DOI:
10.1039/C2RA20787G
(Paper)
RSC Adv., 2012,
2, 7803-7808
A multiporous electrochemical sensor for epinephrine recognition and detection based on molecularly imprinted polypyrrole†
Received
27th April 2012
, Accepted 21st June 2012
First published on 25th June 2012
Abstract
A novel multiporous imprinted electrochemical sensor was developed for the recognition and detection of epinephrine (EP) by combining a molecularly imprinted polymers (MIPs) film, silica nanoparticles (SiO2NPs) and multiwalled carbon nanotubes (MWNTs). A molecular imprinted polypyrrole film was electropolymerized on the surface of a SiO2NPs and MWNTs modified glassy carbon electrode (GCE) in the presence of EP. With the etching of SiO2NPs, the obtained sensor exhibited a multiporous network structure to allow for efficient mass transport, which would improve the rebinding rate and increase the efficiency of imprinted sites of the sensor. The multiporous imprinted sensor was characterized using scanning electron microscopy (SEM), electrochemical impedance spectroscopy (EIS), differential pulse voltammetry (DPV) and amperometric i–t measurement. The influential factors, including the electropolymerization cycles, the scanning rate, the amount of SiO2NPs, and the incubation time of SiO2NPs in HF solution were optimized. Under the optimized conditions, the sensor could recognize EP from other structurally similar compounds. A good linearity was obtained in the range of 3.0 × 10−7–1.0 × 10−3 M with a detection limit of 3.0 × 10−8 M. The MIPs based sensor showed high sensitivity, good selectivity and satisfactory reproducibility for EP determination.
1. Introduction
In recent years, various nanoscaled materials, such as nanoparticles, nanotubes and nanofibers, have been widely used in many fields.1–5 Due to their advantages of highly tunable size- and shape-dependent chemical and physical properties and high specific surface area, these nanomaterials have enjoyed widespread attention for the development of biosensors.6–8 Especially, multiwalled carbon nanotubes (MWNTs), as an attractive nanomaterial with good strength and flexibility, high thermal and electrical conductivity and low density, has been modified on different electrode surfaces to detect various biomolecules and inorganic ions.9–15 Among these analytes, epinephrine (EP) is an important catecholamine neurotransmitter in the mammalian central nervous system, which is about nmol L-1 level in human serum. Many life phenomena are related to EP level in the body fluid. Medically, EP has been used as a common emergency healthcare medicine. Also, low levels of EP have been found in patients with Parkinson's disease.16,17 Therefore, reliable, rapid and selective determination of trace levels of EP is extremely important for pharmaceutical preparation and health care. Many analytical techniques have been developed to meet this demand, such as high-performance liquid chromatography, fluorimetry and chemiluminescence. Loannou et al. have used terbium sensitized fluorescence as a post-column detection system to develop a sensitive high-performance liquid chromatographic method for the detection of EP.18 A chemiluminescence method was employed by Michalowski et al to detect EP in the two-channel manifold.19 In that work, EP could be determined in pharmaceutical preparations with a detection limit of 0.2 mg L−1. While these approaches are fully adequate in terms of their accuracy, they do require expensive instrumentation, highly skilled technicians, and complicated and time-consuming procedures. Electrochemical methods provide useful alternatives due to their simplicity, high sensitivity, good stability, low-cost instrumentation, and on-site monitoring. Many effects have been devoted to the development of new electrochemical sensors for monitoring EP. Edge-plane pyrolytic graphite electrode modified with multiwalled carbon nanotubes (MWNTs/EPPGE) has been used as a sensor for the efficient quantitative determination of epinephrine in the body fluid of smokers and nonsmokers in resting stage by using cyclic voltammetry and square-wave voltammetry.20 Gold electrodes modified with S-containing compounds and gold nanoparticles have been used for determination of norepinephrine in aqueous solution.21 The sensor exhibits excellent stability and reproducibility. Moraes et al. outlined the development and application of a composite electrode prepared by mixing paraffin, multiwalled carbon nanotubes (MWCNTs) and cobalt phthalocyanine (CoPc) for selective determination of epinephrine, and human urine samples without any purification step were successfully analyzed under the standard addition method using a paraffin/MWCNTs/CoPc composite electrode.22 However, for electrochemical sensors, a major problem for EP determination is the very low EP concentration and the large excesses of interfering substances, such as ascorbic acid (AA) and uric acid (UA), which often coexist with EP in physiological fluids and have oxidation potentials close to that of EP, resulting in the electrochemical response of EP being almost overlapped by those of AA and UA.23,24
