DOI:
10.1039/C2RA21054A
(Paper)
RSC Adv., 2012,
2, 9635-9642
Tuning of the electronic and optical properties of 4,4'-bis(1-phenyl-phenanthro[9,10-d]imidazol-2-yl)biphenyl via cyano substitution in un-conjugated phenyl†
Received
28th May 2012
, Accepted 8th August 2012
First published on 10th August 2012
Abstract
A new phenanthroimidazole-based material named 4,4'-bis(1-(4-cyano-phenyl)-1H-phenanthro[9,10-d]imidazol-2-yl)biphenyl (CN-BPPI) was prepared by simple one-pot chemical reaction. Its structure and its photophysical, electrochemical and mobility properties were carefully studied. CN-BPPI exhibited similar absorption and emission spectra to BPPI, while the thermal stability and photoluminescence quantum yield were enhanced. More balanced carrier mobility was realized in CN-BPPI due to the substitution of cyano, which was proved by cyclic voltammetry (CV) and single-carriers measurements. The electroluminescence (EL) properties of a blue emitting CN-BPPI device were researched and the EL performances were much better than those for BPPI.
1. Introduction
Organic light-emitting devices (OLEDs) have been attracting considerable attention due to their potential application in full color flat panel displays in the past decades.1 Nowadays, with the rapid improvement in performances of OLEDs, large amounts of efficient green and red emitters have already emerged. However, highly efficient blue emitters are still very limited in comparison. Unbalanced charge injection and transport is one of the major problems in blue OLEDs. The high energy gap essential for blue emission often results in low electron affinities, which hampers the electron injection balance of the charge carriers and the efficiency of blue OLEDs.2,3 Moreover, since most conjugated molecules are p-type semiconductors due to their inherent richness of π-electrons, balanced charge injection and transport in OLEDs are hard to realize.4 Generally, multilayered devices are applied to improve performance, in which an electron transport layer and a hole-transport layer are used to inject and transport electrons and holes into the emissive layer.5 However, the fabrication of such devices is more complicated and expensive. In order to achieve simpler OLED structures, the design of multifunctional blue emitters to modify the light emission and charge injection is of vital importance.6
Recently, phenanthroimidazole-based derivatives have attracted more and more attention as efficient blue emitters and electron-transport materials, accompanied with excellent thermal properties, high glass-transition temperatures (Tg) and high photoluminescence (PL) quantum yields. Kuo et al. reported the synthesis of a series of blue-emitting bis(phenanthro-imidazolyl)biphenyl derivatives and their external quantum efficiencies up to 6.31% in non-doped devices with Commission Internationale De L′Eclairagey (CIEy) < 0.15.7 Yuan et al. employed phenanthroimidazole derivatives as an organic buffer layer inserted between NPB and Alq3 layers to balance hole-transport ability, and the maximum brightness and luminance efficiency (LE) of the device were doubled.8 Zhang et al. introduced thiophene and triphenylamine groups to tune the highest occupied molecular orbital (HOMO) level and hole transport mobility, and realized high efficiencies with CIEy < 0.10.9 Our group has developed a series of phenanthroimidazole-based materials containing oligomers and polymers, and has discussed the mechanism of fluorescence.10 All of these research data demonstrate that phenanthroimidazole is an excellent building block for blue-light-emitting materials.
In our previous paper, we have shown that 4,4'-bis(1-phenyl-phenanthro[9,10-d]imidazol-2-yl)biphenyl (BPPI) is a good blue emitter with n-type properties.10a The simply structured device based on BPPI exhibited an even higher performance than the multi-layered one with 1,3,5-tris(N-phenylbenzimidazol-2-yl)benzene (TPBi) as an electron injection and transport layer. However, the electron injection barrier remained high (0.9 eV) between the organic layer and the cathode, and the electron mobility was one order of magnitude lower than the hole mobility. Cyano-substitution could effectively modify the interface to decrease this barrier, and limit hole-transport ability to balance the carrier injection and transport, but, at the same time, the PL and EL could exhibit obvious red-shift because of the increased degree of conjugation.11
To preserve the blue emission of BPPI and balance the charge injection and transport, the cyano-group was inserted at the N1 position of the phenyl in the imidazole ring, which does not participate in the formation of the frontier molecular orbitals according to quantum chemical calculations of their electronic structure done in our previous work10a,c and so the molecular ground state and excited state should not be changed by this. Cyano-substituted BPPI (CN-BPPI) was easily prepared from commercially available starting materials through a simple one-step reaction in high yield, and the purity reached 99% by recrystallization from DMF (Scheme 1).† The absorption and fluorescence spectra of CN-BPPI in solution and as a solid showed little change, and the PL quantum yields were enhanced nearly to 100%. It exhibited more excellent thermal stability with a Tg of 217 °C (Tg of BPPI = 195 °C), and the hole and electron mobilities could reach the same level. The current efficiency (ηc) of the non-doped double-layer EL device based on CN-BPPI was improved nearly four times compared to BPPI.
