium salts.13,68 It should be mentioned that the N-alkylquinolinium bromides with an alkyl chain up to an octadecyl chain have been described in the literature, but their liquid-crystalline behaviour seems to have been overlooked.39
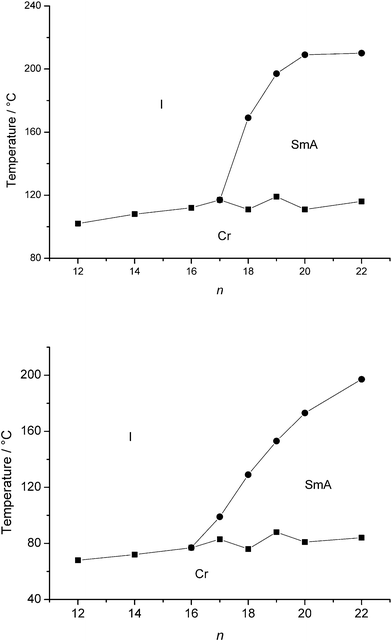 |
| Fig. 4 Schematic representation of the transition temperatures and mesophase behaviour of the quinolinium bromides Qn.a (top) and the isoquinolinium bromides IQn.a (bottom). | |
The thermal behaviour of the isoquinolinium bromides IQ12.a–IQ22.a resembles that of the corresponding quinolinium bromides (Fig. 4). A smectic A phase was observed for the bromides with a hexadecyl or longer alkyl chain (IQ16.a–IQ22.a). The mesophase temperature range increases with chain length, reaching a maximum of 113 °C for IQ22.a. In this series of isoquinolinium salts, the clearing point still rises significantly when going from a C20H41 to a C22H45 chain, in contrast to the quinolinium bromide salts. This is probably due to better packing of the aromatic cores (see the crystal model presented in Fig. S4 (ESI)†). It is expected that for chain lengths longer than twenty-two carbon atoms, the clearing temperatures and mesophase temperature range will at some point start to level off or to decrease. Unfortunately, 1-bromoalkanes with alkyl chains longer than C22H45 are not commercially available or easily accessible via synthetic procedures.
The thermal behaviour of the dodecyl sulfate salts, in which both cation and anion bear a long alkyl chain, is somewhat different from that of the other salts, in the sense that a smectic A phase was already observed for a chain length of twelve carbon atoms or longer (Fig. 5). This is not surprising as the anion is now contributing to the amphiphilicity of the molecule as well. The mesophase stabilities of the dodecyl sulfate salts are lower than those of the (iso)quinolinium bromide salts. Upon melting, the weak van der Waals interactions between these chains are broken and they no longer adapt an all-trans conformation, thus enlarging the aliphatic–ionic interphase area. In other words, the area imposed by the aliphatic chains, which increases with increasing temperature, will no longer fit the area occupied by the ionic head groups. The same reasoning can be applied for the sulfosuccinate salts, although for N-methyl-(iso)quinolinium dioctylsulfosuccinates Q1.f and IQ1.f, only the anion bears alkyl chains, responsible for the fluidity in the system. Both compounds showed thermotropic smectic A phases at room temperature. Elongation of the alkyl chain is not beneficial for mesophase formation, as N-butylquinolinium dioctylsulfosuccinate Q4.f is not liquid-crystalline. Also, the cyclic sulfosuccinate salts Q1.g and IQ1.g were not liquid-crystalline, which could be expected. The use of hexylsulfosuccinate as the anion seems to be less effective, since only a monotropic smectic A phase was observed for the quinolinium hexylsulfosuccinate Q1.h.
