DOI:
10.1039/C2RA21138F
(Paper)
RSC Adv., 2012,
2, 12804-12811
Novel fluorinated polybenzoxazine–silica films: chemical synthesis and superhydrophobicity
Received
7th June 2012
, Accepted 1st October 2012
First published on 2nd October 2012
Abstract
In this study, a novel fluorinated polybenzoxazine (PBZ) is successfully designed for the first time to fabricate superhydrophobic films on a glass surface. 2,2-Bis(3-fluorophenyl-3,4-dihydro-2H-1,3-benzoxazinyl)hexafluoro propane (BAF-fa) is successfully synthesized utilizing a conventional one-pot Mannich reaction. The transformation of the polymeric film of BAF-fa from hydrophobic to superhydrophobic has been achieved with silica modification, thus showing the involvement of the phenomena of low surface free energy and surface roughness combined. The method to fabricate uniform hybrid films resulted in a water contact angle (WCA) of 163°, and possesses the advantages of being straightforward and inexpensive. Morphological studies have revealed the induced hierarchical roughness of the hybrid films over multiple scales. The as-prepared hybrid films are superhydrophobic not only for neutral water, but also for water with a wide pH range. Additionally, these hybrid superhydrophobic films have shown promising durability in tap water and ethanol at different temperatures. This finding provides an effortless method to fabricate sliding superhydrophobic surfaces with high durability, thus expanding the role of PBZ in super water-repellency.
Introduction
The scope of studies of the imitation of super-water repellent surfaces has expanded in the past decade in different phases, starting from self-cleaning surfaces and leading to contamination prevention, anti-fouling and biotechnical applications.1–4 Superhydrophobic materials found in nature rely on Cassie's law and are biphasic on the submicrometer level. Both chemical properties and topographical micro structures contribute to the superhydrophobicity of a material. Thus, for a surface that is micro textured with the amalgamation of low surface free energy materials, the contact angle of water could exceed 160°. To imitate the superhydrophobicity found in nature, the available fabrication methods are inorganic particle deposition,5 sol–gel techniques using alkyl triethoxysilanes,6 plasma treatment of perfluorooctyl acrylates,7 vapour deposition,5 electrospinning to get polymeric and inorganic nanofibers,8–13 and casting techniques.14 Recent opportunities for high impact research lie mainly in elementary research and practical manufacturing.15–17
In the past few years, polybenzoxazine (PBZ), a new type of addition-cure phenolic system, has attracted great interest from both academia and industry.18 The diversity of the phenol and amine parts permits the design of monomers resulting in flexibility with modifiable PBZ properties. During the curing of the benzoxazine monomer to 150 °C, the C–O bonds of the benzoxazine rings cleave heterogeneously to form carbocations19 and phenoxide anions,20 which become the polymer units.21 The pseudo-cyclic structure based on stable intramolecular [–O−⋯HN+–] ionic interactions results in the possibility of helical structure formation in long-chain benzoxazine oligomers.22 The hydrophobic surfaces of PBZ can also be ascribed to this distinctive intramolecular interaction, which persuades the hydrophobic methyl groups to be positioned outside of the helical microstructures,23 thus indicating that PBZ is a strong candidate similar to the advanced materials used in hydrophobic coatings.
After preliminary studies on PBZ, in 2006, 2,2-bis(3,4-dihydro-3-methyl-2H-1,3-benzoxazine) propane (BA-m) and 2,2-bis(3-phenyl-3,4-dihydro-2H-1,3-benzoxazinyl) propane (BA-a) were reported as low surface energy materials.24 BA-m was used again after being treated with Ar and CF4 plasma and after SiO2 modification.25,26 Later, poly(vinyl phenol) was mixed with PBZ to minimize the amount of intermolecular hydrogen-bonding needed to decrease the surface free energy.27 The conversion of films of bis(3-allyl-3,4-dihydro-2H-1,3-benzoxazinyl)isopropane (B-ala) from hydrophobic to hydrophilic by UV-exposure has also been reported.28,29 Poly(siloxane-fluoroacrylate)-grafted SiO2 nanoparticles with superhydrophobicity synthesised via a two-step surface-initiated atom transfer radical polymerization were also reported.30,31 Recently, trimethoxysilane containing benzoxazine with m-trifluoromethyl groups was investigated as a low surface energy material.32 In most studies, the role of PBZ in the field of super water-repellency has been observed preliminarily. Therefore, an extensive series of experiments still needs to be undertaken to explore new PBZs with enhanced stability and durability for superhydrophobic applications.
The use of PBZ instead of fluoropolymers as low surface energy materials is at the centre of attention, because the former are cheaper to synthesize and simple to process. In this study, a novel fluorinated benzoxazine 2,2-bis(3-fluorophenyl-3,4-dihydro-2H-1,3-benzoxazinyl)hexafluoro propane (BAF-fa) monomer was synthesized using fluorinated bisphenol A and p-fluoroaniline. Finally, and just as importantly, both phenomena were incorporated into an organic–inorganic hybrid film that was made by adding SiO2 nanoparticles with high roughness to the low surface energy fluorinated benzoxazine. To our best knowledge, this method is the simplest one reported to fabricate superhydrophobic surfaces with enhanced durability in aqueous and organic media.