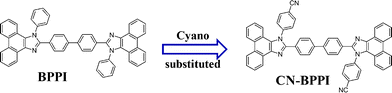 |
| Scheme 1 The structure of BPPI and CN-BPPI. | |
CN-BPPI shows comprehensive enhancement in basic characteristics involving lower preparation costs and better thermal stability, fluorescence efficiency and charge injection properties, and it is proved that appropriate minor alteration of the classic materials could realize the improved performance of materials. Here, we reported these results.
2. Materials and measurement
All the reagents and solvents used for the syntheses were purchased from Aldrich or Acros companies and used as received. All reactions were performed under a dry nitrogen atmosphere.
The 1H NMR spectra were recorded on AVANCZ 500 spectrometers at 298 K by utilizing deuterated dimethyl sulphoxide (DMSO) as the solvent and tetramethylsilane (TMS) as the standard. The compounds were characterized by a Flash EA 1112, CHNS-O elemental analysis instrument. The MALDI-TOF-mass spectra (MS) were recorded using an AXIMA-CFR™ plus instrument. UV-vis absorption spectra were recorded on a UV-3100 spectrophotometer. Fluorescence measurements were carried out with RF-5301PC. The fluorescence quantum yield in solution was measured using a 0.5 M H2SO4 solution of quinine as the reference (0.54), and the solid state quantum yield on the quartz plate was measured by an integrating sphere apparatus using Alq3 as the reference (0.22). The differential scanning calorimeter (DSC) analysis was carried out using a NETZSCH (DSC-204) instrument at 10 °C min−1 under nitrogen flushing. Cyclic voltammetry (CV) was performed with a BAS 100 W Bioanalytical System, using a glass carbon disk (Φ = 3 mm) as the working electrode, platinum wire as the auxiliary electrode with a porous ceramic wick and Ag/Ag+ as the reference electrode, standardized for the redox couple ferricinium/ferrocene. All solutions were purged with a nitrogen stream for 10 min before measurement. The procedure was performed at room temperature and the nitrogen atmosphere was maintained over the solution during measurements.
3. Device fabrication
The EL devices were fabricated by vacuum deposition of the materials at 10−6 Torr onto ITO glass with a sheet resistance of 25 Ω square−1. All of the organic layers were deposited at a rate of 1.0 Å s−1. The cathode was deposited with LiF (1 nm) at a deposition rate of 0.1 Å s−1 and then capped with Al metal (100 nm) through thermal evaporation at a rate of 4.0 Å s−1. The EL spectra and CIE coordination of these devices were measured by a PR650 spectro scan spectrometer. The luminance–current and density–voltage characteristics were recorded simultaneously with the measurement of the EL spectra by combining the spectrometer with a Keithley model 2400 programmable voltage–current source. All measurements were carried out at room temperature under ambient conditions.
4. Synthesis and characterization
4.1 Synthesis
BPPI and CN-BPPI were synthesized in a one-pot reaction.†7–10,12 A mixture of aniline (or 4-aminobenzonitrile), phenanthrenequinone, ammonium acetate and 4,4'-biphenyldicarboxaldehyde was refluxed in acetic acid for 2 h, then poured into a methanol solution with stirring. After filtering, BPPI was purified by chromatography and the yield was above 80.5% in our previous work. Because of the existence of the cyano-group, the compound easily formed intermolecular hydrogen bonds or strong interactions with the polar solution. The recrystallization of CN-BPPI was attempted in ethanol, acetone, acetonitrile, DMF, acetic acid and DMSO. The light-yellow needle crystals were gained using DMF. After two recrystallization repeats from DMF, the purity reached 99%, which was the same as from using the column chromatography purification method, and no impurities were observed by NMR and elemental analysis. This product cost was reduced and the environmental friendliness of CN-BPPI purification was improved significantly, which is very important for its commercial application.