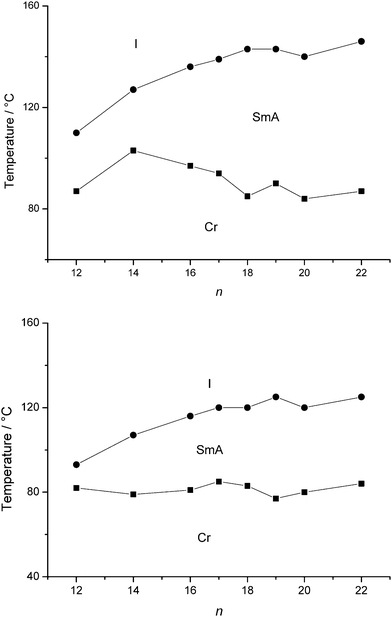 |
| Fig. 5 Schematic representation of the transition temperatures and mesophase behaviour of the quinolinium dodecyl sulfates Qn.c (top) and the isoquinolinium dodecyl sulfates IQn.c (bottom). | |
The type of anion has a major influence on the properties of ionic liquid crystals.26 In this paper, the bromide anion was exchanged with the less hydrophilic tetrafluoroborate, hexafluorophosphate anions. The earlier observations that longer alkyl chains are needed to accommodate the voluminous anions in the liquid-crystalline state are confirmed.13–16 Enantiotropic mesophases were observed for quinolinium tetrafluoroborates Q19.b–Q22.b and isoquinolinium tetrafluoroborates IQ18.b–IQ22.b (Fig. 6). Incorporation of the hexafluorophosphate anion requires a chain length of at least twenty carbon atoms to obtain mesomorphic (iso)quinolinium salts (Qn.d and IQn.d) (Fig. 7). In general, the clearing temperatures and mesophase temperature ranges decrease in the order: Br− > BF4− > PF6−. All mesomorphic tetrafluoroborate and hexafluorophosphate salts showed a smectic A phase.
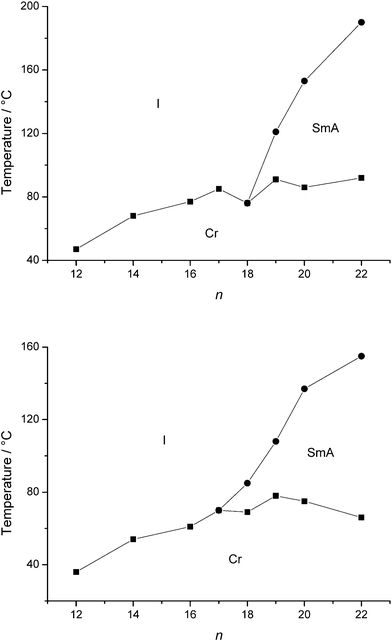 |
| Fig. 6 Schematic representation of the transition temperatures and mesophase behaviour of the quinolinium tetrafluoroborates Qn.b (top) and the isoquinolinium tetrafluoroborates IQn.b (bottom). | |
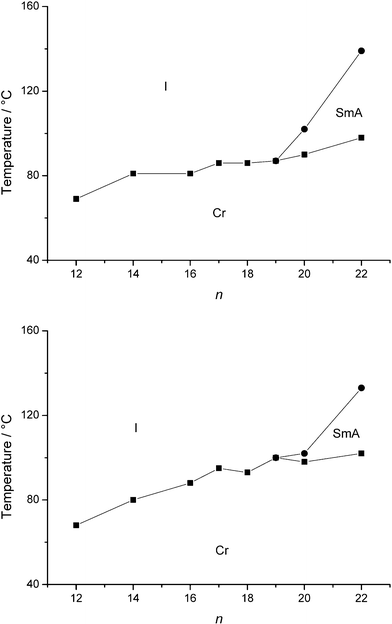 |
| Fig. 7 Schematic representation of the transition temperatures and mesophase behaviour of the quinolinium hexafluorophosphates Qn.d (top) and the isoquinolinium hexafluorophosphates IQn.d (bottom). | |
The smectic A phases were identified on the basis of their optical defect textures, observed by polarizing optical microscopy (POM). Upon cooling from the isotropic liquid, bâtonnets were formed. These bâtonnets coalesced into fans, giving rise to a fan texture (Fig. 8).
