DOI:
10.1039/C2RA20803B
(Paper)
RSC Adv., 2012,
2, 9614-9618
Porous Mn3[Co(CN)6]2·nH2O nanocubes as a rapid organic dyes adsorption material†
Received
28th April 2012
, Accepted 10th August 2012
First published on 14th August 2012
Abstract
Porous Mn3[Co(CN)6]2·nH2O nanocubes were successfully synthesized by a surfactant-assisted (PVP) process through a simple precipitation method at room temperature. X-Ray powder diffraction (XRD) and field emission scanning electron microscopy (FE-SEM) were employed for the morphology and structure characterization. The fine-detailed information about porous structures of the samples has also been studied using the Brunauer–Emmet–Teller (BET) isotherm. Most importantly, the prepared porous structured Mn3[Co(CN)6]2·nH2O showed excellent adsorption capacity and rapid adsorption rate for dyes in aqueous solution, such as methyl violet, neutral red, methyl orange and especially for methylene blue. The effects of contact time, initial concentration and initial pH were also investigated. The maximum adsorption capacity of methylene blue was as high as 489.9 mg g−1 and 96.5% of the dye was removed within initial 5 min of contact time. Due to the simple synthetic method, fast adsorption rate and its high adsorption capacity, the synthesized Mn3[Co(CN)6]2·nH2O porous nanocubes could be used as an effective adsorbent for removing dye pollutants from waste water.
1. Introduction
Dyes are widely used in leather, paper, rubber, textile industries, etc.1,2 With the development of technology and industry, more and more attention has been paid to water pollution which contains dyes, such as methyl violet, neutral red and methylene blue. The discharge of this waste water into natural streams has caused many significant problems such as carcinogenicity and toxicity to aquatic life and is aesthetically objectionable, that can seriously threaten the environment and health of human beings.3–5 To meet tougher colour standards and reduce the harm to humans, it is imperative for industries to decolorizate and degrade the dyes in their wastes to an acceptable level before discharging into the environment. Nowadays, many methods have been designed to treat contaminated water before drainage, such as precipitation, flocculation, membrane filtration, ion-exchange, reverse osmosis and electrochemical techniques.6–9 These processes have their disadvantages and limitations, such as high cost, generation of secondary pollutants and poor removal efficiency. Thus adsorption has been found to be an efficient and economical process used for the removal of dyes from wastewater which has involved the use of various solid adsorbents. Activated carbon is the most effective and widely used adsorbent, 10 due to its vast surface area and affinity for many organic chemicals. However, activated carbon is costly to regenerate and both regeneration and disposal of the used carbon are often very difficult. Consequently, lots of low cost adsorbents are reported in the literature, which include fly ash,11 peat,12 wasted activated sludge,13 kapok fiber,14 cobalt–hectorite composite,15 cotton,16 natural zeolite/base.17 Nevertheless, sorption potential of most of these low cost sorbents is generally low. Searching for inexpensive and high efficiency materials as adsorbents for dyes removal therefore seems to be more and more important.