Experimental
Starting materials
4,4′-(Hexafluoroisopropylidene) bisphenol (BAF, 98%), 4-fluoroaniline, paraformaldehyde, anhydrous calcium chloride and SiO2 nanoparticles (diameter 7–40 nm) were supplied by Aladdin Chemistry Co. Ltd. Hydrochloric acid, sodium hydroxide, tricholoromethane, acetone and ethyl alcohol were supplied by Shanghai Chemical Reagents Co. Ltd. China. Glass slides (25.4 × 76.2 × 1 mm) used as coating substrates were supplied by Shanghai Machinery Imp. & Exp. Corporation). Milli-Q water with a resistance of 18.2 MΩ was obtained from a Millipore system. All chemicals were of analytical grade and were used as supplied.
Chemical Synthesis of (BAF-fa)
The BAF-fa monomer was synthesized using BAF, paraformaldehyde and 4-fluoroaniline through a one step Mannich reaction.33 As per usual, 15.28 g of BAF, 5.46 g of paraformaldehyde and 10.10 g of 4-fluoroaniline were added into a three necked round bottomed flask. The raw chemicals were stirred at room temperature for 30 min. Then the temperature was gradually increased to 100 °C with continued stirring for 2 h in an inert atmosphere. After cooling to room temperature, the obtained yellow product was dissolved in 75 mL of tricholoromethane. The monomeric (BAF-fa) solution was purified by washing three times with 2 wt% aqueous sodium hydroxide and three times with MilliQ water. The solution was treated with anhydrous calcium chloride and then filtered, and dried using rotary evaporation at 60 °C for 4 h to obtain BAF-fa powder. A schematic of the chemical reaction is shown in Scheme 1.
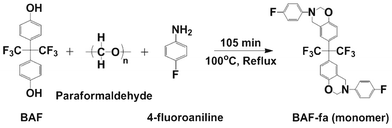 |
| Scheme 1 Synthetic route to BAF-fa. | |
Preparation of BAF-fa/SiO2 hybrid films
For the deposition of pure BAF-fa films, 3 wt% solution of the monomer in acetone was prepared at room temperature. The 3 mL monomer solution was spray coated on a glass slide. Similarly, hybrid films containing 3 wt% of BAF-fa and different concentrations of SiO2 nanoparticles (0.1, 0.5, 1, and 3 wt%) were spray coated on the glass surface (Scheme 2). Before spray coating, the solutions were kept in an ultrasound bath for 2 h. Pure and hybrid spray coated films were first dried at 60 °C in a vacuum oven for 20 min to complete the solvent evaporation, and then cured at 220 °C for 1 h to obtain a glass surface coated by pure and hybrid poly(2,2-bis(3-fluorophenyl-3,4-dihydro-2H-1,3-benzoxazinyl) hexafluoro propane) (P(BAF-fa)) with silica modification.
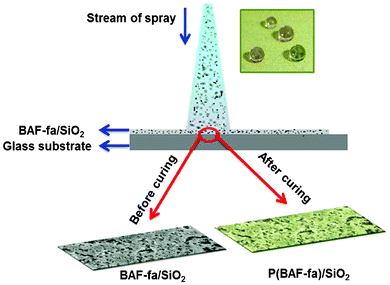 |
| Scheme 2 Pictorial presentation of the spray coating of the glass surface. The inset shows water droplets on hybrid films with perfect spherical shapes. | |
Characterization
The evidence from 1H, 13C and 19F nuclear magnetic resonance (NMR) spectra was obtained using a Bruker Avance 400 and deuterated tricholoromethane was used as the solvent. Fourier transform infra-red (FT-IR) spectra were recorded with a Nicolet 8700 FT-IR spectrometer in the range of 4000–400 cm−1. Differential scanning calorimetry (DSC) was carried out at a heating rate of 5 °C min−1 under a nitrogen atmosphere using an Auto Q20 Differential Scanning Calorimeter, TA Instruments, USA. Static contact angles and water contact angle hysteresis (WCAH) were measured using a Kino SL200B contact angle goniometer. The top morphology was examined by field emission-scanning electron microscopy (FE-SEM) using an S-4800, Hitachi Ltd., Japan. Atomic force microscopic (AFM) images were taken with a scan size of 10 μm × 10 μm using the tapping mode of AFM (Nanoscope IV, Digital Instruments). The transmittance responses were measured using an Ideaoptics FX4000+fiber optic spectrometer (Ideaoptics Technology Ltd., China).