4.2 NMR characterization
Fig. 1 shows a clear 1H NMR spectra of BPPI and CN-BPPI in the aromatic range. The doublets appearing at 8.92, 8.88, 8.70 and 7.08 ppm correspond to resonance of the protons at the 1, 4, 5 and 8-positions, as shown in the spectra for the two compounds. The triplets at 7.57 and 7.34 ppm are affirmed as the resonances of the hydrogen atoms at the 3 and 6-positions. More detailed structural information for the 2, 7, 9–12, a-c-positions of BPPI was not delivered in our previous paper.10a Fortunately, the fine splitting appeared after the cyano-group was introduced in CN-BPPI. The two obvious doublets, which were observed at 8.23 and 8.08 ppm, corresponding to the protons at the a and b-positions, were shifted to lower field because of the electron-withdrawing effect of the cyano group. The new triplets appeared at 7.80 and 7.72 ppm, corresponding to the protons at the 2 and 7-positions, and the doublet at 7.76 and 7.63 ppm are affirmed as the resonances of the 9,10,11 and 12-postions. All the peaks observed in the 1H NMR spectra correspond well to their respective structures. This accurate confirmation is also beneficial to the structural analysis of other phenanthroimidazole-based materials.
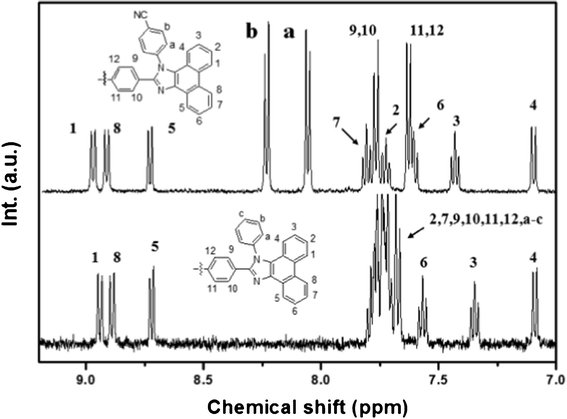 |
| Fig. 1
1H NMR spectra of BPPI and CN-BPPI in the aromatic range. | |
4.3 FTIR characterization
FTIR studies clearly suggested an absorption band due to the cyano group, which exhibits strong vibration normally around 2200 cm−1. For CN-BPPI, the vibrational frequency was observed obviously at 2224 cm−1, while there was no absorption here in the BPPI spectrum (Fig. 2). The peak appearing at 693 cm−1 was attributed to the mono-substituted aromatic C–H vibration in BPPI, which originates from the only mono-substituted phenyl attached to N1 in imidazole. This peak disappeared in CN-BPPI, and a new response at 865 cm−1 appeared, which was attributed to the 1,4-disubstituted phenyl attached to N1. The IR data proved that the cyano group was inserted into BPPI as expected. Due to the presence of the electron-withdrawing cyano group, the C
C vibration in all aromatic structures from 1430 to 1600 cm−1 shifted to the longer wave-number field. Another difference between the two compounds can be seen at around 3000 cm−1, which was attributed to the C–H stretching vibration of the aromatic structure. The absorption band in CN-BPPI shifted to the lower wave-number field, and the intensity was stronger than BPPI, which might imply the formation of a hydrogen bond between the cyano group and the C–H in the aromatic ring as detailed in our previous research.10 The existence of the non-covalent interaction is also consistent with our experiments below.
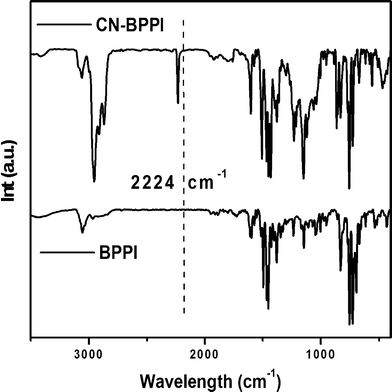 |
| Fig. 2 IR spectra of BPPI and CN-BPPI. | |
4.4 Thermal properties
The thermal properties of BPPI and CN-BPPI were investigated by thermal gravimetric analyses (TGA) and DSC under a nitrogen atmosphere (Fig. 3). The two compounds show the same decomposition progress. The temperatures corresponding to a 5% weight loss (Td) were the same, at 535 °C, and this might originate from them having the same main structure. Even when pyrolyzed at 800 °C, more than 65% of CN-BPPI's weight and 41% of BPPI's weight were left, suggesting that they were promising precursors for non-oxide ceramic materials. CN-BPPI showed a distinct glass transition at 217 °C in the second heating run. This value represented a marked improvement compared to the Tg of 195 °C for BPPI. The improved Tg was probably due to the intermolecular C–N⋯H or C–N⋯π interactions between the cyano-group and the phenanthrene plane, which can induce more condensed molecular packing, as was proved by our previous research.13 The higher Tg value implicated that CN-BPPI could form morphologically stable amorphous films upon thermal evaporation, which is highly important for application in OLEDs.