In recent years, shape-controlled synthesis of nanomaterials has been an interesting research topic for its interesting physical and chemical properties.18–26 Due to the large surface area and porous structure, synthesized nanomaterials such as some metal oxides and metal coordination compounds, nanoparticles could absorb dyes and showed high adsorption capacities, which imply potential application for removing dye pollutants from waste water.27 These have been extensively studied for the removal of toxic ions and organic pollutants from water.28 Nanocrystalline metal oxides such as MgO, CaO showed higher capacities for typical volatile organic compounds compared to high surface area activated carbon.29 Magnetic Fe3O4 nanoparticles were studied as efficient absorbents for removal of an acid dye (new coccine) from aqueous solutions.30
In all these years, there exits considerable interests in Prussian Blues (PBs) or their analogue due to the particular physical properties, including electronic, optical, and magnetic properties.31–38 The gas sorption properties and removal of heavy metals from waste water of PB coordination compounds were only investigated very recently.39–44 Recently, Chen's group have synthesized Mn3[Co(CN)6]2·nH2O porous nanocubes at room temperature by simple mixing of reactants.39,41 N2 adsorption showed that Mn3[Co(CN)6]2·nH2O uniform porous nanocubes possessed high surface area and bimodal size distribution of two kinds of pores. More importantly, they have successfully investigated Mn3[Co(CN)6]2·nH2O porous nanocubes' adsorption capacities and adsorption efficiency for heavy metal ions and CO2 in various conditions. In this work, porous Mn3[Co(CN)6]2·nH2O nanocubes have been synthesized by modifying Chen's method.39,41 The aim of the present work is to explore the possibility of utilizing Mn3[Co(CN)6]2·nH2O for the adsorptive removal of dyes from aqueous solutions. The effect of contact time, initial concentration and solution pH was investigated. The prepared Mn3[Co(CN)6]2·nH2O porous nanocubes showed excellent adsorption capacity and rapid adsorption rate for dyes in water.
2. Materials and methods
2.1 Materials
The adsorbate methyl violet (MV), neutral red (NR), methyl orange (MO) and methylene blue (MB) were purchased from Kermel Chemical Reagent Co., Ltd (Tianjin, China) and used as received without further purification. Accurately weighed quantities of methyl violet, neutral red, methyl orange and methylene blue were respectively dissolved in deionized water to prepare stock solutions (1000 mg L−1). The desired concentrations of solutions in further experiments were obtained by successive dilutions. Hydrochloric acid and sodium hydroxide solutions used for initial pH adjustment were prepared from analytical grade chemicals. Potassium cobalticyanide (K3[Co(CN)6]2), poly(vinylpyrrolidone) (PVP), manganous nitrate 50% solution and all other chemicals were analytical chemicals.
2.2 Preparation
Mn3[Co(CN)6]2·nH2O have been synthesized by a modified L. Hu et al. method,39,41 the product was named P (see ESI† for preparation).
2.3 Characterization
To study the phase structure of the samples, X-ray diffraction (XRD) patterns were recorded by a D8 diffractometer in the 2θ range of 10–70° using a Ultima III X-ray diffractometer with Cu-Ka radiation (λ = 0.1541 nm). Nitrogen adsorption–desorption measurements were performed on a Gemini VII 2390 Analyzer at 77 K using the volumetric method. The specific surface area was obtained from the N2 adsorption–desorption isotherms and was calculated by the Brunauer–Emmett–Teller (BET) method. The pore size distributions were estimated from adsorption branches of the isotherms by the Barrett, Joyner, and Halenda (BJH) method. The morphologies and microstructures of the samples were studied by a JEOL JSM-6701F field emission scanning electron microscopy (FESEM) operating at 5 KV in high vacuum.
To examine the ability of P to remove MB from an aqueous solution, the adsorption of MB from an aqueous solution was performed. After adsorption, the solution was centrifuged for 2 min with rotate speed of 1000 rpm and then the concentration of remaining MB was analyzed by UV-VIS spectrophotometer (Shimadzu, UV-3000) at 670 nm. Each experimental run continued until no significant change in the dye concentration was measured. All experiments were carried out in triplicate. Calibration curves were plotted between absorbance and concentration of the dye solution. The removal efficiency (%), the amount of MB adsorbed at time t (qt, mg g−1) was calculated by using the following equations, respectively: | 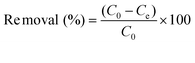 | (1) |
| 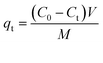 | (2) |
Where C0, Ct and Ce (mg L−1) are the initial, time and equilibrium concentrations of the MB solution, respectively; V (L) is the volume of MB solution and M (g) is the weight of P. The relationship between eqn (1) and eqn (2) can be seen in ESI calculations.†
Conditions: P: 0.1 g; MB: 50 mL, 50–1000 mg L−1; temperature: 16 °C; contact time: 5–300 min.