Durability measurements
To investigate the stability of the hybrid film towards corrosive liquids, water droplets of different pH values prepared using HCl and NaOH were cautiously dropped onto the hybrid films. Additionally, a durability test was carried out by immersing the hybrid films coated by 3 wt% BAF-fa/1 wt% SiO2 on the glass surface in tap water and ethanol. The measurements were performed under two different conditions, at room temperature and at 70 °C. The hybrid films were immersed in both solvents for 30 and 60 min and then were dried in an oven for two hours.
Results and discussion
Structural confirmation of BAF-fa and P(BAF-fa)
By employing a one-pot Mannich reaction, the BAF-fa monomer was successfully synthesized. Fig. 1 illustrates the 1H NMR spectra of BAF-fa and P(BAF-fa). The peaks for Ar–CH2–N and N–CH2–O observed in the 1H NMR spectrum are specific to the oxazine ring, and are distinct from those for the benzoxazine monomer.18,23 For the BAF-fa monomer, the characteristic protons of Ar–CH2–N and N–CH2–O appear at 4.45 ppm, and 5.23 ppm. Moreover, the aromatic protons have chemical shifts in the range of 6.57 to 7.28 ppm (Fig. 1a). After the ring opening polymerization of the monomer a slight change in the chemical shifts was observed.34,35 The protons of Ar–CH2–N have a chemical shift of 4.71 ppm, while the chemical shift for the trisubstituted phenolic proton appears at 5.37 ppm. The aromatic protons have chemical shifts of 6.8 to 7.6 ppm (Fig. 1b). 19F NMR data gives further affirmation of the structure of the BAF-fa monomer (Fig. 2). The chemical shift for N–Ar–F is 120.26 ppm, while for C–CF3 it is 63.87 ppm.36 The 13C NMR spectrum of the BAF-fa monomer signifies the final verification (Fig. 3). The chemical shift for R4C is 50.34 ppm, for CF3 is 77.87 ppm, for R–C
C is 129.23 ppm, for O–CH2–N is 80.1 ppm, for R–CH2–N is 76.34 ppm and for aromatic C
C is around 115–125 ppm.37
Affirmation of the presence of functional groups is by FT-IR spectroscopy. The characteristic bands (for mono substituted benzene) at 720 and 692 cm−1 did not appear.37,38 However, the typical distinguishing bands for the oxazine ring at 835 cm−1, for C–O–C at 950 cm−1, CH2 wagging at 1261 cm−1, and the p-substituted benzene ring at 748 cm−1 were present. For the trisubstituted benzene ring, the absorption band appeared at 1388 cm−1 (Fig. 4). The decrease in peak resolution of this band for the trisubstituted benzene ring in the FT-IR spectrum of the polymeric structure indicated the ring-opening phenomenon in oxazine and thus validated the transformation of monomers into oligomers and polymers.
Curing behavior of BAF-fa
Typically, benzoxazine undergoes exothermic ring opening reactions at around 200–250 °C, which can be monitored using DSC. The DSC thermogram revealed an exothermic ring-opening reaction having an onset temperature of 200.8 °C and a peak point at 237.99 °C. The energy of the exothermic ring-opening reaction is 191.9 J g−1 (Fig. 5). Thus, the results of DSC for the novel benzoxazine monomers indicated that the presence of fluoro groups in the benzoxazine structure has no perceptible effect on the polymerization behavior of the monomers and a high temperature is still obligatory for polymerization.
Wetting behavior of BAF-fa/SiO2 films
It has been established experimentally that the contact angle alters as the roughness changes in accordance with the Wenzel or Cassie–Baxter model, dependent upon whether the surface is hydrophilic or hydrophobic. In PBZ the strong intramolecular hydrogen bonding between the hydroxyl groups and the amino groups in the Mannich bridges tends to decrease the surface free energy. Thus, a smooth glass surface coated with pure P(BAF-fa) has a WCA of 94°, indicating the hydrophobicity of the modified surface. To amend the hydrophobic nature of the modified glass surface to make it superhydrophobic, SiO2 nanoparticles were added to the monomer solution prior to spray coating. The hybrid solutions containing a fixed concentration of the BAF-fa monomer with varying concentrations of SiO2 nanoparticles were spray coated on the glass surface and then subsequently cured at 220 °C for 1 h. The as-cured films show considerable increase in WCA after the creation of roughness over multiple scales (Table 1).