Molecular imprinting is known as a well-established and facile technique to synthesize molecularly imprinted polymers (MIPs) with a large variety of recognition sites, which are complementary to the template molecule in size, shape and interaction.25–28 The attractive features of MIPs, such as high mechanical strength, the ease of preparation, low cost and high selectivity, allow MIPs to be used in various applications, especially in electrochemical sensors.29–31 Many MIPs-based electrochemical sensors have been reported in the literature for the recognition and detection of different template molecules.32–36 However, the diffusion of the template molecules to the imprinted sites, and the sensitivity of template molecules detection are still important problems of MIPs based electrochemical sensors. Conductive nanostructured materials might perform a role to improve the sensitivity of the sensors and MWNTs have also been employed to prepare MIPs-based electrochemical sensors. Yao et al. prepared an electrochemical sensor by combining MWNTs and MIPs for thymidine recognition and sensitive detection. MWNTs were introduced for the enhancement of electronic transmission and sensitivity.37 A screen-printed electrode modified with MWNTs and MIPs was reported by Chai et al.38 The electrochemical experimental results demonstrated that the sensor possessed a favorable sensitivity and selective recognition for ractopamine. An MIPs-based electrochemical sensor was investigated by Kubota et al.39 MWNTs modified by vinyltromethoxysilane were used to enhance the sensitivity of the sensor owing to its unique properties including huge surface area, strong adsorptive ability, subtle electronic properties and catalytic ability. An imprinted electrochemical sensor based on polypyrrole-sulfonated graphene/hyaluronic acid multiwalled carbon nanotubes for sensitive detection of tryptamine was prepared by Huang et al.40 In that work, carboxylated MWNTs were used to enhance the current response of the sensor due to its high aspect ratio, ultra-light weight, high mechanical strength, high electrical conductivity, high thermal conductivity and high surface-to-volume area. A successful strategy for the development of sensors with rapid response relies on the porosity of the polymers prepared on the electrode surface.
By taking the advantages of MIPs and nanostructured materials, a simple and sensitive electrochemical sensor was developed for EP recognition and detection. MWNTs were first electrodeposited on the surface of a glassy carbon electrode (GCE) to improve the sensitivity. After the introduction of silica nanoparticles (SiO2NPs) on the surface of the MWNTs modified electrode, pyrrole was electropolymerized in the presence of EP. With the etching of silica nanoparticles (SiO2NPs) and the extraction of EP from the polymers film, a multiporous MIPs based electrochemical sensor was obtained. The prepared sensor was characterized by scanning electron microscopy (SEM), electrochemical impedance spectroscopy (EIS), differential pulse voltammetry (DPV) and amperometric i–t measurement. Under the optimized conditions, the prepared sensor exhibited a good selectively recognition and a highly sensitive detection toward EP.
2. Experimental
2.1 Chemicals
Multiwalled carbon nanotubes (MWNTs) with a diameter of 10–30 nm and a length of 1–2 μm were obtained from Shenzhen Nanotech Port Co. Ltd., China. Dopamine (DA, ≥98.5%), ascorbic acid (AA, >98%), uric acid (AA, >99%), epinephrine hydrochloride (EP, >98%) and pyrrole were purchased from Fluka (Fluka Chemie AG, Switzerland). Tetraethoxysilane (TEOS), ammonia, sodium dihydrogen phosphate, sodium hydrogen phosphate, and all other reagents were of at least analytical reagent grade. Double-distilled deionized water was used for all solutions.
2.2 Apparatus
Electrochemical experiments such as cyclic voltammetry (CV), differential pulse voltammetry (DPV), electrochemical impedance spectroscopy (EIS) and amperometric i–t measurement were performed on a CHI 660C workstation (ChenHua Instruments Co., Shanghai, China) with a conventional three-electrode system. A bare or modified glassy carbon electrode (GCE) served as a working electrode, and a saturated calomel electrode and a platinum wire electrode were used as the reference and counter electrode, respectively. Field emission scanning electron microscope (FE-SEM) images were obtained on an S-4800 field emission scanning electron microanalyser (Hitachi, Japan).