3. Results and discussion
3.1 Characterization of P
The phase purity and crystal structure of the products obtained were examined by XRD. Fig. 1 shows the X-ray diffraction patterns of the as-prepared products, which are both in good agreement with the standard values for bulk cubic Mn3[Co(CN)6]2·nH2O (JCPDS No.89-3735).
![XRD patterns of as-prepared Mn3[Co(CN)6]2·nH2O.](/image/article/2012/RA/c2ra20803b/c2ra20803b-f1.gif) |
| Fig. 1 XRD patterns of as-prepared Mn3[Co(CN)6]2·nH2O. | |
SEM images of Mn3[Co(CN)6]2·nH2O are shown in Fig. 2. In Fig. 2a, uniform Mn3[Co(CN)6]2·nH2O nanocubes have been successfully synthesized. From the images in Fig. 2b, it could easily be seen that the Mn3[Co(CN)6]2·nH2O nanocube is a corner-truncated cubic structure with a length of 100 nm.
![SEM images of as-prepared Mn3[Co(CN)6]2·nH2O.](/image/article/2012/RA/c2ra20803b/c2ra20803b-f2.gif) |
| Fig. 2 SEM images of as-prepared Mn3[Co(CN)6]2·nH2O. | |
To gain further insight into the porous structure of the samples, Brunauer–Emmett–Teller (BET) measurements were performed to examine their specific structural properties. Fig. 3 shows the nitrogen adsorption–desorption isotherms and the corresponding BJH pore size distribution curves of the obtained P. The adsorption–desorption isotherms display a type IV nitrogen adsorption isotherm typical of a mesoporous material suggesting a potentially useful mesoporous material. The mesopore size distributions were calculated by the BJH method, assuming distributions in the range of 2–5 nm. The high BET surface area is calculated to be 473.99 m2 g−1, which is beneficial for providing more active sites for dye adsorption.
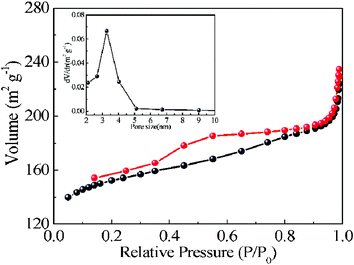 |
| Fig. 3 Nitrogen adsorption–desorption isotherms and pore size distribution (inset) of P. | |
3.2 Adsorption experiment studies
3.2.1 Effect of contact and equilibrium times.
In order to determine the adsorption equilibrium time, the adsorption of the MB dye onto P was studied as a function of contact time. The contact time between the adsorbate and absorbent is one of the most important parameters in the waste water treatment by adsorption. A rapid uptake of absorbates, and the establishment of equilibrium in a short period, stress the efficiency of the adsorbent for its use in wastewater treatment.
Fig. 4 shows the effects of contact time on the amount of MB adsorbed by P under different initial MB concentrations. The necessary contact time to reach the equilibrium depends on the initial dye concentration and the adsorption capacity increases with the initial dye concentration in all cases. The adsorption rate was very high for the first 5 min in Fig. 4a and thereafter it became slower near the equilibrium, finally equilibrium was established after about 60 min. The rapid adsorption observed during the first 5 min can be attributed to the abundant availability of active sites on the P surface, and with the gradual occupancy of these sites, the adsorption became less efficient in Fig. 4b. It was seen that an increase in initial dye concentration resulted in increased dye uptake. The adsorption equilibrium time increased from 5 min for 50 mg g−1 to 60 min for 200 mg g−1, and the amount of MB adsorbed at equilibrium increased from 24.68 mg g−1 for 50 mg L−1 to 99.21 mg g−1 for 200 mg L−1. The increase in the amount of MB absorbed is mainly due to a greater driving force, resulting from a greater concentration gradient between MB in solution and MB on the adsorbent surface at higher initial dye concentration, besides the increase in initial dye concentration also enhances the interaction between MB and P.