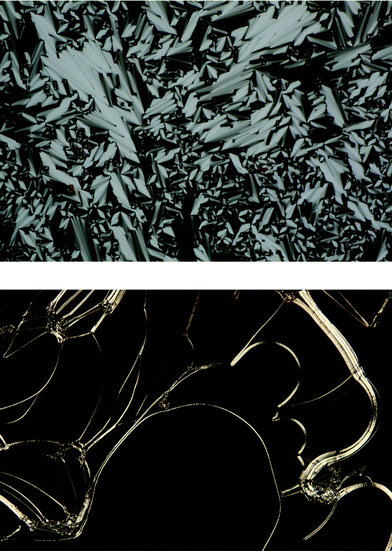 |
| Fig. 8 Mesophase defect textures observed by polarizing optical microscopy (POM). Top: fan texture of compound Q16.c at 132 °C. Bottom: oily streak texture of compound Q19.a at 166 °C. | |
Also, an oily streak texture with pseudo-isotropic domains was observed.
Powder X-ray diffraction (PXRD) and structural models
To obtain a better insight into the ordering of molecules within the smectic A phase, X-ray diffractograms were recorded for compounds Q18.a, IQ18.a, Q16.c and IQ16.c. A typical diffractogram is shown in Fig. 9. An overview of the Bragg reflections observed for these compounds is given in Table 1. In the small-angle region, one sharp reflection was detected for the smectic A phases of the quinolinium salts Q18.a and Q16.c, whereas two equidistant reflections were observed for the isoquinolinium salts IQ18.a and IQ16.c. The fact that only one reflection was observed for compounds that clearly form a smectic A phase is an indication that the smectic layers are ill defined. It is not uncommon for smectic A phases that the second-order reflection has an intensity that is only 1% of that of the first-order reflection.69,70 In that case, the second-order reflection often disappears in the background noise. Very weak or missing second-order reflections have also been observed for other types of smectogenic ionic liquid crystals.71,72,16 The reflections in the small-angle region are related to the thickness d of the smectic layers. A broad, diffuse peak was observed in the wide-angle region, situated around 4.5 Å for the bromide salts (Q18.a and IQ18.a) and around 4.6 Å for the dodecyl sulfate salts (Q16.c and IQ16.c). This signal is related to the short-range order of the molten alkyl chains. The information obtained from the X-ray diffractograms was used to calculate the molecular volume VM and the molecular area AM. The molecular volume was estimated using the relation VM = (M/0.6022)f, where M is the molecular mass (g mol−1) and f is a temperature correcting factor (f = 0.9813 + 7.474 × 10−4T, with T the temperature in °C).73 The molecular area AM was calculated using the equation AM = 2VM/d, where d is the smectic layer thickness. As expected, the calculated molecular area is much smaller for the bromide salts (AM ≈ 48 Å2) than for the dodecyl sulfate salts (AM ≈ 72 Å2), because of the large sulfate head groups. From the crystal structures, it can be derived that the aromatic core of the quinolinium salts is oriented almost perpendicularly to the staggered alkyl chain, while the isoquinolinium core lies in the same plane as the major part of the alkyl chain. As a consequence, the molecular area of the quinolinium salts is somewhat larger than the molecular area of the isoquinolinium salts. In Fig. 10 a structural model is proposed for the quinolinium bromides.