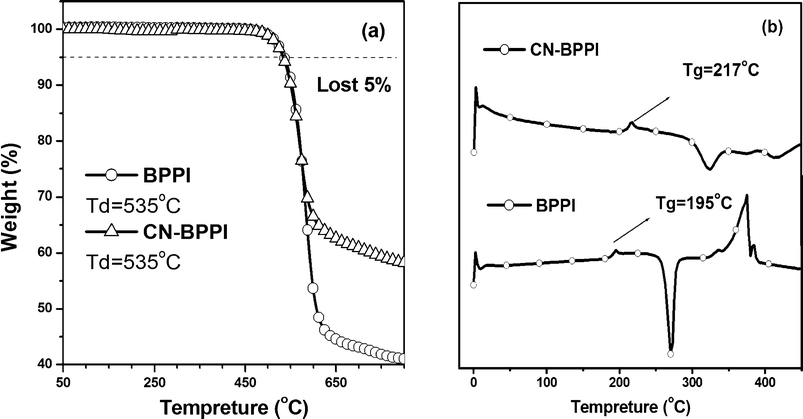 |
| Fig. 3 The TGA (a) and DSC (b) graphs of BPPI and CN-BPPI. | |
4.5 Photophysical properties
Absorption and photoluminescence studies were performed on BPPI and CN-BPPI in THF (10−5 M) and as solids on quartz to investigate the relationship between the cyano substituents and the physical properties (Fig. 4). When a cyano-group is inserted, the increase in the degree of conjugation or the change in the charge-transfer (CT) state would usually cause the absorption and photoluminescence spectra to red-shift.11 But in CN-BPPI, the spectra in solution and in solid do not show remarkable differences compared with BPPI. The maximum absorption at about 260 nm originates from their common benzene attached to N1 in the imidazole ring, and a shoulder at around 369 nm is assigned to the π–π* electronic transition in the conjugated system.14 The main emission peak is at 420 nm and there is a shoulder at 442 nm in solution, and they show a red-shift to 468 nm without the fine structure. As the value of full-width at half-maximum (FWHM) is 30 nm, this red-shift progress can not be due to aggregation in its solid state, and might be from the change of the excited structure.7a The data above implies that the inserted cyano-group scarcely influenced the electronic properties and optical energy gap, and it is in agreement with the results of quantum chemical calculations.
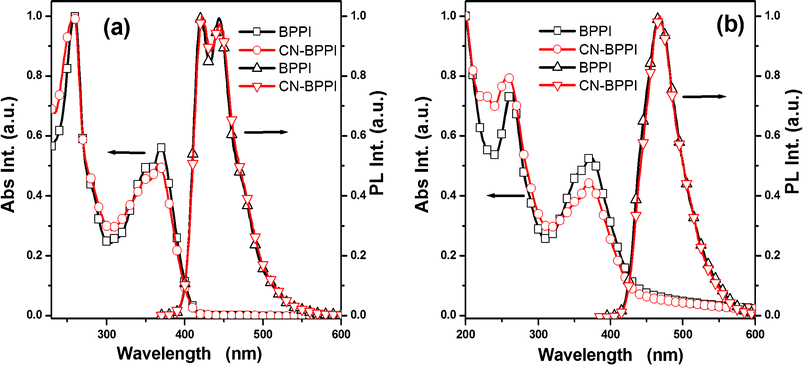 |
| Fig. 4 Normalized absorption and emission spectra of BPPI and CN-BPPIin THF (10−5 M) (a) and in film (b). | |
Concerning the fluorescence quantum yields of CN-BPPI, they exhibit nearly 100% efficiency in solution and solid, and both are higher than BPPI (93% in solution and 85% in solid). The improved quantum yield is attributed to the decreased proportion of the non radiative transition because of the supermolecular interactions, such as the formation of intermolecular C–N⋯H or C–N⋯π between the cyano-group and the phenanthrene plane.13 It is a rare model for whic the PL spectra are unchanged and the yields are increased by inserting an electron-withdrawing group.
4.6 CV measurement
The CV measurement in solution was conducted in CH2Cl2 and DMF 1
:
1 with 0.1 M tetra-n-butylammoniumhexafluorophosphate (n-Bu4NPF6) as the electrolyte at room temperature. HOMO and LUMO levels were estimated using the energy level of the ferrocene (Fc) reference (4.8 eV) and calibrated with E1/2(Fc/Fc+) = 0.22 V.3b The cyclic voltammograms are shown in Fig. 5 and the respective electrochemical data are summarized in Table 1.