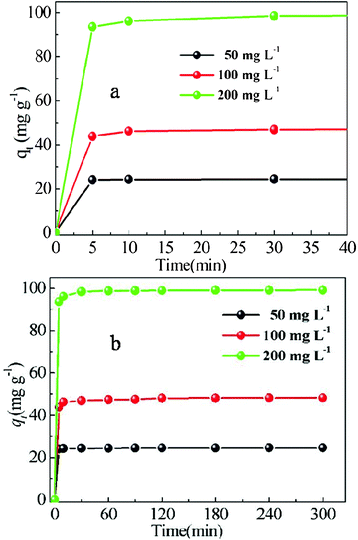 |
| Fig. 4 Adsorption of MB on P as a function of contact time. Conditions: P: 0.1 g; MB: 50 mL, 50–200 mg L−1; temperature: 16 °C; contact time: 5–300 min. | |
After the adsorption of MB, we also have tested the XRD pattern and IR of porous Mn3[Co(CN)6]2·nH2O nanocubes (see ESI Fig. 1,2†). From these data, we can seen that the structural framework of Mn3[Co(CN)6]2·nH2O is stable after dye-adsorption in solution.
3.2.2 Effect of initial MB concentration.
The relationship between initial concentrations and adsorption efficiency was studied with initial MB concentrations ranging from 50 to 1000 mg L−1 and 100 mg P at 16 °C for 5 h. Fig. 5 shows the sorption columns of MB on P with an initial concentration range of 50–1000 mg L−1, from which we can see that P exhibits an excellent adsorption efficiency for MB at various initial concentrations. When the concentration of MB ranges from 50 mg L−1 to 1000 mg L−1, more than 95% of MB could be removed. Meanwhile, the adsorption capacity is listed in Table 1, and it was clear that the adsorption capacity of the dye depended on the concentration of the dye. Especially, when the concentration of MB has been increased to 1000 mg L−1, the adsorption capacity of MB can reach 489.9 mg g−1. What's more, we have also changed the amounts of P used in the experimental section and found that the removal % value is up to 99.9%, when we used 0.12 g of P. The removal % is 28%, when we only used 1 mg of P (see ESI Fig. 3†). The initial dye concentration provided the necessary driving force to overcome the resistances to the mass transfer of MB between the aqueous and solid phases. Therefore, an increase in initial concentration of MB enhances the adsorption uptake of MB. If compared to other nanoscale absorbents, such as polyacrylic acid-bound iron oxide magnetic nanoparticles (199.0 mg g−1),45 and titanate nanotubes (133.3 mg g−1),46 the nanoparticles prepared in this work have a relatively larger adsorption capacity of 489.9 mg g−1.
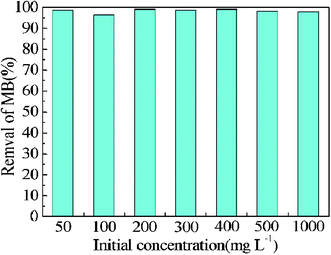 |
| Fig. 5 The influence of initial concentrations on removal efficiency (%) of MB on P. Conditions: P: 0.1 g; MB: 50 mL, 50–1,000 mg L−1; temperature: 16 °C; contact time: 5 h. | |
Table 1 Adsorption capacities and efficiency of P under different initial MB concentrations
Initial concentration (mg L−1) |
50 |
100 |
200 |
300 |
400 |
500 |
1000 |
q
t (mg g−1) |
24.7 |
48.2 |
99.2 |
148.2 |
198.3 |
245.7 |
489.9 |
3.2.3 Effect of initial solution pH.
In the adsorption process, the pH of the aqueous solution is also an important parameter. An ideal absorbent could be extensively used in a wide pH range. In order to evaluate the relationship between the pH value of the solution and the adsorption capacity of P, experiments at different pH ranging from 2 to 11 were carried out with 20 mL of 100 mg L−1 MB initial concentration and 20 mg absorbent at 16 °C for 5 h. The pH was adjusted using 0.1 M HCl and 0.1 M NaOH solutions. Fig. 6 shows the influence of pH on the adsorption of MB. As the pH increased from 2 to 11, there was an increase in the adsorption capacity from 86.87 mg g−1 to 94.94 mg g−1, which indicated that the removal of MB by P fluctuated slightly in the pH range 2–11. The pKa of MB is 9.6, which might cause the increasing adsorption of MB in pH 8–11. This may give more opportunities to use P in further applications.