2.3 Preparation of MWNTs-modified glassy carbon electrode (MWNTs/GCE)
Crude MWNTs (0.5 g) were added to 60 mL of concentrated HNO3 under sonication for 10 min. Next, the mixture was refluxed at 85 °C for approximately 4–5 h. After cooling to room temperature, the mixture was separated by vacuum filtration and washed thoroughly with distilled water several times until the pH value of the filtrate was neutral; this process led to MWNTs-COOH as the product. Prior to modification, a bare GCE was polished with a 0.3 μm alumina slurry on micro-cloth pads and sonicated sequentially in water. After sonication, an amperometry method was employed to deposit the MWNTs-COOH onto the bare GCE surface by applying a potential of +1.7 V for 400 s in a solution containing 0.3 mg mL−1 of MWNTs-COOH; this process gave MWNTs-COOH modified GCE (MWNTs/GCE).
2.4 Fabrication of multiporous MIPs-modified MWNTs/GCE (multiporous MIPs/MWNTs/GCE)
Silica nanoparticles (SiO2NPs) were prepared according to the literature with a slight modification.41 2.08 g tetraethoxysilane (TEOS) was added in 50 mL absolute ethanol for 5 min, followed by adding a mixture of 0.12 g H2O, 3.85 mL ammonia (25%), and 40 mL ethanol. The mixture was stirred at room temperature for 20 h. The obtained milky particles were collected by centrifugation and washed with ethanol for several times to remove the residual reagents. Then 5 mg SiO2NPs was dispersed in 10 mL ethanol with ultrasound for 20 min. Then 5 μL of the above suspension was dropped on the MWNTs/GCE surface and dried at room temperature to obtain SiO2NPs modified MWNTs/GCE (SiO2NPs/MWNTs/GCE).
The prepared SiO2NPs/MWNTs/GCE was immersed into a 0.1 M LiClO4 solution containing 0.029 M pyrrole and 0.02 M EP. Then cyclic voltammetry (CV) was performed from −0.80 to +1.00 V for five cycles at a scan rate of 80 mV s−1, obtaining polymer-modified SiO2NPs/MWNTs/GCE. In order to obtain a multiporous MIPs modified electrode, the above electrode was incubated into hydrofluoric acid (HF) solution to etch off SiO2NPs completely. After washing with water for several times, the electrode was immersed in 0.1 M KCl and 0.05 M phosphate buffer solution (PBS, pH 7.0) to extract embedded EP by scanning between −0.6 and +1.00 V for several cycles until no obvious oxidation peak of EP could be observed. This process gave a multiporous MIPs-modified MWNTs/GCE (multiporous MIPs/MWNTs/GCE). The procedure for the construction of multiporous MIPs/MWNTs/GCE is depicted in Scheme 1.
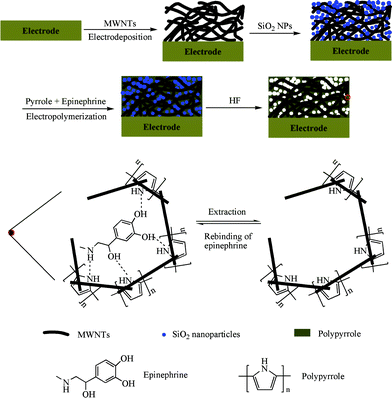 |
| Scheme 1 Schematic illustrations of the fabrication procedure for multiporous MIPs/MWNTs/GCE and the rebinding mechanism in the MIPs cavities. | |
As a control, a multiporous non-molecularly imprinted polymers (NIPs) modified MWNTs/GCE (multiporous NIPs/MWNTs/GCE) was also prepared and treated in exactly the same manner except for the omission of EP in the electropolymerization process.
In order to investigate the effect of SiO2NPs, two further modified electrodes were prepared by direct electropolymerization of pyrrole on the MWNTs/GCE surface in the presence and absence of EP, which were assigned as MIPs/MWNTs/GCE and NIPs/MWNTs/GCE, respectively.
2.5 Electrochemical properties measurements
Electrochemical measurements for the characterization of the prepared modified electrodes were performed by using different electrochemical techniques, including DPV, EIS and amperometric i–t measurement. AA, UA and DA were chosen as the interferences to evaluate the recognition capacity of the prepared sensor. The EIS was carried out in 0.01 M Fe(CN)63−/4− and 0.1 M KCl solution at the formal potential of 0.18 V with a frequency range of 1 × 105 ∼1 Hz and a signal amplitude of 5 mV. A method of standard addition was followed for the detection of DPV peak current of EP on different electrodes, which was recorded between −0.2 and +0.6 V at a scan rate of 100 mV s−1. Amperometric current response of multiporous MIPs/MWNTs/GCE or multiporous NIPs/MWNTs/GCE was recorded by the successive addition of EP with various concentrations under a gentle stirring in PBS.