Table 1 Static WCAs and roughness of pure P(BAF-fa) and hybrid P(BAF-fa)/SiO2 films coated on the glass surface
Sample |
Conc. of BAF-fa (wt%) |
Conc. of SiO2 in BAF-fa (wt%) |
WCAs (°) |
Roughness (rms) (nm) |
A |
3 |
0 |
94 ± 3 |
10.5 ± 9 |
B |
3 |
0.1 |
102 ± 3 |
80.5 ± 11 |
C |
3 |
0.5 |
135 ± 3 |
126 ± 13 |
D |
3 |
1 |
157 ± 2 |
156 ± 11 |
E |
3 |
3 |
163 ± 2 |
202 ± 21 |
The low surface energy of the benzoxazine matrix not only induces the hydrophobicity but also provides a medium for the facile dispersion of SiO2 nanoparticles. After the curing of the hybrid films, the cross-linked structure of the P(BAF-fa) entangled the nanoparticles through attractive electrostatic forces.26 The increased concentration of SiO2 nanoparticles results in an enhanced roughness within the polymerized structure of the P(BAF-fa). By adding 0.1 and 0.5 wt% of SiO2 nanoparticles to the monomer solution, the obtained WCAs were moderately low relative to those required for the hybrid film to be characterized as superhydrophobic. So by further increasing the concentration of the SiO2 nanoparticles the desired superhydrophobicity with significantly lower surface tilt angle and minute WCAH was achieved (Fig. 6a,b).
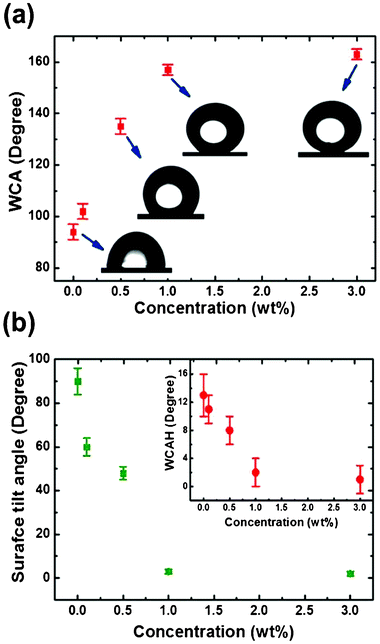 |
| Fig. 6 (a) WCA for 3 wt% P(BAF-fa) films with various concentrations of SiO2 nanoparticles coated on the glass surface. (b) Surface tilt angle and WCAH (inset) for 3 wt% P(BAF-fa) films with various concentrations of SiO2 nanoparticles coated on the glass surface. | |
The 1 wt% increase in the SiO2 nanoparticle content results in the desired WCA of 157° required to classify the films as perfectly superhydrophobic with the lotus effect.9 A further increase in the SiO2 nanoparticle content increases the WCA, and tends to minimize the adhesion of the P(BAF-fa)/SiO2 hybrid film on the glass substrate.26,39 Therefore, in this study, the optimum hybrid film using 3 wt% P(BAF-fa)/1 wt% SiO2 (sample D) has been used to carry out a detailed investigation.
Another very significant wetting property used to study the contact behaviour of a water droplet is the WCAH, which depicts the ability of a surface to oppose the movement of a droplet.40 In fluid flow, films suitable for application in self-cleaning and having low drag should have very low WCAH with high WCAs. The minute WCAH = θa − θr measured was 2 ± 2° and the measured surface tilt angle was around 3 ± 1° for the hybrid film with 3 wt% P(BAF-fa)/1 wt% SiO2 (Fig. 6b). Hybrid films with such minute surface tilt angles and WCAH are of interest in various applications, such as exterior paints for buildings, navigation in ships, roof tiles, and applications requiring anti-fouling and a reduction of drag in fluid flow, (e.g., in micro/nanochannels and energy conservation and conversion).41–43
To further scrutinize the water droplet contact behavior of the films, comparative measurements were made. A comparison of water droplet movement towards pure and hybrid P(BAF-fa) coated glass surfaces is shown in Fig. 7. Firstly, a 3 μL water droplet on a syringe approaches the sessile glass surface coated with pure P(BAF-fa) film (Fig. 7a). After touching the coated surface, the water droplet easily detaches from the syringe, and it spreads out with ease on the surface (image 3 of Fig. 7a). In Fig. 7b, the movement of a water droplet towards the glass surface coated with the hybrid P(BAF-fa)/SiO2 film is shown. The water droplet starts to contact the coated surface, but remains attached to the needle of the syringe. Due to the roughness created by the hierarchical structure of the hybrid film on the glass surface, the water droplet fails to spread out (image 2 of Fig. 7b).44 After continued movement of the needle towards the film surface, it puts forth incessant force on the water droplet to release it (images 3 and 4 of Fig. 7b), but the water droplet still fails to spread out, indicating the very low adhesion of the water droplet to the hybrid surface. In addition, when the needle is removed, the water droplet is dragged back without any remnant left on the surface (images 5 and 6 of Fig. 7b). This water transport behavior is of great importance for applications in the transport of microdroplets.45
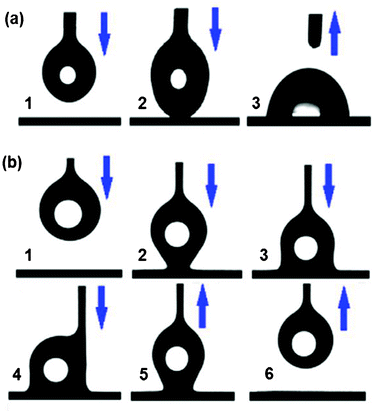 |