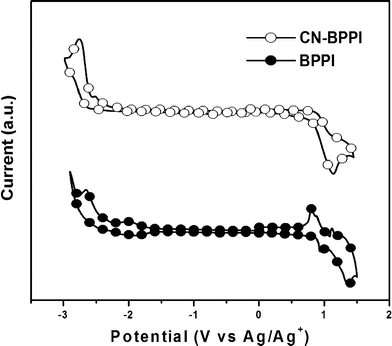 |
| Fig. 5 The CV curves of BPPI and CN-BPPI. | |
Table 1 The thermal, photophysical and electrochemical data for BPPI and CN-BPPI
|
T
g/°C |
T
d
/°C |
PL λmaxb/nm (soln) |
PL λmax/nm (film) |
Φflc (soln/film) |
HOMOd/eV |
LUMO/eV |
E
gap/eV |
The temperature for 5% weight loss of the oligomers.
The main peak except the peripheral phenyl group attached to nitro atom.
The fluorescence quantum yield in solution compared to 0.5 M H2SO4 solution of quinine as a reference (0.54) and the solid state quantum yield (0.22) on the quartz plate by an integrating sphere apparatus.
The energy levels were calculated using the energy level of the ferrocene (Fc) reference (4.8 eV) and calibrated using E1/2 (Fc/Fc+) = 0.22 V.
|
BPPI
|
195 |
535 |
421 |
468 |
0.92/0.85 |
−5.47 |
−2.29 |
3.18 |
CN-BPPI
|
217 |
535 |
421 |
468 |
0.99/0.99 |
−5.59 |
−2.46 |
3.13 |
CN-BPPI exhibited similar re-dox progress to BPPI, but the onset voltages were changed obviously. The onset oxidation potential (Eonsetox) of CN-BPPI was at 0.98 V vs. Ag wire, and the HOMO level was measured to be −5.59 eV, which is a slight positive shift from that of BPPI (−5.47 eV). This shift indicated a decreased hole injection ability. In the negative potential region, the onset reduction potential (Eonsetred) at −2.15 V vs. Ag wire was observed for CN-BPPI, and the LUMO level was calculated to be −2.46 V. This value even exceeds that of TPBi (LUMO level of −2.40 eV), revealing that the electron injection ability of CN-BPPI is similar to or better than TPBi. Compared with BPPI, the hole injection and transport ability of CN-BPPI was limited and the electron ability was enhanced, which reflected the more balanced carrier injection properties.
4.7 Single-carrier device
For better understanding of the effect of the structural change on the energy level and the carrier injection and transport ability, hole-only and the electron-only devices of BPPI and CN-BPPI were fabricated.15 The configuration of the hole-only device was ITO/BPPI or CN-BPPI (90 nm)/Au (100 nm) and the electron-only device had the configuration of ITO/TPBi (10 nm)/BPPI or CN-BPPI (90 nm)/LiF (1 nm)/Al (100 nm). In the electron-only device, the 10 nm TPBi was inserted as a hole-blocking layer to ensure a pure electron current in the device. It can be seen from Fig. 6 that the hole-current density (J) in CN-BPPI was lower than in BPPI. It indicated that the inserted cyano-group limited the holes injection and transport to some extent.8,13 The same tendency of the electron current to change was observed in both devices when the electric field intensity was increased, but a very closed electron current and hole current density in the CN-BPPI device in the low electric field intensity zone was observed, which is suitable for operation in lower voltages for high performance OLEDs. This provides strong evidence that CN-BPPI possesses more balanced charge injection-transport, and it is consistent with the results from CV measurements.
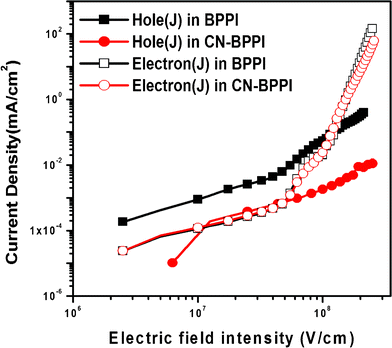 |
| Fig. 6 The current density–electric field intensity curves of the single-carrier devices based on BPPI and CN-BPPI. | |
4.8 Morphology
BPPI and CN-BPPI could both form continuous amorphous films fabricated by vacuum deposition exhibiting fairly smooth surface morphologies with roughness of 0.36 and 0.43 nm, respectively. For comparing the effect of the cyano unit, the films were annealed at 180 °C for 8 h, which is lower than the Tg of BPPI, and both films showed a smooth and featureless morphology (see Fig. 7) with a surface root mean square roughness less than 0.4 nm, except for a weak crystallization in BPPI, but this operated temperature is rare in device fabrication. This result implied that the two compounds, and especially CN-BPPI, were suitably thermally stable for application in OLEDs.