3. Results and discussion
3.1 Characterization of modified electrodes
Electrochemical impedance spectroscopy (EIS) is a valuable approach to investigate the stepwise construction process of the sensor. Fig. 1 illustrates the Nyquist diagrams of the electrodes fabricated at each step in the presence of 0.01 M [Fe(CN)6]3−/[Fe(CN)6]4−. As shown in curve Fig. 1a, there was a very small semicircle domain present, as reported in literature, implying there was a very low electron transfer resistance to the redox-probe dissolved in the electrolyte solution.42 When the surface was coated with MWNTs, the slope of the line part increased dramatically (Fig. 1b), indicating that the MWNTs formed high electron conduction pathways between the electrode and electrolyte. Compared with the SiO2NPs modified GCE, which was only loaded with the same amount of SiO2NPs (Fig. 1d), the SiO2NPs/MWNTs/GCE (Fig. 1c) also showed an obvious low electron transfer resistance. Coupled with the introduction of the polymers, the prepared NIPs/SiO2NPs/MWNTs/GCE (Fig. 1e) or MIPs/SiO2NPs/MWNTs/GCE (Fig. 1f) displayed an obvious increase in the interfacial resistance, implying the formation of the hindered pathway for the electron transfer within the polymers. With the etching of SiO2NPs, a decrease of semicircle diameter of multiporous MIPs/MWNTs/GCE was observed, which could be attributed to the formation of multiporous structured MIPs by the removal of SiO2NPs (Fig. 1g). Furthermore, after immersing multiporous MIPs/MWNTs/GCE in 2.0 × 10−5 M EP, the resistance substantially increased (Fig. 1h), owing to the rebinding of EP into the imprinted cavities and the blocking of the arrival of [Fe(CN)6]3−/[Fe(CN)6]4− to the electrode surface.
![EIS recorded in 0.01 M [Fe(CN)6]3−/[Fe(CN)6]4− solution using bare GCE (a), MWNTs/GCE (b), SiO2NPs/MWNTs/GCE (c), SiO2NPs/GCE (d), NIPs/SiO2NPs/MWNTs/GCE (e) and MIPs/SiO2NPs/MWNTs/GCE (f) before the extraction of EP, and multiporous MIPs/MWNTs/GCE (g) and multiporous MIPs/MWNTs/GCE after incubating in 2.0 × 10−5 M EP solution (h).](/image/article/2012/RA/c2ra20787g/c2ra20787g-f1.gif) |
| Fig. 1 EIS recorded in 0.01 M [Fe(CN)6]3−/[Fe(CN)6]4− solution using bare GCE (a), MWNTs/GCE (b), SiO2NPs/MWNTs/GCE (c), SiO2NPs/GCE (d), NIPs/SiO2NPs/MWNTs/GCE (e) and MIPs/SiO2NPs/MWNTs/GCE (f) before the extraction of EP, and multiporous MIPs/MWNTs/GCE (g) and multiporous MIPs/MWNTs/GCE after incubating in 2.0 × 10−5 M EP solution (h). | |
SEM was employed to characterize the morphological structures of the sensor. It was obvious from the image that the MWNTs were distributed on the surface of the GCE and formed a three-dimensional network structure, which enabled the MWNTs/GCE to have a much higher specific surface area (Fig. 2A). The network structure of the MWNTs/GCE is attractive for electrochemical applications, especially for fabricating electrochemical sensors, because each of the tubes is accessible to analyte, and can be used as an electrochemical sensing unit, yielding a sensitive and efficient signal. After the modification of MWNTs/GCE with SiO2NPs, the surface image (Fig. 2B) showed clearly that there were many granules on the surface of MWNTs or in the gaps between MWNTs with an average size of about 30 nm (amplified SEM image of SiO2NPs shown in the inset of Fig. 2B). With the electropolymerization of the MIPs, a layer of film was coated on the surface of MWNTs and SiO2NPs (Fig. 2C), indicating the formation of the composite film of MIPs/SiO2NPs/MWNTs. All of the granules embedded in the above composite film disappeared after the MIPs/SiO2NPs/MWNTs/GCE was immersed in HF solution, as shown in Fig. 2D. As a result, some pores were left in the MIPs layer to provide high-speed pathways for the template molecules transfer from solution to the sensor.