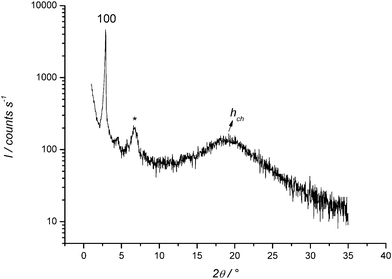 |
| Fig. 9 X-ray diffractogram of the smectic A phase of N-hexadecylisoquinolinium dodecyl sulfate IQ16.c at 90 °C, represented as the logarithm of the scattering intensity (in counts) versus the scattering angle 2θ (Cu-Kα radiation). The broad diffraction signal centred at 2θ ≈ 6.5° is due to the covering foil used in the experimental setup (marked with *). | |
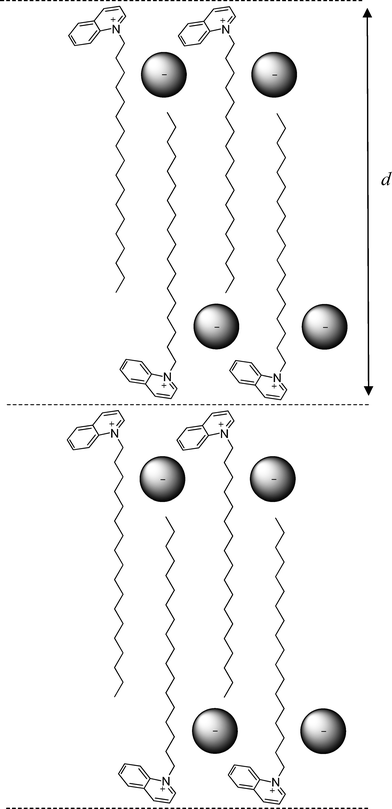 |
| Fig. 10 Structural model for the smectic A phase of compound Q18.a. The smectic layer thickness is indicated by d (at 120 °C, d = 32.62 Å, and AM ≈ 50.4 Å2). | |
Table 1 Bragg reflections collected from the X-ray diffractograms of the enantiotropic mesophases (Cu-Kα radiation)
Compd. |
d
meas/Åa |
I b |
hkl
|
d
calc/Åa |
Parameters of the mesophase at temperature Tdef |
d
meas and dcalc are the measured and calculated diffraction spacings, respectively.
I is the intensity of the reflections: S, strong; M, medium; W, weak; br, broad reflection.
hkl are the Miller indices of the reflections and hch is the periodicity corresponding to the liquid-like order of the molten aliphatic chains.
T is the temperature at which the X-ray diffractogram is recorded.
V
M is the molecular volume and AM the molecular area.
Abbreviation: SmA = smectic A phase.
|
Q18.a
|
32.62 |
S |
001 |
32.62 |
SmA: T = 120 °C |
|
4.53 |
br |
h
ch
|
|
V
M = 823 Å3 |
|
|
|
|
|
A
M = 50.4 Å2 |
IQ18.a
|
34.50 |
S |
001 |
34.60 |
SmA: T = 111 °C |
|
17.35 |
M |
002 |
17.30 |
V
M = 817 Å3 |
|
4.51 |
br |
h
ch
|
|
A
M = 47.3 Å2 |
Q16.c
|
29.14 |
S |
001 |
29.14 |
SmA: T = 111 °C |
|
4.68 |
br |
h
ch
|
|
V
M = 1096 Å3 |
|
|
|
|
|
A
M = 75.2 Å2 |
IQ16.c
|
30.63 |
S |
001 |
30.78 |
SmA: T = 90 °C |
|
15.47 |
W |
002 |
15.39 |
V
M = 1080 Å3 |
|
4.67 |
br |
h
ch
|
|
A
M = 70.1 Å2 |
Conclusions
Thermotropic liquid crystals are composed of molecules that adopt an anisometric shape, most commonly rod-like or disk-like molecules. At present it is still difficult to predict which type of phase is formed, since several factors have to be taken into account. In this study, we tried to provide a better insight into the driving forces for mesophase formation of (iso)quinolinium salts. Although N-alkylquinolinium and N-alkylisoquinolinium salts with bromide, tetrafluoroborate, hexafluorophosphate, dodecyl sulfate anions and sulfosuccinate exhibit smectic A phases as many other ionic liquid crystals,26 the thermal behaviour of these ionic liquid crystals is atypical, in the sense that these compounds only exhibit liquid-crystallinity when their alkyl chain is very long. It had been observed for 1-alkyl-3-imidazolium salts that the temperature range of the mesophase widens with increasing alkyl chain length,13 but it is uncommon that mesophases are formed only in the case of very long chains. After all, the long-chain compounds have relatively large aliphatic parts compared to the ionic parts, while the ionic part is responsible for the formation of the layer structure and thus for the occurrence of the smectic mesophases. It is not true that just increasing the alkyl chain length leads to more stable mesophases in the case of ionic liquid crystals, as illustrated by the disappearance of liquid-crystallinity in the case of the lanthanide(III) alkanoates with very long alkyl chains.74,75 There exist only very few studies on liquid crystals with alkyl chains longer than C20H41,76,77 because the required long-chain 1-bromoalkanes are very expensive or even not commercially available. This study shows that liquid-crystalline behaviour can be observed for ionic liquids with very long alkyl chains as well as for their short-chained analogues, dependent on the choice of anion. The key factor for mesophase formation in these ionic liquid crystals is a delicate equilibrium between microphase segregation and the relative size of areas occupied by incompatible units (aliphatic/ionic) at the interface, rather than the number of carbon atoms present in the alkyl chain.