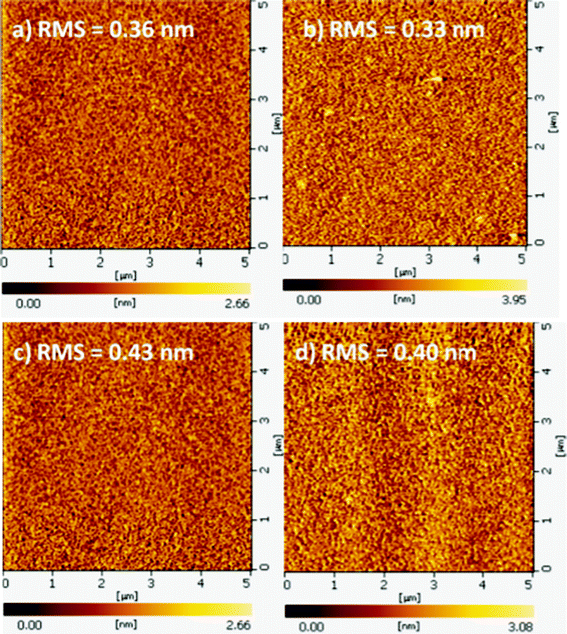 |
| Fig. 7
BPPI and CN-BPPI film on the quartz plate at room temperature (a: BPPI, c: CN-BPPI) and after annealing at 180 °C for 8 h (b: BPPI, d: CN-BPPI). | |
4.9 EL properties
To investigate the EL properties, a simple device structure was fabricated with ITO/NPB (60 nm)/BPPI or CN-BPPI (60 nm)/LiF (1 nm)/Al (100 nm) as shown in Fig. 8a, in which NPB was utilized as a hole-transporting layer and a smooth layer for the irregular ITO surface. Similar to BPPI, the device of CN-BPPI exhibited blue emission peaked around 468 nm, as shown in Fig. 8b. The turn-on voltage of CN-BPPI (5.6 V) was higher than BPPI (4.0 V) in the same measurement, which might originate from the higher hole-injection barrier from the analysis of their hole-only devices.
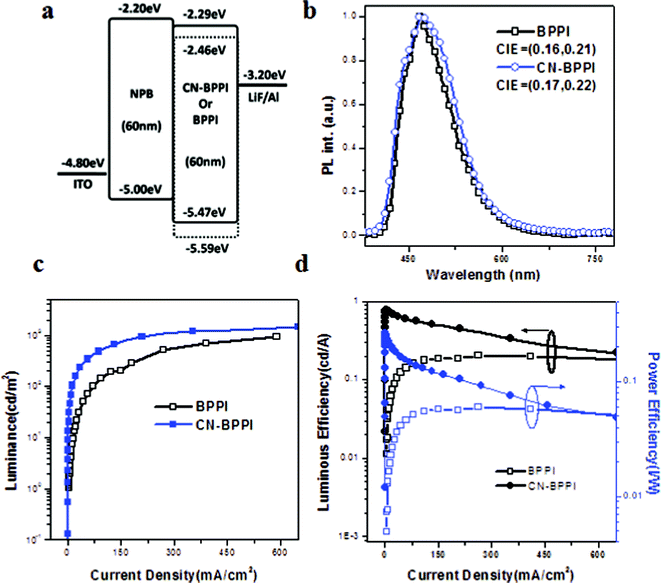 |
| Fig. 8 (a) The EL devices’ structure. (b) The EL spectra of the devices at 8 V. (c) The luminance–current density curves of the devices. (d) The current efficiency and power efficiency versus current density curves of the devices. | |
At the same current density, the CN-BPPI device showed better performance with a maximum luminance of 1400 cd m−2, a maximum current efficiency of 0.78 cd A−1 and a maximum luminance efficiency of 0.26 lm W−1 (shown in Fig. 8c,d and Table 2), which should be due to the more balanced proportion of holes to electrons. It was proved that CN-BPPI could depress excessive holes to transport in the emission-layer and could realize the tuning of carrier mobilities. For the same luminance, the current density (J) in the CN-BPPI device was lower than BPPI, for example it was one fourth at 100 cd m−2. Such lower values could decrease heat effects, and it is very important to extend the service life of photoelectronic devices. The efficiency decreased quickly with increasing J , which was because of the loss of the carrier transport balance. At lower current density, the hole and electron current in CN-BPPI were nearly at the same level, while the electron transport ability increased more quickly than the hole ransport ability accompanied with the J(E). At higher current density, the BPPI shows more stability in EL performance. As we know, it is more meaningful that a device shows high performance at lower current density for OLEDs application, and so BPPI could be applied in some special devices worked at high current density.