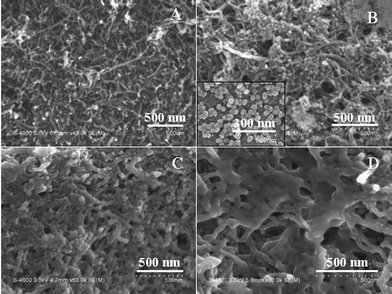 |
| Fig. 2 SEM images of MWNTs/GCE (A), SiO2NPs/MIPs/GCE (B), MIPs/SiO2NPs/MWNTs/GCE (C) and multiporous MIPs/MWNTs/GCE (D). The inset of (B) is the SEM image of SiO2NPs. | |
In order to investigate the effect of SiO2NPs, the electrochemical responses of EP on MIPs/MWNTs/GCE and multiporous MIPs/MWNTs/GCE were compared using DPV, as shown in Fig. 3. It is apparent that multiporous MIPs/MWNTs/GCE displayed much higher current response than that of the MIPs/MWNTs/GCE under the same concentrations of EP, which could be ascribed to the involvement of SiO2NPs in the preparation of the sensor. The pores produced by the etching of SiO2NPs could provide many pathways for the transfer of the template molecules from solution to the MIPs matrix and a higher surface area to make much more imprinted sites situate approximately at the surface of MIPs layer. Therefore, compared with the MIPs/MWNTs/GCE, multiporous MIPs/MWNTs/GCE exhibited a much higher sensitivity.
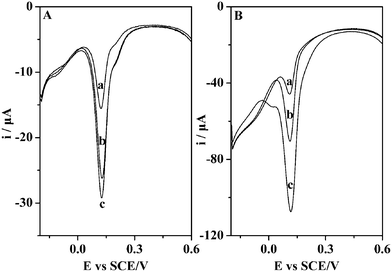 |
| Fig. 3 (A) DPV of MIPs/MWNTs/GCE incubated in 2.5 × 10−5 M (a), 5.0 × 10−5 M (b) and 7.3 × 10−5 M (c) EP solution. (B) DPV of multiporous MIPs/MWNTs/GCE incubated in 2.8 × 10−5 M (a), 5.0 × 10−5 M (b) and 7.5 × 10−5 M (c) EP solution. | |
3.2 The binding behaviors of sensor to EP
To construct an efficient sensor, different influencing factors including pH value of electropolymerization solution, electropolymerization cycles, scan rate, amount of SiO2NPs, and incubation time in HF solution were investigated. The results are summarized in Electronic Supporting Information (ESI†).
DPV measurements were also employed to elucidate the molecular specific adsorption properties of multiporous MIPs/MWNTs/GCE. Fig. 4 displays the DPV curves of EP (7.5 × 10−5 M) on multiporous MIPs/MWNTs/GCE as well as on multiporous NIPs/MWNTs/GCE. Compared with multiporous NIPs/MWNTs/GCE, a remarkable increase of current response was found for EP on multiporous MIPs/MWNTs/GCE. The high electrochemical response of the imprinted sensor might result from the imprinted cavities in the MIPs and the functional groups on the cavities produced by the template molecules. Therefore, the imprinted sensor showed an evident specific adsorption capacity to EP.