| Fig. 7 The behavior of a water droplet moving towards the glass surface coated by pure and hybrid films. (a) A series of images for the glass surface coated by 3 wt% BAF-fa, illustrating the ease with which the water droplet sticks to the film just after touching the surface. (b) A series of images for the glass surface modified by 3 wt% P(BAF-fa)/1 wt% SiO2. (1) Movement of the syringe releasing a 3 μL water droplet towards the sessile film, (2) the water droplet touching the surface, (3,4) the needle being pushed to the surface to overcome the surface tension forces, (5) backward movement of the syringe and (6) finally the water droplet is pulled back without any loss. | |
Surface topographies of hybrid films coated on the glass surface
The top morphology measured by FE-SEM have revealed that the film coated with P(BAF-fa) has a flat morphology but is hydrophobic in nature (Fig. 8a). The 3D AFM image shows negligible roughness (10 nm), which was measured over an area of 10 μm × 10 μm, which is attributed to solvent evaporation during the curing procedure (Fig. 8a′). By increasing the roughness of the hydrophobic film, superhydrophobic films were obtained, which could be explained by Wenzel's equation to describe the water contact angle at a rough surface:46where θ is the intrinsic contact angle at a smooth surface, θr is the contact angle at a rough surface created on the same smooth surface and r is the roughness factor. According to this equation, upon increasing the surface roughness of a hydrophilic material (θ < 90°) the water contact angle decreases, while upon increasing the surface roughness of a hydrophobic material (θ > 90°) the water contact angle increases. In our case, the glass surface coated by P(BAF-fa) was hydrophobic in nature, so by increasing the hierarchical structures of the surface, superhydrophobicity is obtained, which is confirmed by FE-SEM and AFM images. As the concentration of the SiO2 nanoparticles increases, the roughness of the coated film tends to be increased. The low concentrations of SiO2 (sample A and B) impart less roughness to the films, which are not as superhydrophobic (Fig. 8b,c).
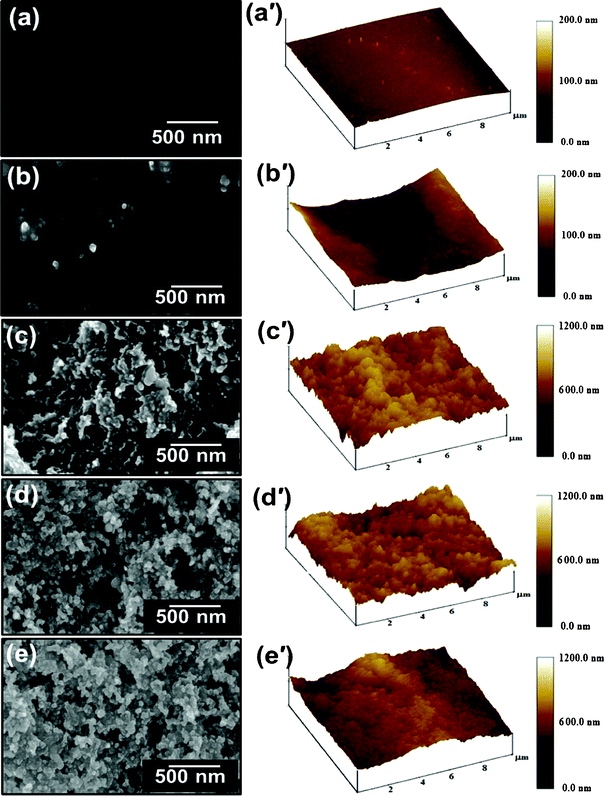 |
| Fig. 8 (a–e) FE-SEM and (a′–e′) corresponding AFM images of pure and hybrid films containing different concentrations of silica nanoparticles coated on the glass surface: (a) 3 wt% P(BAF-fa), (b) 3 wt% P(BAF-fa)/0.1 wt% SiO2, (c) 3 wt% P(BAF-pfa)/0.5 wt% SiO2, (d) 3 wt% P(BAF-pfa)/1 wt% SiO2, and (e) 3 wt% P(BAF-pfa)/3 wt% SiO2. | |
The superhydrophobic hybrid films of P(BAF-fa)/SiO2 exhibit both micro and nanoscale binary structures (hierarchical structure).44 Here, each microisland having a diameter of 300–500 nm was randomly modified within the P(BAF-fa) matrix by covering with nanospheres with size in the range of 20–40 nm (Fig. 8d,e). This composite structure considerably increases the surface roughness of the film, in which air bubbles can be easily trapped to induce superhydrophobicity. The 3D AFM images of samples C, D and E show a definite increase in microscale roughness induced by the SiO2 nanoparticles within the P(BAF-fa) matrix from 80 to 202 nm. (Fig. 8b′–e′). Therefore, the extensive investigation of roughness-induced hydrophobicity has shown that the trapping of air in the cavities of a rough surface results in a composite solid–liquid–air interface, as happened in the case of the hybrid film with P(BAF-fa) with different SiO2 concentrations. The hierarchical roughness and low surface energy imparted by the BAF-fa/SiO2 will prevent a water droplet from contacting the solid surface completely due to the trapped air bubbles at the solid–liquid interface. Cassie and Baxter proposed eqn (2) to describe the relationship between the contact angle on a flat surface (θ) and a rough surface (θr) composed of a solid and air:
Here, f1 and f2 are the fractions of the solid surface and air in contact with the liquid, respectively, that is, f1 + f2 = 1. Given the WCAs of the P(BAF-fa) flat spray coated film (95°) and the BAF-fa/SiO2 hybrid film (157°), the f2 value of the rough surface was calculated to be 0.912, which indicated that the superhydrophobicity of the hybrid film could be attributed to the air trapped in the rough hierarchical micro/nanostructures of the membranes.