Table 2 The device performances of BPPI and CN-BPPI
Device |
CIE (x, y) |
Voltagea/V |
LEMax/cd A−1 |
PEMax/lm W−1 |
LMax/cd m−2 |
Device performance at 100 cd m−2 |
Current density/mA cm−2 |
LE/cd A−1 |
PE/lm W−1 |
The turn-on voltage (L > 1 cd m−2).
|
BPPI
|
0.16, 0.21 |
4 |
0.22 |
0.05 |
1000 |
72.3 |
0.16 |
0.05 |
CN-BPPI
|
0.17, 0.22 |
5.6 |
0.78 |
0.26 |
1450 |
14.3 |
0.73 |
0.21 |
5. Conclusion
In summary, we have developed a new compound (CN-BPPI) for efficient blue light emitting materials with relatively balanced carrier injection and transport ability. Compared with the model compound BPPI, the cyano-group inserted did not influence the blue emission, but the material showed comprehensive enhancement in basic characteristics involving purification costs, thermal stability, fluorescent efficiency, and charge injection properties. The device shows higher device performance at lower current density than BPPI, rendering prospects in actual applications. The work also demonstrates a strategy of chemical modification to improve materials' properties.
Acknowledgements
We are grateful for support from the National Science Foundation of China (Grant No. 20704016, 20834006, 51203091), the Ministry of Education of China (Grant No.20070183202), the Ministry of Science and Technology of China (Grant No. 2009CB623605) and PCSIRT.
References
-
(a) C.W. Tang and S. A. VanSlyke, Appl. Phys. Lett., 1987, 51, 93 Search PubMed;
(b) J. H. Burroughes, D. D. C. Bradley, A. R. Brown, R. N. Marks, K. Mackay, R. H. Friend, P. L. Born and A. B. Holmes, Nature, 1990, 347, 539 CrossRef CAS.
-
(a) S. Chichibu, T. Azuhata, T. Sota and S. Nakamura, Appl. Phys. Lett., 1997, 70, 2822 CrossRef CAS;
(b) T. Noda, H. Ogawa and Y. Shirota, Adv. Mater., 1999, 11, 283 CrossRef CAS.
-
(a) K. Kreger, M. Baete, C. Neuber, H. W. Schmidt and P. Strohriegl, Adv. Funct. Mater., 2007, 17, 3456 CrossRef CAS;
(b) S. Tang, M. R. Liu, P. Lu, H. Xia, M. Li, Z. Q. Xie, F. Z. Shen, C. Gu, H. Wang, B. Yang and Y. G. Ma, Adv. Funct. Mater., 2007, 17, 2869 CrossRef CAS;
(c) Y. Q. Mo, R. Y. Tian, W. Shi and Y. Cao, Chem. Commun., 2005,(39), 4925 RSC.
-
(a) Z. Jiang, Z. Liu, C. Yang, C. Zhong, J. Qin, G. Yu and Y. Q. Liu, Adv. Funct. Mater., 2009, 19, 1 Search PubMed;
(b) M. S. Gong, H. S. Lee and Y. M. Jeon, J. Mater. Chem., 2010, 20, 10735 RSC.
-
(a) A. P. Kulkarni, C. J. Tonzola, A. Babel and S. A. Jenekhe, Chem. Mater., 2004, 16, 4556 CrossRef CAS;
(b) P. Strohrieg and J. V. Grazulevicius, Adv. Mater., 2002, 14, 1439 CrossRef.
-
(a) S. H. Lin, F. I. Wu and R. S. Liu, Chem. Commun., 2009,(45), 6961 RSC;
(b) Y. Shirota, M. Kinoshita, T. Noda, K. Okumoto and T. Ohara, J. Am. Chem. Soc., 2000, 122, 11021 CrossRef CAS.
-
(a) C. J. Kuo, T. Y. Li, C. C. Lien, C. H. Liu, F. I. Wu and M. J. Huang, J. Mater. Chem., 2009, 19, 1865 RSC;
(b)
C. H. Cheng, C. H. Liu and F. I. Wu, U.S. Patent No. 20100253208 2010 Search PubMed.
- Y. Yuan, D. Li, X. Q. Zhang, X. J. Zhao, Y. Liu, J. Y. Zhang and Y. Wang, New J. Chem., 2011, 35, 1534 RSC.
-
(a) Y. Zhang, S. L. Lai, Q. X. Tong, M. Y. Chan, T-W Ng, Z. C. Wen, G. Q. Zhang, S. T. Lee, H. L. Kwong and C. S. Lee, J. Mater. Chem., 2011, 21, 8206 RSC;
(b) Y. Zhang, S. L. Lai, Q. X. Tong, M. F. Lo, T. W. Ng, M. Y Chan, Z. C. Wen, J. He, K. S. Jeff, X. L. Tang, W. M. Liu, C. C. Ko, P. F. Wang and C. S. Lee, Chem. Mater., 2012, 24, 61 CrossRef.