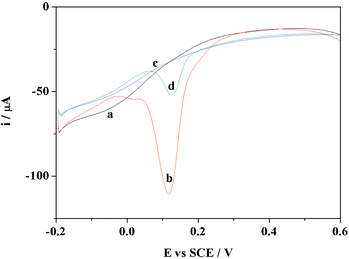 |
| Fig. 4 DPV of multiporous MIPs/MWNTs/GCE before (a) and after (b) incubating in 7.5 × 10−5 M EP solution, and multiporous NIPs/MWNTs/GCE before (c) and after (d) incubating in 7.5 × 10−5 M EP solution. | |
3.3 Detection of EP
In the amperometric detection, the concentrations of EP was set from 0 to 3.1 × 10−3 M. The i–t curves demonstrated that the current depended on the successive additions of EP, as shown in Fig. 5A. The imprinted sensor exhibited a much higher current response than that of the non-imprinted sensor. Fig. 5B shows the current response was proportional to the EP concentrations over the range of 3.0 × 10−7–1.0 × 10−3 M with a correlation coefficient of −0.998, and a limit of detection was measured of 3.0 × 10−8 M. All experiments were replicated three times and the precision was about 2.5%. The results revealed a lower limit of detection and wider linear response compared to a previously reported MIPs based electrochemical sensor for EP detection.43 The response of the imprinted sensor yielded a slope of −0.043 μA mM−1, and the corresponding value for the non-imprinted sensor was −0.011 μA mM−1. Therefore, the sensitivity of the imprinted sensor was about 4-fold of that of the non-imprinted sensor. The much greater slope associated with the imprinted sensor indicated that the significant current response was mainly attributed to the specific interactions between EP and the imprinted sites in the MIPs layer. Meanwhile, the above results also showed that EP could be quantitatively detected using the imprinted sensor by the measurement of current changes. In the case of multiporous MIPs/MWNTs/GCE, the imprinted sites were situated at or approximately at the surface of MIPs, and provided excellent site accessibility and a low mass-transfer resistance for EP. Therefore, multiporous MIPs/MWNTs/GCE exhibited very high sensitivity to the template molecule. Moreover, it is also obvious that the current on the sensor reached equilibrium within almost 30 s, indicating the fast rebinding rate for the template molecule. No linear response of the imprinted sensor to EP was observed when the EP concentration was higher than 1.0 × 10−3 M, probably because of saturated rebinding of imprinted sites to template molecules.
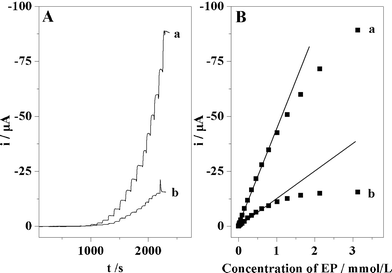 |
| Fig. 5 (A) i–t curves of EP on multiporous MIPs/MWNTs/GCE (a) and on multiporous NIPs/MWNTs/GCE (b), respectively. The concentration of EP for the curves are as follows: 3.0 × 10−7, 9.0 × 10−7, 2.9 × 10−6, 7.9 × 10−6, 1.6 × 10−5, 3.1 × 10−5, 5.6 × 10−5, 9.6 × 10−5, 1.6 × 10−4, 2.4 × 10−4, 3.4 × 10−4, 4.6 × 10−4, 6.1 × 10−4, 7.9 × 10−4, 1.0 × 10−3, 1.3 ×10−3, 1.6 × 10−3, 2.1 × 10−3, and 3.1 × 10−3 M. (B) Linear relationships between the peak current and the concentration of EP in the range of 3.0 × 10−7–1.0 × 10−3 M on multiporous MIPs/MWNTs/GCE. | |
3.4 Selectivity of multiporous MIPs/MWNTs/GCE
The selectivity of the prepared sensor was investigated using AA, UA and DA as structural analogues of EP. The concentration of the tested compounds was 2.5 ×10−4 M, which was the 25 times that of EP used in the selectivity measurements. Compared with multiporous NIPs/MWNTs/GCE, the current response caused by EP on multiporous MIPs/MWNTs/GCE was higher because of the presence of imprinted cavities, as shown in Fig. 6. The current of EP on multiporous NIPs/MWNTs/GCE showed no obvious increase in comparison to the other compounds, whereas the current of EP on multiporous MIPs/MWNTs/GCE was at least 5 or 6 times that of the other compounds.
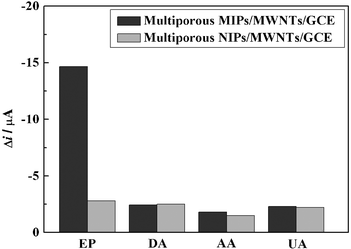 |
| Fig. 6 The selectivity of multiporous MIPs/MWNTs/GCE. | |
The ratio of the current (II/IN) of each compound obtained between multiporous MIPs/MWNTs/GCE and multiporous NIPs/MWNTs/GCE was also compared, where II and IN were the currents of each compound recorded on multiporous MIPs/MWNTs/GCE and multiporous NIPs/MWNTs/GCE by DPV, respectively. The calculated ratios of the current for EP, DA, AA and UA were 5.23, 0.97, 1.20 and 1.05, respectively. The results suggested that multiporous MIPs/MWNTs/GCE possessed an excellent selectively recognition capacity to EP. This is because the imprinting process created binding sites with shape and functional group complementary to the template molecule, resulting in the high selectivity of multiporous MIPs/MWNTs/GCE toward the template molecule. However, all of the other compounds had no complementary shape and functional group to match with the imprinting cavities, resulting in only slightly different current changes.