Acknowledgements
KL is a Research Assistant of the IWT Flanders. Financial support by the KU Leuven (project GOA 08/05) is gratefully acknowledged. CHN elemental analyses were performed by Dirk Henot. Powder X-ray diffractograms were recorded by Dr Jan D'Haen and Bart Ruttens (University of Hasselt, Institute for Materials Research).
References
- T. Welton, Chem. Rev., 1999, 99, 2071–2083 CrossRef CAS.
- T. Wasserscheid and W. Keim, Angew. Chem., Int. Ed., 2000, 39, 3773–3789 CrossRef.
- N. V. Plechkova and K. R. Seddon, Chem. Soc. Rev., 2008, 37, 123–150 RSC.
-
P. Wasserscheid and T. Welton (ed.), Ionic Liquids in Synthesis, 2002, Wiley-VCH, Weinheim Search PubMed.
- M. Deetlefs, K. R. Seddon and M. Shara, Phys. Chem. Chem. Phys., 2006, 8, 642–649 RSC.
- L. Glasser and H. D. B. Jenkins, Chem. Soc. Rev., 2005, 34, 866–874 RSC.
- A. R. Katritzky, A. Lomaka, R. Petrukhin, R. Jain, M. Karelson, A. E. Visser and R. D. Rogers, J. Chem. Inf. Model., 2002, 42, 71–74 CrossRef CAS.
- I. Billard, G. Marcou, A. Ouadi and A. Varnek, J. Phys. Chem. B, 2011, 115, 93–98 CrossRef CAS.
- P. Eiden, S. Bulut, T. Kochner, C. Friedrich, T. Schubert and I. Krossing, J. Phys. Chem. B, 2011, 115, 300–309 CrossRef CAS.
- U. Preiss, V. N. Emel'yanenko, S. P. Verevkin, D. Himmel, Y. U. Paulechka and I. Krossing, ChemPhysChem, 2010, 11, 3425–3431 CrossRef CAS.
- C. Q. Yan, M. J. Han, H. Wan and G. F. Guan, Fluid Phase Equilib., 2010, 292, 104–109 CrossRef CAS.
- U. Preiss, S. Bulut and I. Krossing, J. Phys. Chem. B, 2010, 114, 11133–11140 CrossRef CAS.
- J. D. Holbrey and K. R. Seddon, J. Chem. Soc., Dalton Trans., 1999, 2133–2139 RSC.
- J. G. Huddleston, A. E. Visser, W. M. Reichert, H. D. Willauer, G. A. Broker and R. D. Rogers, Green Chem., 2001, 3, 156–164 RSC.
- C. M. Gordon, J. D. Holbrey, A. R. Kennedy and K. R. Seddon, J. Mater. Chem., 1998, 8, 2627–2636 RSC.