Transmittance and durability measurements
The transparency of the pure P(BAF-fa) and hybrid P(BAF-fa)/SiO2 films were examined and the maximum transparency was shown by the pure film. As expected, when increasing the concentration of the SiO2 the transparency decreased (Fig. 9). Interestingly, the hybrid films are superhydrophobic not only for pure water but also for a broad range of pH values (Fig. 10). The measured contact angles for solutions of different pH values on the hybrid film are around 157°. So the as prepared superhydrophobic films can be used with water with a range of pH values. Previously, nanostructured carbon films prepared from polyacrylonitrile nanofibers, aluminum surfaces treated with NaOH and then modified by perfluorononane and vinyl-terminated poly-(dimethylsiloxane), polyaniline/polystyrene films by electrospinning and later, the use of BA-m have illustrated superhydrophobicity across a broad pH range.26,47–49
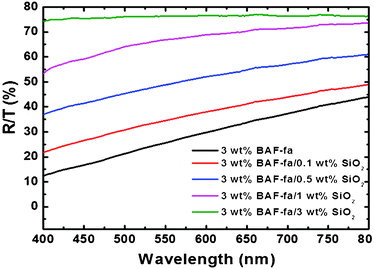 |
| Fig. 9 Transmittance measurements of pure P(BAF-fa) and hybrid P(BAF-fa)/SiO2 films coated on the glass surface. | |
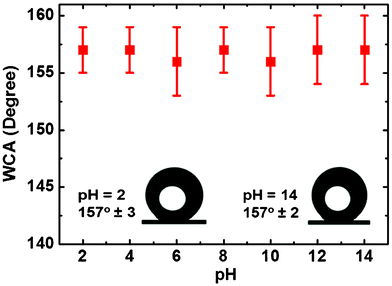 |
| Fig. 10 Contact angles of solutions of different pH on the hybrid film of 3 wt% P(BAF-fa)/1 wt% SiO2 coated on the glass surface. | |
The SiO2 nanoparticles have created adequate roughness to adapt the chemical structure of P(BAF-fa) to form hybrid superhydrophobic films. Moreover, these films are not wettable by pure water. Different surfactants were used to reduce the surface tension and to make the superhydrophobic films wet.50 Previously, a water/ethanol mixture has been used to wet superhydrophobic films.51 So, to check the durability of the as prepared hybrid films, two solvents were chosen: water with high surface tension (71.97 mN m−1), and ethanol with comparatively low surface tension (22.27 mN m−1). The WCAs measured after immersion and drying in the two solvents are shown in Table 2.
Table 2 Durability measurements of the hybrid P(BAF-fa)/SiO2 film coated on the glass surface after immersion in tap water and ethanol at different temperatures
Time (min) |
WCAs measured after immersion in tap water at |
WCAs measured after immersion in ethanol at |
Room temp. |
70 °C |
Room temp. |
70 °C |
0 |
157 ± 2° |
157 ± 2° |
157 ± 2° |
157 ± 2° |
30 |
156 ± 2° |
155 ± 2° |
155 ± 2° |
153 ± 2° |
60 |
156 ± 2° |
152 ± 2° |
153 ± 2° |
149 ± 2° |
A trivial decrease in WCA was observed in both cases but the hybrid films maintained their superhydrophobicity. The SiO2 nanoparticles present at the surface of the hybrid film tended to adsorb water and ethanol molecules, but after drying in the oven, the absorbed molecules could be easily removed. After water immersion for 30 min, a very small change in the WCA of the film was measured. When increasing the time of immersion, a larger decrease in the WCA was observed. In the case of pure ethanol immersion, the decrease in the WCA was a little bit larger, but the films were still superhydrophobic in nature. The hydroxyl groups on the silica nanoparticle surfaces dispersed in the P(BAF-fa) matrix tend to adsorb water molecules after immersion in the binary solvent. The water molecules can be easily removed by drying the composite film under vacuum. The hybrid film, which maintained its superhydrophobicity after immersion in water and particularly in an organic solvent (ethanol) for a long duration of time (30–60 min), has not been reported earlier. Thus, the as prepared hybrid films are important for academic research and are industrially relevant.