3.5 Reproducibility of multiporous MIPs/MWNTs/GCE
The reproducibility of multiporous MIPs/MWNTs/GCE was investigated using an EP concentration of 1.0 × 10−5 M. The peak current response of EP was determined using five different electrodes, which were prepared under the same conditions. The peak current response showed a relative standard deviation of 1.3%, confirming that the prepared sensor could be reproducibly fabricated. Furthermore, multiporous MIPs/MWNTs/GCE could be used more than 11 times after subsequent cycles of washing and measuring operations. The current response of the imprinted sensor decreased to 95% after storing for 1 week at 4 °C. The results demonstrated that the prepared electrochemical sensor had excellent regeneration property and stability. Alternatively, multiporous MIPs/MWNTs/GCE for other template molecules could also be easily prepared using the above described procedure.
Conclusions
In this study, a novel MIPs based electrochemical sensor obtained by cyclic voltammetric electropolymerization of a polypyrrole film on SiO2NPs/MWNTs/GCE surface in the presence of EP was successfully fabricated. The existence of MWNTs and the subsequent removal of SiO2NPs led to the formation of a multiporous network structured MIPs film. The prepared sensor displayed a good recognition capacity for the template molecule compared with other structural analogues. A linear relationship between the EP concentration and the current response was obtained of 3.0 × 10−7–1.0 × 10−3 M with a low detection limit of 3.0 ×10−8 M. The prepared low-cost electrochemical sensor could be possibly applied in measurements of EP in clinical samples and in the pharmaceutical industry.
Acknowledgements
We greatly appreciate the support of the National Natural Science Foundation of China through the Young Program (21005002), the Anhui Provincial Natural Science Foundation through the Young Program (11040606Q35), the Anhui University Provincial Natural Science Foundation Key program (KJ2010A138), and the Dr Start-up Foundation of the Anhui Normal University (160-750834).
References
- J. P. Cleuziou, W. Wernsdorfer, T. Ondarçuhu and M. Monthioux, ACS Nano, 2011, 5, 2348 CrossRef CAS.
- A. R. Hall, J. M. Keegstra, M. C. Duch, M. C. Hersam and C. Dekker, Nano Lett., 2011, 11, 2446 CrossRef CAS.
- J. Puigmartí-Luis, M. Rubio-Martínez, U. Hartfelder, I. Imaz, D. Maspoch and P. S. Dittrich, J. Am. Chem. Soc., 2011, 133, 4216 CrossRef.
- L. N. Protasova, E. V. Rebrov, K. L. Choy, S. Y. Pung, V. Engels, M. Cabaj, A. E. H. Wheatley and J. C. Schouten, Catal. Sci. Technol., 2011, 1, 768 Search PubMed.
- K. Yu, Z. Bo, G. Lu, S. Mao, S. Cui, Y. Zhu, X. Chen, R. S. Ruoff and J. Chen, Nanoscale Res. Lett., 2011, 6, 202 CrossRef.
- B. Pérez-López and A. Merkoci, Trends Food Sci. Technol., 2011, 22, 625–639 CrossRef.
- H. Qi, Y. Peng, Q. Gao and C. Zhang, Sensors, 2009, 9, 674 CrossRef CAS.
- H. Li, S. Liu, Z. Dai, J. Bao and X. Yang, Sensors, 2009, 9, 8547 CrossRef CAS.
- Y. C. Tsai, S. C. Li and S. W. Liao, Biosens. Bioelectron., 2006, 22, 495 CrossRef CAS.
- M. A. Rahman, P. Kumar, D. S. Park and Y. B. Shim, Sensors, 2008, 8, 118 CrossRef CAS.
- M. Pumera, Chem.–Eur. J., 2009, 15, 4970 CrossRef CAS.
- H. Beitollahi, H. Karimi-Maleh and H. Khabazzadeh, Anal. Chem., 2008, 80, 9848 CrossRef CAS.
- A. Benvidi, P. Kakoolaki, H. R. Zare and R. Vafazadeh, Electrochim. Acta, 2011, 56, 2045 CrossRef CAS.
- R. T. Kachoosangi, M. M. Musameh, I. Abu-Yousef, J. M. Y. S. M. Kanan, L. Xiao, S. G. Davies, A. Russell and R. G. Compton, Anal. Chem., 2009, 81, 435 CrossRef CAS.