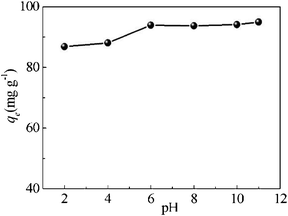 |
| Fig. 6 Adsorption of MB on P as a function of pH. Conditions: P: 20 mg; MB: 20 mL, 100 mg L−1; temperature: 16 °C; contact time: 5 h. | |
3.2.4 Removal of other dyes from aqueous solution.
An ideal absorbent could be extensively able to adsorb different kinds of dyes. To determine the adsorption capacities and efficiency for other dyes on P, experiments with different kinds of dyes including MV, NR and MO was carried out with 50 mL of 100 mg L−1 dyes initial concentration and 0.1 g adsorbent at 16 °C for 5 h. MO, MV and NV are cation type dyes, while MB is anion type dye (see ESI Fig. 4†). Adsorption capacities and removal efficiency of the adsorbents for other dyes are listed in Table 2, from which we can see that the removal efficiency of MV, NR and MO is 82.60%, 95.79% and 80.20%, respectively. Moreover, the adsorption capacity of MV, NR and MO on P can be up to above 40 mg g−1. This result shows that P exhibited good adsorption capacity and high removal efficiency for other dyes, especially for anion dye MB.
Table 2 Adsorption capacities and removal efficiency of the adsorbent for other dyes
— |
Initial concentration (mg L−1) |
q
t (mg g−1) |
Removal efficiency (%) |
MV |
100 |
41.3 |
82.60 |
NR |
100 |
47.9 |
95.79 |
MO |
100 |
40.1 |
80.20 |
3.3 Others
For comparison, we have successfully synthesized Fe(II)/Fe(III) Prussian Blue nanostructures (see ESI Fig. 5†), which have been used for the adsorption of MB under the same conditions as that of porous Mn3[Co(CN)6]2·nH2O nanocubes. Fe(II)/Fe(III) Prussian Blue can be used for the adsorption of MB and its removal % is much lower than that of porous Mn3[Co(CN)6]2·nH2O nanocubes under our experimental conditions (F1-83.4%; F2-76.5%; P-98.8% for MB: 50 mL, 50 mg L−1; temperature: 16 °C; contact time: 5 h).
More importantly, we found different morphologies of Mn3[Co(CN)6]2·nH2O have different BET areas with different capacities of adsorpting MB (see ESI Fig. 6 and Table 1†). It is clear that formless Mn3[Co(CN)6]2·nH2O nanoparticles have a smaller BET area than Mn3[Co(CN)6]2·nH2O nanocubes, which might cause the low capacities of the adsorption of MB. Compared with activated carbon in this system, we have also found that the capacity of MB for porous Mn3[Co(CN)6]2·nH2O nanocubes was larger than that of activated carbon, but for MO, MV and NV, activated carbon had a larger capacity (see ESI Table 2†), which might be caused by the structure of the MB anion dye type.