- A. E. Bradley, C. Hardacre, J. D. Holbrey, S. Johnston, S. E. J. McMath and M. Nieuwenhuyzen, Chem. Mater., 2002, 14, 629–635 CrossRef CAS.
- K. M. Lee, C. K. Lee and I. J. B. Lin, Chem. Commun., 1997, 899–900 RSC.
- X. J. Wang, F. W. Heinemann, M. Yang, B. U. Melcher, M. Fekete, A. V. Mudring, P. Wasserscheid and K. Meyer, Chem. Commun., 2009, 7405–7407 RSC.
- F. Neve, O. Francescangeli, A. Crispini and J. Charmant, Chem. Mater., 2001, 13, 2032–2041 CrossRef CAS.
- F. Neve and M. Imperor-Clerc, Liq. Cryst., 2004, 31, 907–912 CrossRef CAS.
- J. Przedmojski and P. Dynarowicz-Latka, Phase Transitions, 1999, 70, 133–146 CrossRef CAS.
- E. Alami, H. Levy, R. Zana, P. Weber and A. Skoulios, Liq. Cryst., 1993, 13, 201–212 CrossRef CAS.
- K. Goossens, K. Lava, P. Nockemann, K. Van Hecke, L. Van Meervelt, K. Driesen, C. Gorller-Walrand, K. Binnemans and T. Cardinaels, Chem.–Eur. J., 2009, 15, 656–674 CrossRef CAS.
- K. Lava, K. Binnemans and T. Cardinaels, J. Phys. Chem. B, 2009, 113, 9506–9511 CrossRef CAS.
- T. Cardinaels, K. Lava, K. Goossens, S. V. Eliseeva and K. Binnemans, Langmuir, 2011, 27, 2036–2043 CrossRef CAS.
- K. Binnemans, Chem. Rev., 2005, 105, 4148–4204 CrossRef CAS.
- T. Kato, N. Mizoshita and K. Kishimoto, Angew. Chem., Int. Ed., 2006, 45, 38–68 CrossRef CAS.
- I. J. B. Lin and C. S. Vasam, J. Organomet. Chem., 2005, 690, 3498–3512 CrossRef CAS.
- K. V. Axenov and S. Laschat, Materials, 2011, 4, 206–259 CrossRef CAS.
- J. D. Holbrey, I. Lopez-Martin, G. Rothenberg, K. R. Seddon, G. Silvero and X. Zheng, Green Chem., 2008, 10, 87–92 RSC.
- A. E. Visser, J. D. Holbrey and R. D. Rogers, Chem. Commun., 2001, 2484–2485 RSC.
- A. E. Visser, J. G. Huddleston, J. D. Holbrey, W. M. Reichert, R.P. Swatloski and R. D. Rogers, ACS Symp. Ser., 2007, 975, 362–380 CrossRef CAS.
- C. M. Lee, J. Korean Chem. Soc., 1972, 16, 205–207 CAS.
- U. Domanska, M. Zawadzki, M.M. Tshibangu, D. Ramjugernath and T. M. Letcher, J. Chem. Thermodyn., 2010, 42, 1180–1186 CrossRef CAS.
- U. Domanska, M. Zawadzki, M. Krolikowska, M.M. Tshibangu, D. Ramjugernath and T. M. Letcher, J. Chem. Thermodyn., 2011, 43, 499–504 CrossRef CAS.
- U. Domanska, M. Zawadzki and M. Zwolinska, J. Chem. Thermodyn., 2011, 43, 775–781 CrossRef CAS.
- U. Domanska and M. Zawadzki, J. Chem. Thermodyn., 2012, 48, 276–283 CrossRef.
- U. Domanska, M. Zawadzki, M.M. Tshibangu, D. Ramjugernath and T. M. Letcher, J. Phys. Chem. B, 2011, 115, 4003–4010 CrossRef CAS.