- Q. Yang, Q. Sun, T. Zhou, G. Shi and L. Jin, J. Agric. Food Chem., 2009, 57, 6558 CrossRef CAS.
- S. Dimitrov, S. Benedict, D. Heutling, J. Westermann, J. Born and T. Lange, Blood, 2009, 113, 5134–5143 CrossRef CAS.
- S. F. Kemp, R. F. Lockey and F. E. R. Simons, Allergy, 2008, 63, 1061–1070 CrossRef CAS.
- M.A. Fotopoulou and P.C. Loannou, Anal. Chim. Acta, 2002, 462, 179 CrossRef CAS.
- J. Michalowski and P. Halaburda, Talanta, 2001, 55, 1165 CrossRef CAS.
- R.N. Goyal and S. Bishnoi, Electrochim. Acta, 2011, 56, 2717 CrossRef CAS.
- T. Łuczak, Electroanalysis, 2010, 22, 2641 CrossRef.
- F. C. Moraes, D. L. C. Golinelli, L. H. Mascaro and S. A. S. Machado, Sens. Actuators, B, 2010, 148, 492 CrossRef.
- S. Shahrokhiana, M. Ghalkhani and M. K. Amini, Sens. Actuators, B, 2009, 137, 669 CrossRef.
- P. Kalimuthu and S. A. John, Anal. Chim. Acta, 2009, 647, 97 CrossRef CAS.
- G. Wulff, Angew. Chem., Int. Ed. Engl., 1995, 34, 1812 CrossRef CAS.
- G. Vlatakis, L. I. Andersson and K. Mosbach, Nature, 1993, 361, 645 CrossRef CAS.
- M. Riskin, R. Tel-Vered, T. Bourenko, E. Granot and I. Willner, J. Am. Chem. Soc., 2008, 130, 9726 CrossRef CAS.
- Y. Xie, D. Chen, J. Zhao, Y. Peng, N. Jiang, X. Zhou, S. Du and Z. Zhang, RSC Adv., 2012, 2, 273 RSC.
- T. Alizadeh, M. Zare, M. R. Ganjali, P. Norouzi and B. Tavana, Biosens. Bioelectron., 2010, 25, 1166 CrossRef CAS.
- S. Yang, J. Li, D. Shao, J. Hu and X. Wang, J. Hazard. Mater., 2009, 166, 109 CrossRef CAS.
- A. Aghaei, M. R. M. Hosseini and M. Najafi, Electrochim. Acta, 2010, 55, 1503 CrossRef CAS.
- X. Kan, T. Liu, H. Zhou, C. Li and B. Fang, Microchim. Acta, 2010, 171, 423 CrossRef CAS.
- S. P. Ozkorucuklu, Y. Sahin and G. Alsancak, Sensors, 2008, 8, 8463 CrossRef CAS.
- C. Xie, S. Gao, Q. Guo and K. Xu, Microchim. Acta, 2010, 169, 145 CrossRef CAS.
- M. C. Blanco-López, M. J. Lobo-Castañón, A. J. Miranda-Ordieres and P. Tuñón-Blanco, Biosens. Bioelectron., 2003, 18, 353 CrossRef.
- T. Jing, Y. Wang, Q. Dai, H. Xia, J. Niu, Q. Hao, S. Mei and Y. Zhou, Biosens. Bioelectron., 2010, 25, 2218 CrossRef CAS.
- Z. Zhang, Y. Hu, Z. Zhang, L. Luo and S. Zhuo, J. Electroanal. Chem., 2010, 644, 7 CrossRef CAS.
- H. Zhang, G. Liu and C. Chai, Sens. Actuators, B, 2012, 168, 103 CrossRef CAS.
- W. J. R. Santos, M. Santhiage, I. V. P. Yoshida and L. T. Kubota, Sens. Actuators, B, 2012, 166–167, 739 CrossRef CAS.
- X. Xing, S. Liu, J. Yu, W. Lian and J. Huang, Biosens. Bioelectron., 2012, 31, 277 CrossRef CAS.
- W. Stöber and A. Fink, J. Colloid Interface Sci., 1968, 26, 62 CrossRef.
- W. Song, Y. Chen, J. Xu, X. R. Yang and D. B. Tian, J. Solid State Electrochem., 2010, 14, 1909 CrossRef CAS.
- C. W. Hsu and M. C. Yang, Sens. Actuators, B, 2008, 134, 680 CrossRef.
|
This journal is © The Royal Society of Chemistry 2012 |