Conclusion
In summary, a combination of low surface energy fluorinated PBZ and surface roughness induced by SiO2 is employed to make superhydrophobic films, having a WCA of 163°. The films were prepared by a simple and inexpensive, one-step spray coating method. The chemical structure of the newly synthesized BAF-fa was confirmed by FT-IR and NMR spectroscopy, while surface topography and roughness was measured using FE-SEM and AFM. Surface roughness and surface heterogeneity resulted in very small WCAH of 2 ± 2° and a surface tilt angle of around 3 ± 1° for the optimum hybrid film, thus proving an extraordinary material for low drag and self-cleaning applications. The hybrid films composed of SiO2 nanoparticles embedded in the P(BAF-fa) matrix exhibited superhydrophobicity for water over a broad pH range, consequently showing their appropriateness for coating materials in a corrosive atmosphere. Moreover, the durability measurements of the coated films in water and ethanol have also shown promising results showing their wider industrial applicability.
Acknowledgements
This work is supported by the National Basic Research Program of China (973 Program, 2011CB606103 and 2012CB525005), the National Natural Science Foundation of China (no. 51173022), the “111 Project” (no. 111-2-04 and B07024), the Shanghai Committee of Science and Technology (no. 10JC1400600), the Shanghai Nano Special Projects (11nm0502900), the Innovation Program of Shanghai Municipal Education Commission (11ZZ59), the “Dawn” Program of Shanghai Education Commission (10SG32), the Scientific Research Foundation for the Returned Overseas Chinese Scholars, Ministry of Education of China, the Program for New Century Talents of the University in China, and the Fundamental Research Funds for the Central Universities.
References
- Y. T. Cheng and D. E. Rodak, Appl. Phys. Lett., 2005, 86, 144101–144103 CrossRef.
- R. D. Narhe and D. A. Beysens, Europhys. Lett., 2006, 75, 98–104 CrossRef CAS.
- J. Du and Y. L. Hsieh, Cellulose, 2009, 16, 247–260 CrossRef CAS.
- F. Likibi, B. Jiang and B. Li, J. Mater. Res., 2008, 23, 3222–3228 CrossRef CAS.
- M. Miwa, A. Nakajima, A. Fujishima, K. Hashimoto and T. Watanabe, Langmuir, 2000, 16, 5754–5760 CrossRef CAS.
- N. J. Shirtcliffe, G. McHale, M. I. Newton and C. C. Perry, Langmuir, 2003, 19, 5626–5631 CrossRef CAS.
- D. O. H. Teare, C. G. Spanos, P. Ridley, E. J. Kinmond, V. Roucoules, J. P. S. Badyal, S. A. Brewer, S. Coulson and C. Willis, Chem. Mater., 2002, 14, 4566–4571 CrossRef CAS.
- K. Acatay, E. Simsek, C. Ow-Yang and Y. Z. Menceloglu, Angew. Chem., Int. Ed., 2004, 43, 5210–5213 CrossRef CAS.
- F. Zhao, X. Wang, B. Ding, J. Lin, J. Hu, Y. Si, J. Yu and G. Sun, RSC Adv., 2011, 1, 1482–1488 RSC.
- M. Sun, X. Li, B. Ding, J. Yu and G. Sun, J. Colloid Interface Sci., 2010, 347, 147–152 CrossRef CAS.
- B. Ding, C. Li, Y. Hotta, J. Kim, O. Kuwaki and S. Shiratori, Nanotechnology, 2006, 17, 4332–4339 CrossRef CAS.
- M. Guo, B. Ding, X. Li, X. Wang, J. Yu and M. Wang, J. Phys. Chem. C, 2010, 114, 916–921 CAS.
- J. Lin, Y. Cai, X. Wang, B. Ding, J. Yu and M. Wang, Nanoscale, 2011, 3, 1258–1262 RSC.
- J. Bico, C. Marzolin and D. Quéré, Europhys. Lett., 1999, 47, 743 CrossRef CAS.
- J. P. Youngblood and N. R. Sottos, MRS Bull., 2008, 33, 732–741 CrossRef CAS.
- X. Wang, B. Ding, J. Yu and M. Wang, Nano Today, 2011, 6, 510–530 CrossRef CAS.
- J. Lin, X. Wang, B. Ding, J. Yu, G. Sun and M. Wang, Crit. Rev. Solid State Mater. Sci., 2012, 37, 94–114 CrossRef CAS.
- X. Ning and H. Ishida, J. Polym. Sci., Part B: Polym. Phys., 1994, 32, 921–927 CrossRef CAS.
- V. M. Russell, J. L. Koenig, H. Y. Low and H. Ishida, J. Appl. Polym. Sci., 1998, 70, 1413–1425 CrossRef CAS.
- J. A. Macko and H. Ishida, Polymer, 2001, 42, 6371–6383 CrossRef CAS.