4. Conclusions
In summary, Mn3[Co(CN)6]2·nH2O porous nanocubes with a large surface area have been successfully obtained at room temperature through a simple precipitation method in the presence of poly(vinylpyrrolidone) (PVP) and had been characterized by XRD, FE-SEM and BET techniques. Adsorption of MB dye on Mn3[Co(CN)6]2·nH2O can be considered as a simple, fast and economic method for its removal from water and wastewater. The effects of equilibrium time, initial concentrations and pH value have been studied, which suggest that the as-prepared products show excellent adsorption capacities at a wide pH range and high concentration of dyes, moreover, the adsorption rate for the first 5 min is very high and finally an equilibrium was established after about 60 min. Meanwhile, Mn3[Co(CN)6]2·nH2O exhibited a good adsorption capacity and high removal efficiency for other dyes. Based on these results, Mn3[Co(CN)6]2·nH2O are recommended as effective adsorbents for the removal of dyes from wastewater.
References
- M. S. Chiou and H. Y. Li, Chemosphere, 2003, 50, 1095–1105 CrossRef CAS
.
- B. H. Hameed and A. A. Ahamad, J. Hazard. Mater., 2009, 164, 870–875 CrossRef CAS
.
- Z. Aksu and S. Tezer, Process Biochem., 2001, 36, 431–439 CrossRef
.
- B. Royer, N. F. Cardodo, E. C. Lima, T. R. Macedo and C. Airoldi, Sep. Sci. Technol., 2010, 45, 129–141 CrossRef CAS
.
- G. R. Xu, L. Y. Deng and G. B. Li, Water Sci. Technol., 2010, 62, 1705–1712 CrossRef
.
- E. Lorenc-Grabowska and G. Gryglewicz, Dyes Pigm., 2007, 74, 34–40 CrossRef CAS
.
- P. K. Malik and S. K. Saha, Sep. Purif. Technol., 2003, 31, 241–250 CrossRef CAS
.
- P. K. Malik and S. K. Sanyal, Sep. Purif. Technol., 2004, 36, 167–175 CrossRef CAS
.
- I. M. Banat, P. Nigam, D. Singh and R. Marchant, Bioresour. Technol., 1996, 58, 217–227 CrossRef CAS
.
- M. S. El-Geundi, Water Res., 1991, 25, 271–273 CrossRef CAS
.
- V. K. Gupta, D. Mohan, S. Sharma and M. Sharma, Sep. Sci. Technol., 2000, 35, 2097–2113 CrossRef CAS
.
- K. R. Ramakrishna and T. Viraraghavan, Am. Dyestuff Rep., 1996, 85, 28–34 CAS
.
- G. Annadurai, R. S. Juang, P. S. Yen and D. J. Lee, Adv. Environ. Res., 2003, 7, 739–744 CrossRef CAS
.
- Y. Liu, J. T. Wang, Y. A. Zheng and A. Q. Wang, Chem. Eng. J., 2012, 184, 248–255 CrossRef CAS
.
- J. Ma, Y. Z. Jia, Y. Jing, Y. Yao and J. H. Sun, Dyes Pigm., 2012, 93, 1441–1446 CrossRef CAS
.
- K. Sawada and M. Ueda, Dyes Pigm., 2003, 58, 37–40 CrossRef CAS
.
- D. Y. Yue, Y. Jing, J. Ma, C. L. Xia, X. J. Yin and Y. Z. Jia, Desalination, 2011, 56, 219–229 Search PubMed
.
- H. Pang, Q. Y. Lu, Y. Z. Zhang, Y. C. Li and F. Gao, Nanoscale, 2010, 2, 920–922 RSC
.
- C. Burda, X. Chen, R. Narayanan and M. A. El-Sayed, Chem. Rev., 2005, 105, 1025–1102 CrossRef CAS
.
- K. B. Zhou, X. Wang, X. M. Sun, Q. Peng and Y. D. Li, J. Catal., 2005, 229, 206–212 CrossRef CAS
.
- H. Pang, J. W. Deng, J. M. Du, S. J. Li, J. Li, Y. H. Ma, J. S. Zhang and J. Chen, Dalton Trans., 2012, 41, 10175 RSC
.
- H. Pang, Q. Y. Lu, Y. C. Li and F. Gao, Chem. Commun., 2009, 7542–7544 RSC
.