-
(a) Z. M. Wang, P. Lu, S. M. Chen, Z. Gao, F. Z. Shen, W. S. Zhang, Y. X. Xu, H. S. Kwok and Y. G. Ma, J. Mater. Chem., 2011, 21, 5451 RSC;
(b) Z. M. Wang, Z. Gao, S. F. Xue, Y. L. Liu, W. S. Zhang, C. Gu, F. Z. Shen, P. Lu and Y. G. Ma, Polym. Bull., 2012, 69, 273 CrossRef CAS;
(c) W. J. Li, D. D Liu, F. Z. Shen, D. G Ma, Z. M. Wang, T. Feng, Y. X. Xu, B. Yang and Y. G. Ma, Adv. Funct. Mater., 2012, 22, 2797 CrossRef CAS.
-
(a) W. Z. Yuan, S. M. Chen, J. W. Y. Lam, C. M. Deng, P. Lu, H. M. H. Sung, I. D. Williams, H. S. Kwok, Y. M. Zhang and B. Z. Tang, Chem. Commun., 2011, 47, 11216 RSC;
(b) X. J. Xu, S. Y. Chen, G. Yu, C. A. Di, H. You, D. G. Ma and Y. Q. Liu, Adv. Mater., 2007, 19, 1281 CrossRef CAS.
-
(a) Y. T. Chang, S. L. Hsu, G. Y. Chen, M. H. Su, T. A. Singh, E. W. G. Diau and K. H. Wei, Adv. Funct. Mater., 2008, 18, 1 CrossRef;
(b) Y. T. Chang, S. L. Hsu, M. H. Su and T. K. H. Wei, Adv. Funct. Mater., 2007, 17, 3326 CrossRef CAS.
-
(a) Y. P. Li, F. Li, H. Y. Zhang, Z. Q. Xie, W. J. Xie, H. Xu, B. Li, F. Z. Shen, L. Ye, M. Hanif, D. G. Ma and Y. G Ma, Chem. Commun., 2007,(3), 231 RSC;
(b) Y. P Li, F. Z Shen, H. Wang, F. He, Z. Q. Xie, H. Y Zhang, Z. M. Wang, L. L. Liu, F. Li, M. Hanif, L. Ye and Y. G. Ma, Chem. Mater., 2008, 20, 7312 CrossRef.
- C. H. Chien, C. K. Chen, F. M. Hsu, C. F. Shu, P. T. Chou and C. H. Lai, Adv. Funct. Mater., 2009, 19, 1 CrossRef.
- C. H. Chien, F. M. Hsu, C. F. Shua and Y. Chi, Org. Electron., 2009, 10, 871 CrossRef CAS.
Footnote |
† Experimental: preparation of BPPI and CN-BPPI.The synthetic route of BPPI is in our previous report, which is similar to that of CN-BPPI. A mixture of phenanthrene-9,10-dione (2.08 g, 10 mmol), 4-aminobenzonitrile (3.57 g, 30 mmol), ammonium acetate (3.85 g, 50 mmol) and 4,4′-biphenyldicarboxaldehyde (1.01 g, 4.8 mmol) were refluxed in acetic acid for 2 h, then poured into a methanol solution with stirring. After filtering, the BPPI was purified by recrystallization from DMF, and light-yellow crystals were gained (3.16 g, Yield: 83.3%). 1H NMR (500 MHz, DMSO, ppm): 8.92 (d, J = 8.5 Hz, 2H), 8.88 (d, J = 8.5 Hz, 2H), 8.70 (d, J = 7.9 Hz, 2H), 8.23 (d, J = 8.2 Hz, 4H), 8.08 (d, J = 8.2 Hz, 4H), 7.80 (t, J = 7.6 Hz, 7.0 Hz, 2H), 7.76 (d, J = 8.2 Hz, 4H), 7.72 (t, J = 7.6 Hz, 7.2 Hz, 2H), 7.63 (d, J = 8.2 Hz, 4H), 7.57 (t, J = 7.9 Hz, 7.6 Hz, 2H), 7.34 (t, J = 7.6 Hz, 7.6 Hz, 2H), 7.08 (d, J = 7.9 Hz, 2H). MALDI-TOF (m/z): [M+] calcd. C56H32N6, 788.28; found, 788.9. Anal. Calc. for C56H32N6: C, 85.26; H, 4.09; N, 10.65. Found: C, 85.10; H, 4.08; N, 10.66. |
|
This journal is © The Royal Society of Chemistry 2012 |