- A. Busetti, D. E. Crawford, M. J. Earle, M. A. Gilea, B. F. Gilmore, S. P. Gorman, G. Laverty, A. F. Lowry, M. McLaughlin and K. R. Seddon, Green Chem., 2010, 12, 420–425 RSC.
- M. Zawadzki and U. Domanska, J. Chem. Thermodyn., 2011, 43, 989–995 CrossRef.
- K. Tanabe, Y. Suzui, M. Hasegawa and T. Kato, J. Am. Chem. Soc., 2012, 134, 5652–5661 CrossRef CAS.
-
SAINT, Manual Version 5/6.0, Bruker Analytical X-ray Systems Inc.: Madison, Wisconsin, 1997 Search PubMed.
-
SHELXTL-NT, Manual Version 5.1, Bruker Analytical X-ray Systems Inc.: Madison, Wisconsin, 1997 Search PubMed.
- G. M. Sheldrick, Acta Crystallogr., Sect. A: Found. Crystallogr., 2008, 64, 112–122 CrossRef.
- F. H. Allen, Acta Crystallogr., Sect. B: Struct. Sci., 2002, 58, 380–388 CrossRef.
- H. H. Li, Z. R. Chen, L. C. Cheng, M. Feng, H. D. Zheng and J. Q. Li, Dalton Trans., 2009, 4888–4895 RSC.
- G. Menczel, J. Kurti and R. Lehocz, Acta Chim. Hung., 1984, 116, 335–344 CAS.
- C. M. Zhong, Y.J. Zuo, H. S. Jin, S. Q. Liu and T. C. Wang, Acta Crystallogr., Sect. E: Struct. Rep. Online, 2006, 62, o5050–5051 Search PubMed.
- H. H. Li, Z. R. Chen, Y. Liu, K. N. Ding, J. Q. Li, C. C. Huang and L. Q. Guo, J. Cluster Sci., 2007, 18, 817–829 CrossRef CAS.
- H. H. Li, Z. R. Chen, L. Q. Guo, K. N. Ding, J. Q. Li, C. C. Huang and Z. L. Cai, Aust. J. Chem., 2007, 60, 595–602 CrossRef CAS.
- Y. J. Zuo, C. M. Zhong, H. S. Jin, Z.H. Su and T. C. Wang, Acta Crystallogr., Sect. E: Struct. Rep. Online, 2006, 62, o3561–o3562 Search PubMed.
- G. Rindorf, N. Thorup and K. Kamaras, Synth. Met., 1988, 25, 189–195 CrossRef CAS.
- Y. J. Wang, H. H. Li, C. C. Chen and J. B. Liu, Acta Crystallogr., Sect. E: Struct. Rep. Online, 2007, 63, m2736–u963 Search PubMed.
- M. Sokolov, A. Virovets, V. Nadolinnyi, K. Hegetschweiler, V. Fedin, N. Podherezskaya and V. Fedorov, Inorg. Chem., 1994, 33, 3503–3509 CrossRef CAS.
- T. M. Bockman and J. K. Kochi, J. Am. Chem. Soc., 1989, 111, 4669–4683 CrossRef CAS.
- J. P. Cornelissen, E. J. Creyghton, R. A. G. de Graaff, J. G. Haasnoot and J. Reedijk, Inorg. Chim. Acta, 1991, 185, 97–102 CrossRef CAS.
- J. L. Zuo, R. G. Xiong, X. Z. You and X. Y. Huang, Acta Crystallogr., Sect. C: Cryst. Struct. Commun., 1996, 52, 46–48 CrossRef.
- H. Xue, Z. F. Tong, F. Y. Wei and S. G. Qing, C. R. Chim., 2008, 11, 90–94 CrossRef CAS.
- E. O. Lykova, M. S. Chernov'yants, O. N. Kazheva, A. N. Chekhlov and O. A. D'yachenko, Russian J. Phys. Chem., 2004, 78, 1785–1789 Search PubMed.