- H. Pang, B. Zhang, J. Du, J. Chen, J. S. Zhang and S. Li, RSC Adv., 2012, 2, 2257–2261 RSC
.
- H. Pang, F. Gao and Q. Y. Lu, Chem. Commun., 2009, 1076–1078 RSC
.
- H. Pang, Q. Y. Lu, J. J. Wang, Y. C. Li and F. Gao, Chem. Commun., 2010, 46, 2010–2012 RSC
.
- H. Pang, F. Gao and Q. Y. Lu, Chem. Commun., 2011, 47, 11772–11774 RSC
.
- R. Zhai, Y. Z. Wan, L. Liu, W. Q. Wang, J. D. Liu and B. Zhang, Water Sci. Technol., 2012, 65, 1054–1059 CAS
.
- Y. F. Zhao, B. Zhang, X. Zhang, J. H. Wang and J. D. Liu, Water Sci. Technol., 2010, 62, 937–946 CrossRef CAS
.
- A. Khaleel, P. N. Kapoor and K. J. Klabunde, Nanostruct. Mater., 1999, 11, 459–468 CrossRef CAS
.
- C. H. Weng, Y. T. Lin, C. L. Yeh and Y. C. Sharma, Water Sci. Technol., 2010, 62, 844–851 CrossRef CAS
.
- S. Vaucher, J. Fielden, M. Li, E. Dujardin and S. Mann, Nano Lett., 2002, 2, 225–229 CrossRef CAS
.
- P. H. Zhou, D. S. Xue, H. Q. Luo and X. G. Chen, Nano Lett., 2002, 2, 845–847 CrossRef CAS
.
- T. Uemura and S. Kitagawa, J. Am. Chem. Soc., 2003, 125, 7814–7815 CrossRef CAS
.
- N. L. K. Torad, M. Hu, M. Imura, M. Naito and Y. Yamauchi, Dalton Trans., 2012, 41, 10175 RSC
.
- M. H. Cao, X. L. Wu, X. Y. He and C. W. Hu, Chem. Commun., 2005, 2241–2243 RSC
.
- R. K. Motkuri, P. K. Thallapally, B. P. McGrail and S. B. Ghorishi, CrystEngComm, 2010, 12, 4003–4006 RSC
.
- M. Hu, J. S. Jiang, C. C. Lin and Y. Zeng, CrystEngComm, 2010, 12, 2679–2683 RSC
.
- H. L. Sun, H. T. Shi, F. Zhao, L. M. Qi and S. Gao, Chem. Commun., 2005, 4339–4341 RSC
.
- L. Hu, J. Y. Mei, Q. W. Chen, P. Zhang and N. Yan, Nanoscale, 2011, 3, 4270–4274 RSC
.
- L. Hu, P. Zhang, Q. W. Chen, J. Y. Mei and N. Yan, RSC Adv., 2011, 1, 1574–1578 RSC
.
- L. Hu, P. Zhang, Q. W. Chen, N. Yan and J. Y. Mei, Dalton Trans., 2011, 40, 5557–5562 RSC
.
- L. G. Beauvis and J. R. Long, Inorg. Chem., 2006, 45, 236–243 CrossRef
.
- S. Natesakhawat, J. T. Culp, C. Matranga and B. J. Bockrath, J. Phys. Chem. C, 2007, 111, 1055–1060 CAS
.
- P. K. Thallapally, R. K. Motkuri, C. A. Fernandez, B. P. McGrail and G. S. Behrooz, Inorg. Chem., 2010, 49, 4909–4915 CrossRef CAS
.
- S. Y. Mak and D. H. Chen, Dyes Pigm., 2004, 61, 93–98 CrossRef CAS
.
- L. Ai, Y. Zhou and J. Jiang, Desalination, 2011, 226, 72–77 CrossRef
.
Footnote |
† Electronic Supplementary Information (ESI) available. See DOI: 10.1039/c2ra20803b |
|
This journal is © The Royal Society of Chemistry 2012 |