- W. J. Burke, J. L. Bishop, E. L. M. Glennie and W. N. Bauer, J. Org. Chem., 1965, 30, 3423–3427 CrossRef CAS.
- H. D. Kim and H. Ishida, Macromol. Symp., 2003, 195, 123–140 CrossRef CAS.
- H. Ishida and H. Y. Low, Macromolecules, 1997, 30, 1099–1106 CrossRef CAS.
- C. F. Wang, Y. C. Su, S. W. Kuo, C. F. Huang, Y. C. Sheen and F. C. Chang, Angew. Chem., Int. Ed., 2006, 45, 2248–2251 CrossRef CAS.
- C. F. Wang, S. F. Chiou, F. H. Ko, C. T. Chou, H. C. Lin, C. F. Huang and F. C. Chang, Macromol. Rapid Commun., 2006, 27, 333–337 CrossRef CAS.
- C. F. Wang, Y. T. Wang, P. H. Tung, S. W. Kuo, C. H. Lin, Y. C. Sheen and F. C. Chang, Langmuir, 2006, 22, 8289–8292 CrossRef CAS.
- S. W. Kuo, Y. C. Wu, C. F. Wang and K. U. Jeong, J. Phys. Chem. C, 2009, 113, 20666–20673 CAS.
- C. S. Liao, C. F. Wang, H. C. Lin, H. Y. Chou and F. C. Chang, J. Phys. Chem. C, 2008, 112, 16189–16191 CAS.
- C. S. Liao, C. F. Wang, H. C. Lin, H. Y. Chou and F. C. Chang, Langmuir, 2009, 25, 3359–3362 CrossRef CAS.
- H. J. Yu and Z. H. Luo, J. Polym. Sci., Part A: Polym. Chem., 2011, 49, 174–183 CrossRef CAS.
- H. J. Yu and Z. H. Luo, J. Polym. Sci., Part A: Polym. Chem., 2010, 48, 5570–5580 CrossRef CAS.
- L. Qu and Z. Xin, Langmuir, 2011, 27, 8365–8370 CrossRef CAS.
- H. Ishida and H. Y. Low, J. Appl. Polym. Sci., 1998, 69, 2559–2567 CrossRef CAS.
- N. N. Ghosh, B. Kiskan and Y. Yagci, Prog. Polym. Sci., 2007, 32, 1344–1391 CrossRef CAS.
- T. Takeich and T. Agag, High Perform. Polym., 2006, 18, 777–797 CrossRef.
- Y. X. Wang and H. Ishida, Polymer, 1999, 40, 4563–4570 CrossRef CAS.
- H. Ishida and D. P. Sanders, Macromolecules, 2000, 33, 8149–8157 CrossRef CAS.
- T. Agag and T. Takeichi, Macromolecules, 2001, 34, 7257–7263 CrossRef CAS.
- C. F. Wang, T. F. Wang, C. S. Liao, S. W. Kuo and H.C. Lin, J. Phys. Chem. C, 2011, 115, 16495–16500 CAS.
- R. H. Dettre and R. E. Johnson, J. Phys. Chem., 1965, 69, 1507–1515 CrossRef CAS.
- M. Nosonovsky and B. Bhushan, J. Phys.: Condens. Matter, 2008, 20 Search PubMed.
- M. Nosonovsky and B. Bhushan, Curr. Opin. Colloid Interface Sci., 2009, 14, 270–280 CrossRef CAS.
- M. Nosonovsky and B. Bhushan, Philos. Trans. R. Soc. London, Ser. A, 2009, 367, 1511–1539 CrossRef CAS.
- L. Jiang, Y. Zhao and J. Zhai, Angew. Chem., Int. Ed., 2004, 43, 4338–4341 CrossRef CAS.
- R. Mohammadi, J. Wassink and A. Amirfazli, Langmuir, 2004, 20, 9657–9662 CrossRef CAS.
- Z. Burton and B. Bhushan, Nano Lett., 2005, 5, 1607–1613 CrossRef CAS.
- L. Feng, Z. Yang, J. Zhai, Y. Song, B. Liu, Y. Ma, Z. Yang, L. Jiang and D. Zhu, Angew. Chem., Int. Ed., 2003, 42, 4217–4220 CrossRef CAS.
- Z. Guo, F. Zhou, J. Hao and W. Liu, J. Am. Chem. Soc., 2005, 127, 15670–15671 CrossRef CAS.
- Y. Zhu, J. Zhang, Y. Zheng, Z. Huang, L. Feng and L. Jiang, Adv. Funct. Mater., 2006, 16, 568–574 CrossRef CAS.
- T. Soeno, K. Inokuchi and S. Shiratori, Appl. Surf. Sci., 2004, 237, 543–547 CAS.
- X. Hong, X. Gao and L. Jiang, J. Am. Chem. Soc., 2007, 129, 1478–1479 CrossRef CAS.
|
This journal is © The Royal Society of Chemistry 2012 |