- A. S. Kumbhar, S. B. Padhye, A. A. Kelkar, R. P. Patil, R.V. Chaudhari, V. G. Puranik, N. N. Dhaneshwar and S.S. Tavale, Polyhedron, 1996, 15, 1931–1935 CrossRef CAS.
- D. R. MacFarlane, P. Meakin, J. Sun, N. Amini and M. Forsyth, J. Phys. Chem. B, 1999, 103, 4164–4170 CrossRef CAS.
- J. Golding, S. Forsyth, D. R. MacFarlane, M. Forsyth and G. B. Deacon, Green Chem., 2002, 4, 223–229 RSC.
- D. R. MacFarlane, S. A. Forsyth, J. Golding and G. B. Deacon, Green Chem., 2002, 4, 444–448 RSC.
- A. Triolo, A. Mandanici, O. Russina, V. Rodriguez-Mora, M. Cutroni, C. Hardacre, M. Nieuwenhuyzen, H. J. Bleif, L. Keller and M. A. Ramos, J. Phys. Chem. B, 2006, 110, 21357–21364 CrossRef CAS.
- J. D. Holbrey, R. M. Reichert, M. Nieuwenhuyzen, S. Johnston, K. R. Seddon and R. D. Rogers, Chem. Commun., 2003, 1636–1637 RSC.
- A. V. Mudring, Aust. J. Chem., 2010, 63, 544–564 CrossRef CAS.
- C. Tschierske, Curr. Opin. Colloid Interface Sci., 2002, 7, 355–370 CrossRef CAS.
- G. A. Knight and B. D. Shaw, J. Chem. Soc., 1938, 682–683 RSC.
- J. Stamatoff, P. E. Cladis, D. Guillon, M. C. Cross, T. Bilash and P. Finn, Phys. Rev. Lett., 1980, 44, 1509–1512 CrossRef CAS.
- W.R. Krigbaum, J. C. Poirier and M. J. Costello, Mol. Cryst. Liq. Cryst., 1973, 20, 133–163 CrossRef CAS.
- P. H. J. Kouwer and T. M. Swager, J. Am. Chem. Soc., 2007, 129, 14042–14052 CrossRef CAS.
- J. M. Suisse, S. Bellemin-Laponnaz, L. Douce, A. Maisse-Francois and R. Welter, Tetrahedron Lett., 2005, 46, 4303–4305 CrossRef CAS.
- K. Goossens, P. Nockemann, K. Driesen, B. Goderis, C. Görller-Walrand, K. Van Hecke, L. Van Meervelt, E. Pouzet, K. Binnemans and T. Cardinaels, Chem. Mater., 2008, 20, 157–168 CrossRef CAS.
- K. Binnemans and C. Görller-Walrand, Chem. Rev., 2002, 102, 2303–2345 CrossRef CAS.
- K. Binnemans, L. Jongen, C. Bromant, D. Hinz and G. Meyer, Inorg. Chem., 2000, 39, 5938–5945 CrossRef CAS.
- K. Binnemans, R. Van Deun, B. Thijs, I. Vanwelkenhuysen and I. Geuens, Chem. Mater., 2004, 16, 2021–2027 CrossRef CAS.
- Y. Wang, N. Archambault, A.M. Belcher, D. Busse, D.B. Damon, A. Mills, A.E. Riddle, I.J. Samardjiev, B.L. Lucht and W.B. Euler, Macromolecules, 2008, 41, 7115–7121 CrossRef CAS.
Footnote |
† Electronic supplementary information (ESI) available: Synthetic procedures and characterization of all the compounds; table with transition temperatures and mesophase behaviour of the liquid-crystalline (iso)quinolinium salts; table with melting points of the non-mesomorphic compounds; figures showing the packing of the molecules in the crystal structures; structural model for the quinolinium dodecyl sulfates. CCDC reference numbers 837736 and 837737. For ESI and crystallographic data in CIF or other electronic format see DOI: 10.1039/c2ra21208k |
|
This journal is © The Royal Society of Chemistry 2012 |